DOI:
10.1039/D4RA00036F
(Paper)
RSC Adv., 2024,
14, 3519-3524
Novel pyrazoline and pyrazole “turn on” fluorescent sensors selective for Zn2+/Cd2+ at λem 480 nm and Fe3+/Fe2+ at λem 465 nm in MeCN†
Received
2nd January 2024
, Accepted 17th January 2024
First published on 22nd January 2024
Abstract
A small series of simple pyrazoline and pyrazole based sensors, all derived from the same chalcone precursors, were synthesised, characterised and screened for their fluorescence “turn on” properties in the presence of multiple metals. Pyrazole 8 displayed an excellent fluorescence profile with approx. 20× fold increase in λem 480 nm with Zn2+ compared to a 2.5× fold increase with Cd2+. Pyrazole 9 displayed a 30× fold increase at λem 465 nm for Fe3+ compared to Fe2+ with a Fe3+ limit of detection of 0.025 μM. The corresponding pyrazolines displayed contrasting properties with important implications for future pyrazoline and pyrazole sensor design.
1 Introduction
Zinc is the second most abundant transition metal in the human body1 critical to a diverse range of biological functions including enzyme maintenance,2 gene expression3 and neurological functions.4 Unregulated zinc is implicated in a number of biological illnesses ranging from Alzheimer's disease,5 epilepsy6 and Parkinson's disease.7 Cadmium, also a group 12 transition metal sitting beneath zinc in the periodic table, is a highly toxic environmental and industrial pollutant with long term exposure linked to renal, breast and lung cancers.8,9 An ideal analytical technique to track and trace these two metals in biological systems is fluorescence spectroscopy due to its high specificity, low limit of detection and ability to fine-tune the emission wavelength.10–12 Fluorescence sensing typically involves two common approaches, either a “turn on” sensor13,14 in which the presence of the analyte of interest increases fluorescence emission intensity (λem) or a “turn off” sensor15,16 in which the analyte decreases fluorescence intensity. A major challenge remains which is the ability to selectively detect zinc over cadmium and vice versa in complex mixtures, with only a few examples having been reported in the literature.17 Multiple heterocyclic scaffolds are used to tune metal ion selection, photophysical and chemical properties to meet a particular sensor requirement. Pyrazoline,18 a 5 membered heterocyclic ring, and the closely related pyrazole19 are leading examples combining the advantageous properties of modular design from commercially available starting materials and the ability to Taylor the orientation of the three branching units off the main pyrazoline or pyrazole core (shown in blue and red respectively in Fig. 1). Pyrazolines chelators for gold,20 tin21 and ruthenium22 have all been reported with the groups of Miao and Zhao pioneering both “turn on” and “turn off” pyrazoline fluorescent sensors for multiple systems including Zn2+ in aqueous environments and living systems.23 A variety of photophysical processes result in increased fluorescence emission18,19 with the blocking of the photoinduced electron transfer (PET) process upon Zn2+ chelation a common pathway.13,17a,23a,35 Mixed fluorescent sensors composed of both pyrazoline and pyrazole units have also been reported24 highlighting that structural complexity is not a prerequisite for complex functionality, simple molecular structures provide the tools to detect biologically important analytes in living systems.
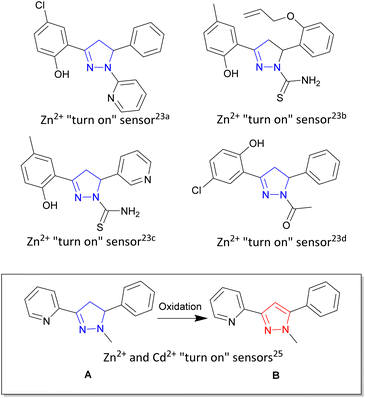 |
| Fig. 1 Pyrazoline heterocycle (shown in blue) in fluorescent “turn on” and “turn off” sensors developed by Miao and Zhao groups.23 Inset demonstrates “turn on” fluorescent sensor pyrazoline A can be converted into pyrazole B with different photophysical properties.25 | |
Previous work25 reported simple pyridine pyrazoline A as a “turn on” fluorescent sensor for both Zn2+ and Cd2+ producing a 1.7× fold higher response for Cd2+/Zn2+ (Fig. 1 inset). Oxidation of A to the corresponding pyrazole B produced a “turn on” sensor capable of distinguishing Zn2+/Cd2+ with λem 380 nm and λem 350 nm respectively. Herein we report the next generation of sensors with three novel pyrazolines 4–6 and their related three novel pyrazoles 7–9 with pyrazole 8 able to detect Zn2+ with a 20× fold increase in fluorescence at λem 480 nm compared to absence of Zn2+. Cd2+ resulted in only a 2.5× fold increase in fluorescence at λem 480 nm. In contrast, pyrazole 9 displayed only a minor increase in fluorescence with Zn2+ and Cd2+ but 30× fold increase in λem 465 nm with Fe3+ but surprisingly not Fe2+. These results within provide valuable insight for the design of the next generation of pyrazoline and pyrazole fluorescent sensors specific for Zn2+ and Fe3+.
