DOI:
10.1039/D3RA07928G
(Paper)
RSC Adv., 2024,
14, 3712-3722
New insights on the optical properties and upconversion fluorescence of Er-doped CoAl2O4 nanocrystals
Received
19th November 2023
, Accepted 14th January 2024
First published on 24th January 2024
Abstract
In this study, Er-doped CoAl2O4 nanocrystals (NCs) were synthesized via co-precipitation. All the NCs were crystallized in the form of a single phase with a spinel structure and Er3+ ions replaced Al3+ ions in the formation of the CoAl2−xErxO4 alloy structure. The optical characteristics of the Er3+ ion-doped CoAl2O4 NCs were thoroughly investigated by analyzing both the UV-VIS and photoluminescence spectra, using the Judd–Ofelt theory. The effect of Er doping content on the luminescent properties of the CoAl2O4 pigment (using lasers emitting at wavelengths of 413 and 978 nm) has been studied. The values of Judd–Oflet intensity parameters (Ω2, Ω4, and Ω6) were determined from the absorption spectra using the least square fitting method. The J–O parameters were calculated and compared with those of other host materials; the values of the Ω2, Ω4, and Ω6 parameters decreased with an increase in Er concentration. This suggests that the rigidity and local symmetry of the host materials become weaker as the concentration of Er3+ ions increases. The highest value of the Ω2 parameter, when compared with Ω4 and Ω6, suggests that the vibrational frequencies in the given samples are relatively low. The upconversion fluorescence phenomenon was observed and explained in detail under an excitation wavelength of 978 nm when the excitation power was varied.
Introduction
Spinel-structured oxides with the general chemical formula AB2O4 are widely employed as ceramic pigments, magnetic materials, catalysts, and more.1,2 Among all spinel oxides, cobalt aluminate (CoAl2O4), also known Thenard's blue, is an inorganic blue pigment. Within this pigment, Co2+ ions are situated in tetrahedral positions, whereas Al3+ ions occupy octahedral positions.3 This special CoAl2O4 blue pigment has played an important role in color printing technology for materials such as plastics, paints, glass, enamels, and ceramics. In addition to its brilliant blue color, this inorganic CoAl2O4 pigment exhibits remarkable resistance to both acids and alkalis, along with enhanced thermal stability.4
CoAl2O4 is a superparamagnetic single-domain crystal material at room temperature.5 Research has mainly focused on the absorption properties of CoAl2O4 and there are very few studies on the fluorescence properties of CoAl2O4 owing to its weak emission ability. The vibrancy of CoAl2O4 pigments can be compromised because of their susceptibility to contain Co3O4 phase impurities, which impart a black hue.4,5 The coloration of a ceramic pigment is intricately linked to factors such as its composition, crystal structure, grain size, and micro-morphology.2–4 The impact of the preparation process of the ceramic pigment on its microstructure and color performance is important for enhancing its chromatic richness and broadening its range of applications.3–5 Some studies have doped transition metals and rare earth ions into the CoAl2O4 host to change the color and enhance the luminescence of the material. Y. Tong et al. doped Eu3+ ions into a CoAl2O4 host and found that CoAl1.95Eu0.05O4 NCs can be considered a good “colored cool pigment” candidate for use in surface coating applications.6 Ce and Mn co-doped CoAl2O4 NCs were successfully synthesized using a novel wet chemical method.7 The introduction of Ce and Mn ions into CoAl2O4 enhanced its photoluminescence properties without altering the cubic structure of the host. The improved photoluminescence performance of Ce and Mn co-doped CoAl2O4 is not attributed to energy transfer between Ce4+ and Mn4+; rather, it is influenced by surface or impurity defects. Strontium-doped cobalt aluminate NCs were fabricated using Co1−xSrxAl2O4 L-alanine as a fuel in an ignition cycle.8 CoAl2O4 and Ni-doped CoAl2O4 were synthesized using the pechini route.9 The breakdown of the colored and colorless organic dyes under natural sunlight was investigated using the prepared photocatalysts. Ni-doped CoAl2O4 exhibited greater photocatalytic activity than undoped CoAl2O4.
