DOI:
10.1039/D3RA08925H
(Paper)
RSC Adv., 2024,
14, 11728-11733
Organic dye-loaded reduced titanium dioxide as a broadband saturable absorber for ultrafast fiber lasers
Received
29th December 2023
, Accepted 1st April 2024
First published on 11th April 2024
Abstract
As a rising star among metal oxide nanomaterials, titanium dioxide (TiO2) has been widely investigated and employed in optical applications because of its excellent optical properties. In this work, we demonstrate the efficient and broadband nonlinear photonic properties of methylene blue (MB)-loaded reduced TiO2 (TiO2−x-MB) and explore the performance of a TiO2−x-MB-microfiber photonic device in broadband ultrafast photonics. Within an erbium-doped fiber laser (EDFL) system, utilizing the TiO2−x-MB-microfiber photonic device as a saturable absorber (SA), steady mode-locked pulses together with chaotic pulses were successfully achieved at the wavelength of 1.55 μm. Furthermore, by incorporating the TiO2−x-MB SA into a thulium-doped fiber laser (TDFL) system, an ultrashort single pulse and multiple pulses were obtained at 2.0 μm. These results indicate that TiO2−x-MB is an excellent nanomaterial for use in mode-locked lasers, being an alternative candidate for ultrafast fiber lasers via exploiting the chemical and physical properties of oxide nanomaterials.
1 Introduction
Ultrafast fiber lasers are widely used in various military and civilian applications,1–3 such as optical communication4 and precision machining.5,6 One of the main methods for generating ultrashort pulses is passive mode-locked technology, where the key is to introduce a saturable absorber (SA) into the laser cavity. Mode-locked fiber lasers can be realized using suitable materials as SAs, providing advantages in performance and output stability.6 Existing saturable absorber materials include semiconductor saturable absorber mirrors7,8 and two-dimensional materials represented by graphene,9,10 molybdenum disulfide (MoS2)11,12 and black phosphorus.13,14 In addition, various materials have been used for mode-locked devices in ultrafast lasers, including SnSe2,15 GeAs2,16 rGO-Co3O4 (ref. 17) and WCNs.18 However, the investigation of new materials for use as SAs is still in early stages. Therefore, it is necessary to explore novel materials as alternative SAs with excellent nonlinear optical properties for achieving mode-locked ultrashort pulse lasers.
Recently, titanium dioxide (TiO2) nanomaterials have been attracting increasing research interest because of their excellent photocatalytic properties, good biocompatibility and excellent chemical stability. Their potential application in all-optical switching devices has also received extensive attention, especially in thin film form.19 TiO2 sheets have strong planar bonding and weak van der Waals coupling, making them suitable for mechanical or chemical exfoliation. In 2015, Ahmad et al. obtained Q-switched pulses in an EDFL with TiO2 film as the SA.20 Afterwards, Reddy et al. demonstrated a passively mode-locked thulium-holmium-doped fiber laser based on a TiO2 SA and observed the ultrashort pulses.21 Further, TiO2-doped fiber (TiO2 DF) was utilized as a passive SA in an EDFL,22 providing a stable and self-powered mode-locked pulse laser under proper conditions. Thus, it is important to continue the research on ultrafast fiber lasers with TiO2 SAs in the 1.55 μm and 2.0 μm region, which is of great significance, due to the great potential in various fields, including supercontinuum generation23 and material processing.24
In this work, TiO2 was reduced into TiO2 nanoparticles (TiO2−x), which have a low band gap compared with undoped TiO2 particles. In addition, the organic dye methylene blue (MB) was used as a dopant to modify TiO2−x to form the TiO2−x-MB nanostructure, which further enhanced its working wavelength. Then, we fabricated the TiO2−x-MB nanomaterial by utilizing a liquid-phase exfoliation process. Furthermore, a TiO2−x-MB photonic device was constructed on microfiber via an optical deposition method, and the nonlinear optical absorption properties at 1.55 μm and 2 μm were evaluated. We then studied the performance of the TiO2−x-MB photonic device as a SA in EDFL and TDFL systems. Based on the outstanding saturable absorption of the TiO2−x-MB photonic device, we realized mode-locked pulses together with chaotic pulses in the EDFL. In addition, we obtained stable single pulses as well as multiple pulses in the TDFL using the TiO2−x-MB photonic device as the SA. Multi-pulse operation in a TDFL with TiO2−x-MB SA is reported for the first time. Our experimental results suggest that the TiO2−x-MB photonic device is suitable for efficient application in ultrafast photonics. Besides, this work will promote the exploration of the optical behavior of metal oxides and further applications of optimized nanomaterials in ultrafast fiber lasers.