2 Results and discussion
2,6-Diacetylpyridine underwent Claisen–Schmidt condensation26 with the required substituted aromatic aldehyde to afford the chalcone precursors 1–3 in acceptable yield (34–73%). After extensive investigation, it was discovered that a 2
:
1 ratio of ketone to aldehyde with catalytic amount of NaOH promoted formation of the required mono-chalcone and minimal formation of the bis-chalcone side product (ESI S3†). Bis-chalcone could be easily removed by gravity filtration allowing the desired chalcone to be further purified by recrystallisation preventing the requirement for time consuming column chromatography. Conversion of chalcone into pyrazoline 4–6 was achieved (ESI S2†) by adapting previous methods25,27 involving the 1,2 addition of methylhydrazine in slight excessive at room temperature (36–43% yield). Hydrazone formation was possible however following an extensive aqueous wash only the desired product was observed, this is comparable with other reports of hydrazone hydrolysis under similar conditions in the literature (Scheme 1).31
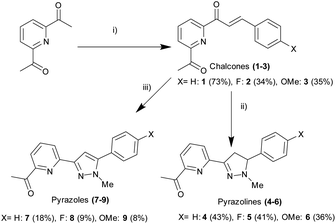 |
| Scheme 1 Synthesis of pyrazolines 4–6 and pyrazoles 7–9 from chalcone precursors 1–3. (i) 0.5 eq. of substituted aldehyde, 0.5 eq. NaOH, MeOH, rt, 18 h (ii) 1.2 eq. H2NNHMe, MeOH, rt, 3 h (iii) 8 eq. H2NNHMe, MeOH, rt, 24 h. Chemical yields are displayed in brackets. | |
This method was modified to yield the closely related pyrazole series 7–9 using excess methylhydrazine and longer reaction time to afford the pyrazole series in satisfactory yield (8–18%). Oxidation of pyrazoline to pyrazole has been reported previously25,28 with only a few literature examples of direct transformation from chalcone to pyrazole29,30 typically involving a catalyst and/or heating. One pot pyrazole synthesis from chalcone using excess methylhydrazine was confirmed by 1H NMR spectroscopy with formation of an aromatic pyrazole 1H singlet signal at approx. 7.1 ppm (Hd in Fig. 4 for 7, ESI S3† for 6 and 9) with the absence of the three sets of doublet of doublet signals characteristic of a pyrazoline ring reported previously.25,27 High resolution mass spectrometry confirmed pyrazole formation. The photophysical properties of these six novel compounds was explored using well-established protocols in the literature.12–20,23,25
Zn2+ chelation for the pyrazoline series was confirmed using UV/Vis spectroscopy in MeCN, this solvent was selected to enable direct comparison with previous studies25 and to determine the optimum photophysical properties for a future water-soluble sensor. The initial absorbance band at 320 nm (ε = 16
550 M−1 cm−1) decreasing with the linear appearance of a new band at 340 nm up to 2.0 equivalents (eq.) Zn2+ (Fig. 2 for pyrazoline 5 and ESI S4† for 4 and 6). All three pyrazolines exhibited similar absorbance profiles suggesting substitution at the aryl ring was not detrimental to chelation.