Up-conversion luminescence is a phenomenon in which light is emitted at a wavelength shorter than the wavelength of the incident radiation. The up-conversion emission mechanism is often observed in certain materials, such as host materials doped with rare-earth ions (e.g., erbium, ytterbium, and holmium). This phenomenon originates from the absorption of two or more photons and is commonly observed in rare-earth ions, that possess intricate energy-level structures.10,11 Rare earth elements exhibit fluorescence spectra at various energy levels, providing a wide range of wavelengths, making them valuable luminescent materials.12 Er3+ ions are particularly intriguing because they can achieve up-conversion luminescence without the need for a sensitizer like some other rare earth ions.13,14 The intricate configuration of their electronic energy levels enables the detection of transitions at various wavelengths. When stimulated by infrared radiation, Er3+ ions exhibit diverse color emissions, rendering them significant luminescent ions in the mid-infrared spectrum, and leading to a multitude of applications.15 Er3+ ions can emit both green and red light when excited by infrared radiation.16,17 Up-conversion materials have applications in various fields, including photovoltaics, bioimaging, and laser technology.
Some studies have been conducted on the optical properties of rare earth ions-doped CoAl2O4 NCs.6–9 However, there has been no research related to the optical parameters of CoAl2O4 NCs doped with Er3+ ions, particularly using the Judd–Ofelt theory and their upconversion fluorescence. In this study, for the first time the local environment around Er3+ ions, the bond between Er3+ and ligands, and the local symmetry of the host material CoAl2O4 were studied using J–O theory. The nature of the upconversion fluorescence mechanism of Er ion in the CoAl2O4 host (using an excitation wavelength of 978 nm) has been studied and quantitatively calculated by changing the excitation power. The effect of Er doping content on the luminescent properties of the CoAl2O4 pigment (using lasers emitting at wavelengths of 413 and 978 nm) has been studied. The development of these new ceramic pigments has scientific significance and practical value.
Experimental description
Synthesis of Er-doped CoAl2O4 spinel nanocrystals
In our experiment, 0.06 mol Al(NO3)3·9H2O and 0.03 mol Co(NO3)2·6H2O were dissolved in 100 ml deionized water under magnetic stirring at 70 °C for 20 min. Er(NO3)3·5H2O was then added to the above solution. The Er3+ concentration depended on the Er3+/Al3+ ratio. Then NH3 solution was added to the above solution to adjust the pH value to approximately 10 and continued stirring the solution at 70 °C until a pink xerogel formed. The xerogel was then heat treated at 200 °C for 2 h to remove a significant portion of the organic solvent and water. Finally, the dried xerogel was ground into a fine powder and calcined for 3 h at 800 °C in air. Schematic flow chart for the synthesis of Er-doped CoAl2O4 NCs is observed in Fig. 1. The evolution process can be depicted by the following reactions: |
NH3 + H2O → NH4+ + OH−
| (1) |
|
Al3+ + 3OH− → Al(OH)3
| (2) |
|
Al(OH)3 + OH− → 2H2O + AlO2−
| (3) |
|
Co2+ + 2OH− → Co(OH)2
| (4) |
|
Er3+ + 3OH− → Er(OH)3
| (5) |
|
2Al(OH)3 → Al2O3 + 3H2O
| (6) |
|
2Er(OH)3 → Er2O3 + 3H2O
| (8) |
|
2CoO + (2−x)Al2O3 + xEr2O3 → 2CoAl2−xErxO4
| (9) |
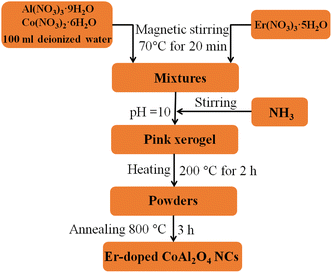 |
| Fig. 1 Schematic flow chart for the synthesis of Er-doped CoAl2O4 NCs. | |
Characterization
The crystal structures of the synthesized nanocrystals (NCs) were analyzed using an X-ray diffraction (Siemen, D5005) equipped with a Cu-Kα radiation source (λ = 1.5406 Å) within the 2θ range of 20° to 80°. The ultraviolet-visible (UV-vis) absorption spectra of the NCs were measured using a Jasco V-770 double-beam spectrophotometer, covering a wavelength range of 190 to 2700 nm. The morphology of NCs was checked by transmission electron microscopy (TEM, Joel-JEM 1010) operating at 80 kV. Photoluminescence (PL) spectra were acquired using the spectrophotometric system FLS1000 with a 450 W Xe lamp and the MicroSpec-2300i spectrometer with a He–Cd laser as the excitation source, and the excitation power (Pex) was adjusted from 5 × 10−4 to 5.6 mW. The Raman scattering (RS) spectra of the samples were recorded using a LABRAM-HR800 spectrometer (Jobin Yvon) with a wavelength of λ = 488 nm. X-ray photoelectron spectroscopy (XPS) was performed using a Thermo VG Escalab 250 photoelectron spectrometer.