2 Material preparation and characterization
2.1 Preparation and characterization of the TiO2−x-MB nanomaterial
The TiO2−x-MB nanomaterial was obtained via the introduction of Ti3+ ions on the surface of TiO2 by reducing TiO2 particles followed by modification with the organic dye MB.25,26 Briefly, 6 g of sodium borohydride was added to 60 mL of water, then 0.50 g of anatase TiO2 powder was added, and the hydrothermal reaction was carried out in an autoclave at 180 °C for 16 hours. Subsequently, the reaction solution was centrifuged (10
000 rpm) for 5 minutes, and the centrifuged TiO2−x powder doped with Ti3+ was alternately rinsed using deionized water and ethanol three times, with the TiO2−x powder and washing solution being separated by centrifugation each time. The TiO2−x powder was collected and further dried in air at 60 °C for 10 hours. Then, 250 mg of the TiO2−x powder was dispersed in 50 mL of 7 × 10−5 M MB solution (TiO2
:
MB = 250
:
1) and mixed using a magnetic stirrer at room temperature for 60 minutes. Upon reaction, the precipitate and solution were separated by centrifugation. The resulting precipitate was then rinsed with water and acetone three times alternately, then dried at 25 °C for 12 hours and ground to obtain the TiO2−x-MB nanomaterial.
Fig. 1(a) shows the absorption spectra for TiO2, TiO2−x and TiO2−x-MB, indicating that the prepared TiO2−x-MB material has good absorption in the 500–2000 nm region. The dynamic light scattering (DLS) characterization of TiO2, TiO2−x and TiO2−x-MB is shown in Fig. 1(b), which was carried out to measure their average particle size. As can be seen from the figure, the average particle size of TiO2−x without MB incorporation is 382 nm, which is close to the average particle size of unmodified TiO2 (363 nm), indicating that the nanoparticle size was maintained after the reduction of the TiO2 nanoparticles. The particle size of the prepared TiO2−x-MB is between 400 and 1100 nm, and the average particle size of the main component is 688 nm. The results show that the loading of MB obviously increases the particle size of the material. Scanning electron microscope (SEM) images of TiO2, TiO2−x, and TiO2−x-MB indicate that the modification of MB on the TiO2−x nanomaterial increases the particle size of the nanoparticles (Fig. 1(c–e)), which is consistent with DLS results. The high-resolution transmission electron microscopy (HRTEM) image of the prepared TiO2−x-MB nanostructure is shown in Fig. 1(f). The lattice stripes with interlayer distance of 0.350 nm can be observed from HRTEM, which corresponds to the (101) crystal surface of TiO2.