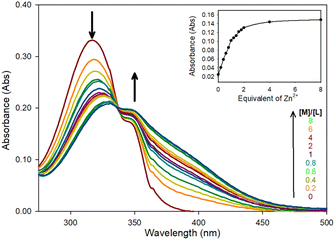 |
| Fig. 2 Absorbance spectra of 5 (20 μM) with incremental Zn2+ in MeCN. | |
A 1
:
2 ratio of sensor to Zn2+ was suggested from the linear increase in absorbance at 375 nm observed up to 2 eq. of Zn2+ (Fig. 2 inset) with further increases producing no significant increase in absorbance. Job plot analysis32 (Fig. 3 for 5 ESI S5† for 4–9) also indicated a 1
:
2 ratio between all pyrazolines and Zn2+. This is in contrast to pyrazoline A which had a 1
:
1 ratio of sensor to Zn2+. The addition of the acetyl group may be responsible for this additional Zn2+ chelation.
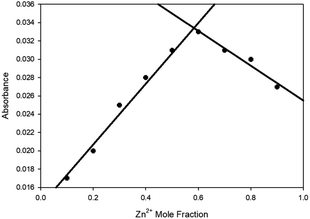 |
| Fig. 3 Job plot32 analysis of 5 with varying Zn2+ mole fractions in MeCN with absorbance at 400 nm, [Zn2+] + [5] = 100 μM. | |
Pyrazole 8 was selected for a Zn2+ 1H NMR spectroscopy titration experiment to confirm Zn2+ chelation (Fig. 4). Addition of 2 eq. Zn2+ resulted in significant shifts for Ha and Hc doublet of doublet peaks on the pyridine ring at 7.88 ppm and 8.17 ppm downfield to 8.26 ppm and 8.37 ppm. The Hb triplet pyridine proton peak and Hd singlet pyrazole peak increased to higher chemical shift from 7.95 ppm and 7.08 ppm to 8.45 ppm and 7.17 ppm consistent with Zn2+ chelation and previous literature examples.14,17,25,31 A similar response was observed with pyrazole 7 and pyrazoline compounds 4–6 (see ESI S2†) suggesting the pyridine ring chelates Zn2+ regardless if connected to a pyrazoline or pyrazole heterocycle.
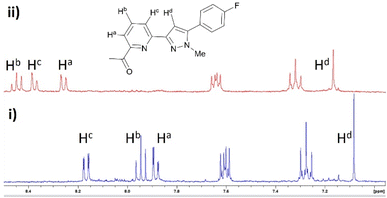 |
| Fig. 4 Partial 1H NMR spectra of (i) pyrazole 8 (MeCN-d3, 2 μM) and (ii) with addition of 2 eq. Zn2+. | |
Fluorescence spectroscopy was used to screen multiple metals to determine useful fluorescence properties. Pyrazoline 4 remains more sensitive to Cd2+ than Zn2+ with a 7.3× fold increase at λem 480 nm compared to 4.5× fold with Zn2+ (Fig. 5). It is interesting to note the similarity with the previous reported sensor A25 lacking the acetyl group also displayed higher fluorescence with Cd2+ over Zn2+ with a ratio of 1.7× fold higher emission at the same λem 465 nm with Cd2+ over Zn2+, comparable to the 1.6× fold reported above. A Zn2+ limit of detection (LoD)33 of 0.0319 μM for 4 and 0.010 μM for 5 was calculated (see ESI S7†) which is similar to the 0.0202 μM Zn2+ LoD for A25 other Zn2+ based “turn on” fluorescent sensors.23b This suggests the additional acetyl group is not conveying any beneficial effect in terms of Zn2+/Cd2+ analyte selectivity but provides a slight improvement in fluorescence response. This should be factored into future pyrazoline based sensor design. It is noteworthy to highlight further chemical modification to introduce additional functional groups via the acetyl group may enhance “turn on” fluorescence properties and this is ongoing research in our laboratory.
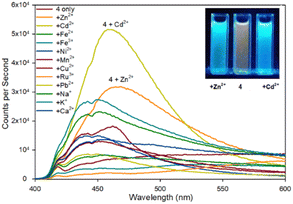 |
| Fig. 5 Fluorescence spectra of pyrazoline 4 upon addition of 5 eq. of the indicated metal. Inset from left to right, +Zn2+, 4 only, +Cd2+, λex365 nm 10 W lamp, 100 μM 4 with 500 μM metal cation. | |
Previous studies demonstrated pyrazolines can be converted into the closely related pyrazoles displaying “turn on” fluorescence properties in the presence of different cations. To investigate further, pyrazole series 7–9 were screened across multiple metals and to our surprise pyrazole 8 demonstrated an excellent “turn on” fluorescence response for Zn2+/Cd2+ (Fig. 6 for 8, ESI S6† for 7).
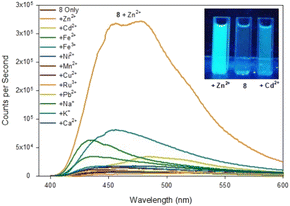 |
| Fig. 6 Fluorescence spectra of pyrazole 8 upon addition of 5 eq. of the indicated metal. Inset from left to right, +Zn2+, 8 only, +Cd2+, λex365 nm 100 W lamp, 100 μM 8 with 500 μM metal cation. | |
Upon addition of 5 eq. Zn2+ the fluorescence at λem 480 nm increased 20× fold whereas the addition of 5 eq. Cd2+ only resulted in a 2.5× fold increase at λem 480 mn resulting in approximately 8× fold increase in selectivity for Zn2+ over Cd2+. This is in contrast to the previously reported pyrazole B25 lacking the acetyl group which displayed a 13× fold increase λem 380 nm upon addition of 5 eq. Zn2+. Job plot analysis (ESI S5†) suggests a 1
:
1 ratio between 8 and Zn2+ similar to pyrazole B reported previously.25 Pyrazole 7 did not display a significant increase in fluorescence on addition of Zn2+ or Cd2+ (see ESI S6†) suggesting the electronegative fluorine group on the aryl ring was a key requirement of this “turn on” fluorescent response. Substitution of the 4-F for an electron donating 4-OMe group resulted in 9 which abolished this Zn2+ response. Further investigation revealed 9 displayed a 30× fold increase at λem 465 mn with Fe3+ but not Fe2+ (Fig. 7).
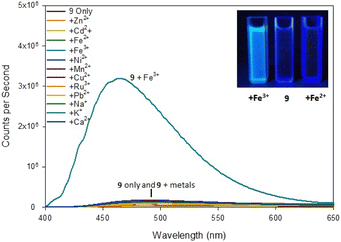 |
| Fig. 7 Fluorescence spectra of pyrazoline 9 on addition of 5 eq. of the indicated metal. Inset from left to right, +Fe3+, 9 only, +Fe2+ with λex 254 nm 6 W lamp. 100 μM 9 with 500 μM metal cations. | |
The difference was profound and could be visibly observed using a low power 6 W λex 254 nm TLC lamp (Fig. 7 inset). Job plot analysis (ESI S5†) suggested a 1
:
2 sensor to Fe3+ ratio. Pyrazole 9 is an excellent candidate for a “turn on” fluorescent sensor with a calculated Fe3+ LoD of 0.025 μM. This is noteworthy as the first reported Fe3+ specific pyrazoline sensor had a Fe3+ LoD of 3 μM (ref. 34) with recent pyrazole based Fe3+ sensors reporting Fe3+ LoD ranging from 0.021 μM (ref. 35) to 0.0004 μM.24 The proposes 1
:
1 binding mechanism of pyrazole 8 with Zn2+ and a 1
:
2 binding mechanism of pyrazole 9 with Fe3+ is displayed in Fig. 8. This agrees with previously reported pyrazoles with Zn2+ and Fe3+ in the literature.19,36–38
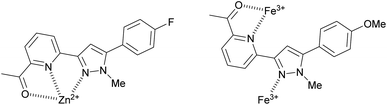 |
| Fig. 8 Proposed binding mechanisms for pyrazole 8 with Zn2+ and 9 with Fe3+. | |
Competition assays were performed to assess pyrazole 8 Zn2+ “turn on” fluorescence response in the presence of competing metal cations (Fig. 9). Fluorescence quenching was observed upon addition of a range of paramagnetic metals including Fe3+, Ni2+ and Co2+, a common phenomenon observed in the literature.25 A good fluorescence response in the presence of Na+ and K+ cations was observed suggesting the presence of these singularly charged metals was not detrimental to the Zn2+ sensing properties of 8.