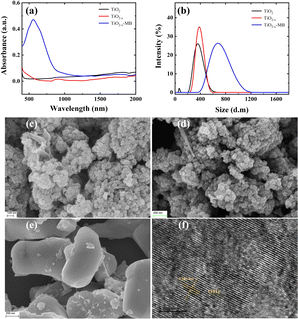 |
| Fig. 1 Characterization of the TiO2−x-MB nanomaterial. (a) Absorption spectra and (b) DLS spectra for TiO2, TiO2−x and TiO2−x-MB. SEM images of (c) TiO2, (d) TiO2−x, and (e) TiO2−x-MB. (f) HRTEM image of TiO2−x-MB. | |
2.2 Nonlinear optical properties of TiO2−x-MB SA
First, the above-prepared TiO2−x-MB nanomaterial was uniformly dispersed in isopropyl alcohol. Then, the TiO2−x-MB nanomaterial was deposited on a microfiber as an optical device using the optical deposition method.27 Herein, the microfiber is drawn from a standard single-mode fiber using a typical flame-brushing method. The fabricated microfiber has a diameter of approximately 12 μm and exhibits an insertion loss as low as ∼0.5 dB at 1550 nm. Because of the long interaction length, a strong evanescent field interaction occurs when the light propagates along the TiO2−x-MB sample, which significantly improves the damage threshold of the TiO2−x-MB SA. Afterwards, the nonlinear transport behavior of the TiO2−x-MB SA at different wavelengths was investigated by utilizing the twin-power-meter method.28 The input fiber laser sources are a homemade mode-locked EDFL (1565 nm, 98 MHz, 500 fs) and a TDFL (1957 nm, 12 MHz, 1 ps). The TiO2−x-MB SA displays typical nonlinear optical absorption properties, as shown in Fig. 2(a) and (b). The optical modulation depths (ΔT) of the TiO2−x-MB SAs are 7.1 % at 1.55 μm and 10.2 % at 2.0 μm, respectively, and corresponding non-saturable losses (αn) are 51.9% and 68.7%, respectively. These characteristics indicate that the TiO2−x-MB has typical nonlinear optical absorption and is suitable for application in ultrafast photonics.
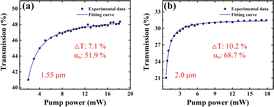 |
| Fig. 2 Nonlinear absorption transmission curves for TiO2−x-MB SA on a microfiber at (a) 1.55 μm and (b) 2 μm. | |
3 Ultrafast photonics applications
The fabricated TiO2−x-MB SA can be spliced into the EDFL and TDFL, as depicted in Fig. 3. The pump laser is launched into the gain fiber (3 m long EDF or 2.7 m long TDF) using a wavelength-division multiplexer (WDM). The central wavelengths of pump lasers are ∼980 nm for EDFL and ∼1570 nm for TDFL. A polarization-independent isolator (PI-ISO) was used to guarantee the unidirectional operation of the fiber laser. For the EDFL, the 30% port of the 3
:
7 optical coupler (OC) is used as the output. For the TDFL, the 20% port of the 2
:
8 OC is applied as the output. The homemade methylene blue (MB)-loaded reduced TiO2 (TiO2−x-MB) on microfiber is used as the saturable absorber (SA). A polarization controller (PC) with three paddles is used to finely adjust the intracavity polarization state. The total lengths of the EDFL and TDFL are ∼19.1 m and ∼21.4 m, respectively. The output pulses can be recorded using a commercial optical spectrum analyzer, oscilloscope, radio-frequency (RF) analyzer and autocorrelator.