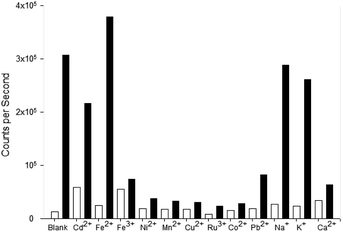 |
| Fig. 9 Competition experiments for pyrazole 8. The white bar represents 8 (MeCN, 20 μM, λex 290 nm, λem = 480 nm) with 5 eq. of the indicated cation; the black bars is the same plus 5 eq. Zn2+ after equilibrating for 3 min. | |
Pyrazole 9 was also submitted to a competition assay to evaluate its ability as a Fe3+ “turn on” sensor and it displayed a slightly better profile than 8 retaining modest fluorescence response in the presence of Fe2+, Mn2+, Cu2+, Ru3+ and Co2+ (Fig. 10). Unfortunately, the presence of Na+ and K+ did significantly reduce λem 455 nm fluorescence in contrast to 8.
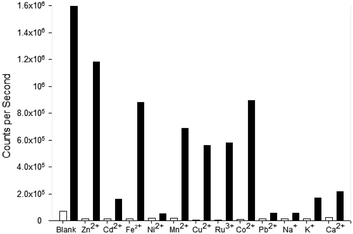 |
| Fig. 10 Competition experiments for pyrazole 9. The white bar represents 9 (MeCN, 20 μM, λex 290 nm, λem = 455 nm) with 5 eq. of the indicated cation; the black bars is the same plus 5 eq. Fe3+ after equilibrating for 3 min. | |
The competition assay profiles for 8 and 9 are similar to previously reported pyrazolines with paramagnetic metals typically hampering fluorescent response.25 Further work is required to enhance analyte chelation and prevent competing cations from disrupting the “turn on” response. The unexpected switch from a Zn2+ to a Fe3+ “turn on” sensor for 8 and 9 highlight the modular nature of the pyrazole heterocycle and how small modifications can have a profound influence on photophysical properties.
3 Conclusions
The addition of an acetyl group on the pyridine of pyrazole sensor 8 improved fluorescence properties (20× fold increase at λem 480 nm) for the detection of Zn2+ over Cd2+(2.5× fold increase also at λem 480 nm) compared to sensor B25 reported previously. Substitution of the electronegative 4-F on pyrazole 8 for an electron donating 4-OMe group in 9 resulted in the unexpected discovery of a “turn on” fluorescent sensor for Fe3+ at λem 465 nm. 9 displayed a Fe3+ LoD of 0.025 μM which is comparable to recently reported Fe3+ fluorescent sensors.24,35 These results suggest the acetyl group is highly beneficial and should be factored into future pyrazole sensor design. In contrast the same modification to pyrazoline sensors conferred no significant advantage in selectivity towards Zn2+/Cd2+ compared to sensor A25 however it did produce a slight increase in Zn2+ LoD. The above studies were performed in MeCN to aid comparison to previous work which was also carried out in MeCN. Previous exploratory reports for Zn2+ fluorescent sensors were performed in pure organic solvents also including MeOH,39,40 THF,41,42 DMF43 and DMSO.44,45 The results within provide a firm foundation for developing aqueous based sensors for lead pyrazole 8 with Zn2+ and 9 with Fe3+, this is ongoing work and will be reported in due course.
Author contributions
Alexander Ciupa designed, synthesised and characterised all compounds, performed all UV/Vis, NMR and fluorescence spectroscopy experiments and authored the manuscript.
Conflicts of interest
There are no conflicts to declare.
Acknowledgements
The author acknowledges Steven Robinson for assistance with qToF high resolution mass spectrometry, Glyn Connolly for NMR spectroscopy guidance and Krzysztof Pawlak with fluorescence spectroscopy. This work made use of shared equipment located at the Materials Innovation Factory; created as part of the UK Research Partnership Innovation Fund (Research England) and co-funded by the Sir Henry Royce Institute.
References
- C. T. Chasapis, C. A. Spiliopoulou, A. C. Loutsidou and M. E. Stefanidou, Arch. Toxicol., 2012, 86, 521 CrossRef CAS PubMed.
- C. Andreini and I. Bertini, J. Inorg. Biochem., 2012, 111, 150 CrossRef CAS PubMed.
- T. V. O'Halloran, Science, 1993, 261, 715 CrossRef PubMed.
- C. J. Frederickson, BioMetals, 2001, 14, 353 CrossRef CAS PubMed.
- M. A. Lovell, J. D. Robertson, W. J. Teesdale, J. L. Campbell and W. R. J. Markesbery, J. Neurol. Sci., 1998, 158, 47 CrossRef CAS PubMed.
- U. Doboszewska, K. Młyniec, A. Wlaz, E. Poleszak, G. Nowak and P. Wlaz, Pharmacol. Ther., 2019, 193, 156 CrossRef CAS PubMed.
- J. Sikora and A. M. Ouagazzal, Int. J. Mol. Sci., 2021, 22, 4724 CrossRef CAS PubMed.
- L. Järup, Br. Med. Bull., 2003, 68, 167 CrossRef PubMed; V. A. Florez-Garcia, E. C. Guevara-Romero, M. M. Hawkins, L. E. Bautista, T. E. Jenson, J. Yu and A. E. Kalkbrenner, Environ Res., 2022, 219, 115109 CrossRef PubMed.
- G. Genchi, S. M. Sinicropi, G. Lauria, A. Carocci and A. Catalano, Int. J. Environ. Res. Public Health, 2020, 17, 3782 CrossRef CAS PubMed.
- H. N. Kim, W. X. Ren, J. S. Kim and J. Yoon, Chem. Soc. Rev., 2012, 41, 1130 RSC.
- Y. Chen, Y. Bai, Z. Han, W. He and Z. Guo, Chem. Soc. Rev., 2015, 44, 4517 RSC.
- L. Xu, Y. Xu, W. Yhu, C. Yang, L. Han and X. Qian, Dalton Trans., 2012, 41, 7212 RSC.
- G. Wu, M. Li, J. Zhu, K. W. Chiu Lai, Q. Tong and F. Lu, RSC Adv., 2016, 6, 100696 RSC.
- A. Dhara, N. Guchhait, I. Mukherjee, A. Mukherjee and S. C. Bhattacharya, RSC Adv., 2016, 6, 105930 RSC.
- S. Manickam and S. K. Lyer, RSC Adv., 2020, 10, 11791 RSC.
- Y. Liu, S.-Q. Wang and B.-X. Zhao, RSC Adv., 2015, 5, 32962 RSC.
- Selected examples;
(a) P. Li, X. Zhou, R. Huang, L. Yang, X. Tang, W. Dou, Q. Zhao and W. Liu, Dalton Trans., 2014, 43, 706 RSC;
(b) H.-Y. Gong, Q.-Y. Zheng, X.-H. Zhang, D.-X. Wang and M.-X. Wang, Org. Lett., 2006, 8, 4895 CrossRef CAS PubMed;
(c) Z. Li, L. Zhang, L. Wang, Y. Guo, L. Cai, M. Yu and L. Wei, Chem. Commun., 2011, 47, 5798 RSC.
- B. Varghese, S. N. Al-Busafi, F. O. Suliman and S. M. Z. Al-Kindy, RSC Adv., 2017, 7, 46999 RSC.
- A. Tigreros and J. Portilla, RSC Adv., 2020, 10, 19693 RSC.
- S. Wang, W. Shao, H. Li, C. Liu, K. Wang and J. Zhang, Eur. J. Med. Chem., 2011, 46, 1914 CrossRef CAS PubMed.
- G. F. De Sousa, E. Garcia, C. C. Gatto, I. S. Resck, V. M. Deflon and J. D. Ardisson, J. Mol. Struct., 2010, 981, 46 CrossRef CAS.
- D. Havrylyuk, D. K. Heidary, Y. Sun, S. Parkin and E. C. Glazer, ACS Omega, 2020, 5, 18894 CrossRef CAS PubMed.
- Selected examples
(a) Z. Zhang, F.-W. Wang, S.-Q. Wang, F. Ge, B.-X. Zhao and J.-Y. Miao, Org. Biomol. Chem., 2012, 10, 8640 RSC;
(b) Z. L. Gong, F. Ge and B.-X. Zhao, Sens. Actuators, B, 2011, 159, 148 CrossRef CAS;
(c) M. M. Li, F. Wu, X. Y. Wang, T. T. Zhang, Y. Wu, Y. Xiao, J. Y. Miao and B.-X. Zhao, Anal. Chim. Acta, 2014, 826, 77 CrossRef CAS PubMed;
(d) T.-T. Zhang, F.-W. Wang, M.-M. Li, J. T. Liu, J. Y. Miao and B.-X. Zhao, Sens. Actuators, B, 2013, 186, 755 CrossRef CAS;
(e) T.-T. Zhang, X.-P. Chen, J.-T. Liu, L.-Z. Zhang, J.-M. Chu, L. Su and B.-X. Zhao, RSC Adv., 2014, 4, 16973 RSC.