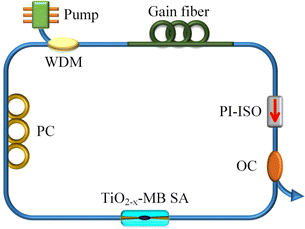 |
| Fig. 3 Schematic diagram of the ultrafast fiber laser setup with the TiO2−x-MB SA. | |
3.1 Mode-locked pulses and chaotic pulses in the EDFL
Because of the strong nonlinear optical absorption of the TiO2−x-MB SA, it is easy to obtain mode-locked pulses by properly adjusting the pump power and polarization controller (PC). The stable mode-locked pulses at a pump power of 40 mW are shown in Fig. 4. Clearly, the optical spectral center is about 1556.14 nm, and the 3 dB bandwidth is approximately 5.03 nm, as shown in Fig. 4(a). Moreover, typical sidebands are observed on the left and right of the optical spectrum. Besides, an obvious continuous wave (CW) peak can be seen at 1557.34 nm, resulting from the operation state with imperfect mode locking.11 The pulse trains are illustrated in Fig. 4(b). The temporal separation of the two pulses is ∼100.7 ns. Therefore, the corresponding repetition frequency is 9.93 MHz, matching the total cavity length. Fig. 4(c) presents the measured radio-frequency (RF) spectrum. Obviously, the signal-to-noise ratio (SNR) is ∼59.8 dB, indicating that the laser operation maintains a relatively steady state. The autocorrelation curve in Fig. 4(d) has a full width at half maximum of ∼1.51 ps, corresponding to a pulse duration of ∼978 fs if a sech2 fit is assumed. Thus, the time-bandwidth product (TBP) is higher than the transform-limiting of 0.315, indicating that the obtained pulses have a slight chirp.
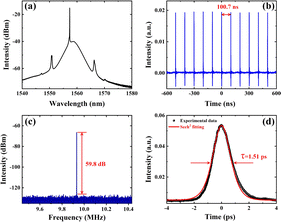 |
| Fig. 4 Mode-locked operation of the EDFL with the TiO2−x-MB SA. (a) Optical spectrum, (b) temporal trains, (c) RF spectrum, and (d) autocorrelation curve. | |
Further, chaotic-pulse bunches can be observed by suitably enhancing the pump power and adjusting the paddles of the PC due to the energy quantization of the ultrashort pulses under higher pump power.29 Fig. 5 depicts their output characteristics at 400 mW. The measured optical spectrum shows a center of 1558.84 nm with a 3 dB bandwidth of 0.2 nm, as illustrated in Fig. 5(a). In addition, no obvious sidebands were observed in the optical spectrum. The pulse sequence based on the 1 GHz low-speed oscilloscope is presented in Fig. 5(b). The temporal separation of 100.7 ns indicates that the pulse operation follows the fundamental frequency regime. We also measured temporal pulse trains using a 20 GHz real-time oscilloscope, as shown in Fig. 5(c). Several obvious multiple-pulse bunch envelopes can be seen in the temporal domain. Besides, the intensities of these bunches are chaotic and random. Furthermore, the details of a single envelope are shown in Fig. 5(d). It is obvious that the single multiple-pulse bunch envelope consists of many smaller pulses, where these random pulses have varying intensities with an irregular trend.
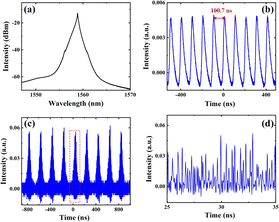 |
| Fig. 5 Chaotic-pulse operation of the EDFL with the TiO2−x-MB SA. (a) Optical spectrum, (b) temporal trains with low-speed oscilloscope, (c) temporal trains with high-speed oscilloscope, and (d) close-up view of the red dotted section in (c). | |
3.2 Ultrashort single pulse and multiple pulses in the TDFL
To dig into the nonlinear optical absorption behaviors of the as-fabricated TiO2−x-MB SA, especially the phenomenon when the wavelength is extended to the 2.0 μm region, a TDFL system was constructed, as shown in Fig. 3. By raising the pump power to 240 mW, it appears that the mechanism might be assigned to CW. Through manipulating accessories together with the power, steady mode-locked operation was obtained. Fig. 6 clearly shows the output pulse performance of this system at 300 mW. There are obvious Kelly sidebands located in the spectrum, as displayed in Fig. 6(a). As can be seen, the resulting spectrum is centered at 1888.8 nm, with a full width at half maximum of 2.65 nm. Furthermore, there are noticeable characteristic dips, which should be attributed to the absorption line for water from the atmosphere. Moreover, Fig. 6(b) confirmed that the temporal pulse has a separation of 103.3 ns, suggesting a repetition rate of 9.68 MHz, matching well with the cavity length. The operation steadiness of the laser is demonstrated, with the SNR of the recorded RF spectrum reaching ∼60.2 dB (Fig. 6(c)). In addition, to check the data quality of the output pulses, we recorded the autocorrelation trace shown in Fig. 6(d). The measured FWHM is 2.36 ps, which suggests that the pulse width of the autocorrelation curve should be 1.53 ps by assuming a sech2 intensity profile. Furthermore, the estimated TBP value is ∼0.340, manifesting a slightly chirped pulse. Moreover, the output performance of ultrafast fiber lasers based on various SAs reported in recent years is summarized in Table 1. As can be seen from this table, the TiO2−x-MB material can be used as an excellent broadband SA for obtaining ultrashort pulses in mode-locked fiber lasers.
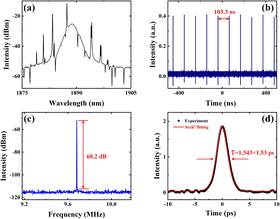 |
| Fig. 6 Single-pulse operation of the TDFL with the TiO2−x-MB SA. (a) Optical spectrum, (b) temporal trains, (c) RF spectrum, and (d) autocorrelation curve. | |
Table 1 Summary of the performance of different materials used as SAs in ultrafast fiber lasers at 1.55 μm and 2.0 μm
Material |
Wavelength (nm) |
Duration (ps) |
Frequency (MHz) |
Ref. |
NiS2 |
1560.2 |
0.524 |
21.1 |
30 |
Ti2CTx |
1530.85 |
0.265 |
19 |
31 |
SnSe2 |
1560.14 |
0.598 |
10.83 |
15 |
Graphdiyne |
1565.72 |
0.940 |
5.05 |
32 |
WS2 |
1557 |
1.32 |
8.86 |
33 |
TiO2−x-MB |
1556.14 |
0.978 |
9.93 |
This work |
WS2 |
1941 |
1.3 |
34.8 |
34 |
Bi2Te3 |
1909.5 |
1.26 |
21.5 |
35 |
Black phosphorus |
1898 |
1.58 |
19.2 |
36 |
WSe2 |
1863.96 |
1.16 |
11.36 |
37 |
SnSe |
1897 |
2.12 |
5.98 |
38 |
TiO2−x-MB |
1888.8 |
1.53 |
9.68 |
This work |
It is well-known that when the pump power is at a high level, the mode-locked fiber cavity easily generates complicated pulses because of the soliton energy quantization effect.29 Therefore, when enhancing the pump power continuously, our TDFL operation will exhibit a multiple-pulse state. The output optical spectrum of multiple pulses at 340 mW is displayed in Fig. 7(a). As shown, the spectrum is centered at 1888.63 nm, with a FWHM of 2.25 nm. Besides, Fig. 7(b) shows that the measured pulse signals largely rely on the high-speed oscilloscope. The corresponding details are depicted in Fig. 7(c), indicating that the output pulses are made up of six pulses. The first pulse distance is ∼1.37 ns, and the final one is ∼1.06 ns. The average pulse distance is about 1.05 ns. Obviously, the pulse distance trend is changeable. Further, optical spectra were studied at four pump powers, with the results presented in Fig. 8(a). Clearly, the spectral shape is almost unchanged, despite the enhanced pump power. The number of pulses in a single bunch of pulses at these pump powers was investigated, as illustrated in Fig. 8(b). With increasing pump power, a nearly linear increase in the number of pulses is observed from 1 to 12. Similar phenomena have been obtained in EDFLs.39–42 This is the first time that such an operation regime has been observed in a TDFL, to the best of our knowledge. The formation of this special pulse regime is closely associated with the slow recovery and depletion mechanism for the gain in the EDFL.39 When the pump power is elevated one step further, multiple pulses disappear, and the pulse operation becomes unstable, which may be attributed to the strong and weak interactions of these multiple pulses in this state.