- Y. P. Zhang, X. F. Li, Y. S. Yang, J. L. Wang, Y. C. Zhao and J. J. Xue, J. Fluoresc., 2021, 31, 29 CrossRef CAS PubMed.
- A. Ciupa, M. F. Mahon, P. A. De Bank and L. Caggiano, Org. Biomol. Chem., 2012, 10, 8753 RSC.
- See selected examples;
(a) L. Claisen and A. Claparède, Ber. Dtsch. Chem. Ges., 1881, 14, 2460 CrossRef;
(b) J. G. Schmidt, Ber. Dtsch. Chem. Ges., 1881, 14, 1459 CrossRef;
(c) D. Coutinho, H. G. Machado, V. H. Carvalho-Silva and W. A. da Silva, Phys. Chem. Chem. Phys., 2021, 23, 6738 RSC;
(d) C. L. Perrin and J. Woo, Phys. Chem. Chem. Phys., 2022, 24, 18978 RSC.
- A. Ciupa, P. A. De Bank, M. F. Mahon, P. J. Wood and L. Caggiano, MedChemComm, 2013, 4, 956 RSC.
- S. Hofmann, M. Linden, J. Neuner, F. N. Weber and S. R. Waldvogel, Org. Biomol. Chem., 2023, 21, 4694 RSC.
- Y. Ding, T. Zhang, Q.-Y. Chen and C. Zhu, Org. Lett., 2016, 18, 4206 CrossRef CAS PubMed.
- S. M. Landge, A. Schmidt, V. Outerbridge and B. Török, Synlett, 2007, 10, 1600 Search PubMed.
- R. Nguyen and I. Huc, Chem. Commun., 2003, 8, 942 RSC.
- P. Job, Ann. Chim., 1928, 9, 113 CAS.
- B. P. Joshi, J. Park, W. I. Lee and K.-H. Lee, Talanta, 2009, 78, 903 CrossRef CAS PubMed.
- S. Hu, S. Zhang, C. Gao, C. Xu and Q. Gao, Spectrochim. Acta, Part A, 2013, 113, 325 CrossRef CAS PubMed.
- K. Wei, Z. Deng, Y. Lui, M. Kang, P. Lui, X. Yang, M. Pai and G. Zhang, J. Photochem. Photobiol., A, 2023, 437, 114470 CrossRef CAS.
- M. Barceló-Oliver, A. Terrón, A. García-Raso, I. Turel and M. Morell, Acta Crystallogr., Sect. E: Struct. Rep. Online, 2010, 66, m899 CrossRef PubMed.
- U. P. Singh, P. Tyagi and S. Pal, Inorg. Chim. Acta, 2009, 362, 4403 CrossRef CAS.
- A. M. Asiri, M. M. Al-Amari and S. A. Khan, J. Fluoresc., 2020, 30, 969 CrossRef CAS PubMed.
- Y. Zhang, H. Lui, W. Gao and S. Pu, RSC Adv., 2019, 9, 27476 RSC.
- H. Taş, J. Adams, J. C. Namyslo and A. Schmidt, RSC Adv., 2021, 11, 36450 RSC.
- Z. Shi, Y. Tu and S. Pu, RSC Adv., 2018, 8, 6727 RSC.
- E. Feng, Y. Tu, C. Fan, G. Liu and S. Pu, RSC Adv., 2017, 7, 50188 RSC.
- M. Akula, P. Z. El-Khoury, A. Nag and A. Bhattacharya, RSC Adv., 2014, 4, 25605 RSC.
- S. Lohar, S. Pal, M. Mukherjee, A. Maji, N. Demitri and P. Chattopadhyay, RSC Adv., 2017, 7, 25528 RSC.
- W.-J. Qu, J. Guan, T.-B. Wei, G.-T. Yan, Q. Lin and Y.-M. Zhang, RSC Adv., 2016, 6, 35804 RSC.
|
This journal is © The Royal Society of Chemistry 2024 |