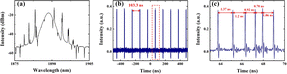 |
| Fig. 7 Multiple-pulse operation of the TDFL with the TiO2−x-MB SA. (a) Optical spectrum, (b) temporal trains with high-speed oscilloscope, and (c) close-up view of the red dotted section in (b). | |
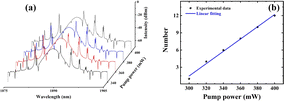 |
| Fig. 8 (a) Optical spectra and (b) pulse numbers with different pump powers. | |
4 Conclusions
In summary, this work has reported the fabrication and characterization of a TiO2−x-MB nanomaterial, and its application in nonlinear optics in ultrafast fiber lasers at 1.55 μm and 2.0 μm. Due to the excellent nonlinear optical characteristics of the TiO2−x-MB nanomaterial, a TiO2−x-MB-decorated microfiber device was fabricated and used as a SA. Using the TiO2−x-MB SA in an EDFL, stable mode-locked pulses and chaotic pulses were successfully obtained. Moreover, the TiO2−x-MB SA can be used in a TDFL, generating not only a single pulse but also multiple pulses. Our results suggest that TiO2−x-MB has promising potential for use in ultrafast fiber lasers, extending the application of these metal oxides, which are generally used as photocatalysts and providing efficient candidates for use in nonlinear photonics.
Author contributions
Xiaochan Lu and Shuang Li: conceptualization, investigation, writing – original draft preparation. Miao Yan and Jianming Chen: investigation, formal analysis. Tingting Deng: data curation, methodology. Guohui Nie and Zhenhong Wang: supervision. Hu Liang and Bin Zhang: resources, funding acquisition.
Conflicts of interest
The authors declare that they have no known competing financial interests or personal relationships that could have appeared to influence the work reported in this paper.
Acknowledgements
This work was supported by the National Key Research and Development Project (Grant No. 2022YFA0912500, 2020YFC2005205, and 2020YFC2005200), National Natural Science Foundation of China (Grant No. 52203335, 62005178, 82002936, 62105307, 82192865 and 81970875), Guangdong Basic and Applied Basic Research Foundation (Grant No. 2023A1515010093, 2023A1515220240), Shenzhen Science and Technology Innovation Committee (Grant No. ZDSYS201707281114196, KCXFZ20201221173413038, LCYSSQ20220823091403007, JCYJ20190806163209126, JCYJ20190808143611709, JCYJ20200109105216803, JCYJ20220809170611004 and JCYJ20220530150414031), Sanming Project of Medicine in Shenzhen (Grant No. SZSM202211022), and Development and Reform Commission of Shenzhen Municipality.
Notes and references
- M. E. Fermann and I. Hartl, Nat. Photonics, 2013, 7, 868–874 CrossRef CAS.
- J. Ma, Z. Qin, G. Xie, L. Qian and D. Tang, Appl. Phys. Rev., 2019, 6, 021317 Search PubMed.
- G. Chang and Z. Wei, iScience, 2020, 23, 101101 CrossRef CAS PubMed.
- K. Anbarasi, C. Hemanth and R. G. Sangeetha, Opt. Laser Technol., 2017, 97, 161–171 CrossRef.
- Z. Lin and M. Hong, Ultrafast Sci., 2021, 2021, 9783514 Search PubMed.
- T. Jiang, K. Yin, C. Wang, J. You, H. Ouyang, R. Miao, C. Zhang, K. Wei, H. Li, H. Chen, R. Zhang, X. Zheng, Z. Xu, X. Cheng and H. Zhang, Photonics Res., 2020, 8, 78–90 CrossRef CAS.
- L. M. Zhao, D. Y. Tang, X. Wu, H. Zhang and H. Y. Tam, Opt. Lett., 2009, 34, 3059–3061 CrossRef CAS PubMed.
- Y. Mashiko, E. Fujita and M. Tokurakawa, Opt. Express, 2016, 24, 26515–26520 CrossRef CAS PubMed.
- Q. Bao, H. Zhang, Y. Wang, Z. Ni, Y. Yan, Z. X. Shen, K. P. Loh and D. Y. Tang, Adv. Funct. Mater., 2009, 19, 3077–3083 CrossRef CAS.
- M. Pawliszewska, T. Martynkien, A. Przewłoka and J. Sotor, Opt. Lett., 2018, 43, 38–41 CrossRef CAS PubMed.
- H. Xia, H. Li, C. Lan, C. Li, J. Du, S. Zhang and Y. Liu, Photonics Res., 2015, 3, A92–A96 CrossRef CAS.
- Y. Zhang, J. Zhu, P. Li, X. Wang, H. Yu, K. Xiao, C. Li and G. Zhang, Opt. Commun., 2018, 413, 236–241 CrossRef CAS.
- M. Zhang, Q. Wu, F. Zhang, L. Chen, X. Jin, Y. Hu, Z. Zheng and H. Zhang, Adv. Opt. Mater., 2019, 7, 1800224 CrossRef.
- K. Park, J. Lee, Y. T. Lee, W.-K. Choi, J. H. Lee and Y.-W. Song, Ann. Phys., 2015, 527, 770–776 CrossRef CAS.
- Z. Wang, B. Zhang, B. Hu, Z. Li, C. Ma, Y. Chen, Y. Song, H. Zhang, J. Liu and G. Nie, Photonics Res., 2020, 8, 1687–1696 CrossRef CAS.
- S. Liu, G. Li, F. Zhu, H. Huang, J. Lu, J. Qu, L. Li and Q. Wen, Adv. Funct. Mater., 2022, 32, 2112252 CrossRef CAS.
- M. An, Z. Pan, X. Li, W. Wang, C. Jiang, G. Li, P. Guo, H. Lu, Y. Han, X. Chen and Z. Zhang, ACS Appl. Mater. Interfaces, 2022, 14, 53971–53980 CrossRef CAS PubMed.
- F. Wang, D. Lan, J. Zhao, Y. Qu, X. Zhou, X. Zhang and T. Cheng, Opt. Laser Technol., 2023, 158, 108778 CrossRef CAS.
- H. Long, A. Chen, G. Yang, Y. Li and P. Lu, Thin Solid Films, 2009, 517, 5601–5604 CrossRef CAS.
- H. Ahmad, S. A. Reduan, Z. A. Ali, M. A. Ismail, N. E. Ruslan, C. S. J. Lee, R. Puteh and S. W. Harun, IEEE Photonics J., 2016, 8, 1500107 Search PubMed.
- P. H. Reddy, M. F. A. Rahman, M. C. Paul, A. A. Latiff, A. H. A. Rosol, S. Das, A. Dhar, S. K. Bhadra, K. Dimyati and S. W. Harun, Optik, 2018, 158, 1327–1333 CrossRef CAS.
- M. F. Mohd Rusdi, A. A. Latiff, M. C. Paul, S. Das, A. Dhar, H. Ahmad and S. W. Harun, Opt. Laser Technol., 2017, 89, 16–20 CrossRef CAS.
- Z. Zheng, D. Ouyang, J. Zhao, M. Liu, S. Ruan, P. Yan and J. Wang, Photonics Res., 2016, 4, 135–139 CrossRef CAS.
- I. Mingareev, N. Gehlich, T. Bonhoff, A. Abdulfattah, A. M. Sincore, P. Kadwani, L. Shah and M. Richardson, Int. J. Adv. Des. Manuf. Technol., 2016, 84, 2567–2578 CrossRef.
- R. Ren, Z. Wen, S. Cui, Y. Hou, X. Guo and J. Chen, Sci. Rep., 2015, 5, 10714 CrossRef CAS PubMed.
- E. Savinkina, L. Obolenskaya and G. Kuzmicheva, Appl. Nanosci., 2015, 5, 125–133 CrossRef CAS.
- Y. Song, K. You, Y. Chen, J. Zhao, X. Jiang, Y. Ge, Y. Wang, J. Zheng, C. Xing and H. Zhang, Nanoscale, 2019, 11, 12595–12602 RSC.
- Z.-C. Luo, M. Liu, H. Liu, X.-W. Zheng, A.-P. Luo, C.-J. Zhao, H. Zhang, S.-C. Wen and W.-C. Xu, Opt. Lett., 2013, 38, 5212–5215 CrossRef PubMed.
- D. Y. Tang, L. M. Zhao, B. Zhao and A. Q. Liu, Phys. Rev. A, 2005, 72, 043816 CrossRef.
- P. Wang, H. Zhang, Y. Yin, Q. Ouyang, Y. Chen, E. Lewis, G. Farrell, M. Tokurakawa, S. Wadi Harun, C. Wang and S. Li, Opt. Laser Technol., 2020, 132, 106492 CrossRef CAS.
- Y. Shi, N. Xu and Q. Wen, J. Lightwave Technol., 2020, 38, 1975–1980 CAS.
- J. Guo, Z. Wang, R. Shi, Y. Zhang, Z. He, L. Gao, R. Wang, Y. Shu, C. Ma, Y. Ge, Y. Song, D. Fan, J. Xu and H. Zhang, Adv. Opt. Mater., 2020, 8, 2000067 CrossRef CAS.
- D. Mao, Y. Wang, C. Ma, L. Han, B. Jiang, X. Gan, S. Hua, W. Zhang, T. Mei and J. Zhao, Sci. Rep., 2015, 5, 7965 CrossRef CAS PubMed.
- M. Jung, J. Lee, J. Park, J. Koo, Y. M. Jhon and J. H. Lee, Opt. Express, 2015, 23, 19996–20006 CrossRef CAS PubMed.
- K. Yin, B. Zhang, L. Li, T. Jiang, X. Zhou and J. Hou, Photonics Res., 2015, 3, 72–76 CrossRef CAS.
- H. Yu, X. Zheng, K. Yin, X. a. Cheng and T. Jiang, Appl. Opt., 2015, 54, 10290–10294 CrossRef CAS PubMed.
- J. Wang, W. Lu, J. Li, H. Chen, Z. Jiang, J. Wang, W. Zhang, M. Zhang, I. L. Li, Z. Xu, W. Liu and P. Yan, IEEE J. Sel. Top. Quantum Electron., 2018, 24, 1–6 CAS.
- Z. Wang, F. Li, J. Guo, C. Ma, Y. Song, Z. He, J. Liu, Y. Zhang, D. Li and H. Zhang, Adv. Opt. Mater., 2020, 8, 1902183 CrossRef CAS.
- A. Zaviyalov, P. Grelu and F. Lederer, Opt. Lett., 2012, 37, 175–177 CrossRef CAS PubMed.
- Z. Wang, Z. Wang, Y. g. Liu, R. He, G. Wang, G. Yang and S. J. C. O. L. Han, Chin. Opt. Lett., 2017, 15, 080605 CrossRef.
- Z. Wang, X. Wang, Y. Song, J. Liu and H. Zhang, Phys. Rev. A, 2020, 101, 013825 CrossRef CAS.
- Y.-T. Yang, Y. Zou, Q. Zeng, Y.-F. Song, K. Wang and Z.-H. Wang, Acta Phys. Sin., 2022, 71, 134201–134208 CrossRef.
Footnote |
† These authors contributed equally to this work. |
|
This journal is © The Royal Society of Chemistry 2024 |