Results and discussion
Morphological
TEM images of three typical CoAl2O4, Er1%-doped CoAl2O4, and Er5%-doped CoAl2O4 samples are shown in Fig. 2. TEM images showed that the obtained NCs were a nearly spherical shape with fairly uniform sizes. The sizes of the particles were mostly in the range of 30 to 40 nm. The observation results show that Er doping did not significantly change the size and shape of the Er-doped CoAl2O4 alloy NCs.
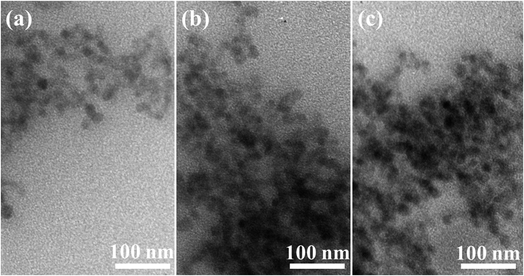 |
| Fig. 2 TEM images of NCs: (a) CoAl2O4, (b) Er1%-doped CoAl2O4, and (c) Er5%-doped CoAl2O4. | |
X-ray diffraction studies
Fig. 3 shows the XRD patterns of the CoAl2O4 and Er-doped CoAl2O4 NCs with different Er concentrations. All the nanocrystals (NCs) exhibited single-phase crystallization with a spinel structure (card JCPDS No. 44-0160) and a space group of Fd
mz.18 In the case of pure CoAl2O4 NCs, the diffraction peaks observed at 2θ angles correspond to 31.89, 36.68, 45.06, 56.27, 59.86, and 65.84°, indicating the lattice planes (220), (311), (400), (422), (511) and (440) of CoAl2O4. After doping with Er, only a CoAl2O4 spinel structure was obtained, with no detectable presence of Er oxide. This suggests that all Er was successfully incorporated into the CoAl2O4 lattice, without generating any oxide. The diffraction peaks of the Er-doped CoAl2O4 NCs shifted slightly toward smaller 2θ angles with increasing Er concentration. This result can be explained by the replacement of Al3+ with Er3+ ions to produce CoAl2−xErxO4 alloy NCs. Er3+ ions replaced Al3+ ions in the formation of the CoAl2−xErxO4 alloy structure (see in Fig. 4) because of the principle of preferential substitution based on similar valence states and analysis of element valence states.4,19
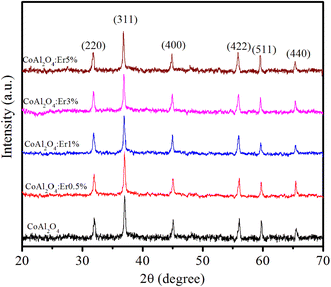 |
| Fig. 3 XRD patterns of Er-doped CoAl2O4 nanocrystals with varying Er concentrations. | |
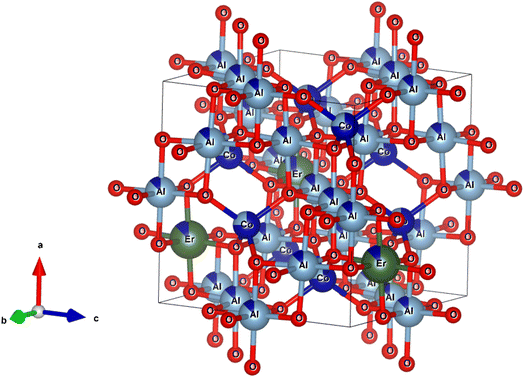 |
| Fig. 4 The unit-cells scheme of Er-doped CoAl2O4 with spinel structure. | |
According to Pauling's rule, Er has a coordination number of 6, resulting in the formation of an octahedral structure denoted as ErO6. The radii of ions Co2+, Al3+, and Er3+ are 0.54, 0.675, and 1.03 Å, respectively.19 Owing to the significantly larger ionic radius of Er3+ compared to Co2+, an increase in the Er doping concentration results in an expansion of the lattice parameters of CoAl2O4. This observation strongly suggests that Er doping led to lattice expansion. The effective crystallite strain in the NCs was determined using the Stokes–Wilson equation:20
|
 | (10) |
where
θ is the diffraction angle, and
β is the broadening of the diffraction line (measured at half of its maximum intensity). The average crystallite size (
D) of the NCs was calculated using Debye–Scherrer equation:
21 |
 | (11) |
where
k is the constant and usually a value of ∼0.9,
λ is the wavelength of the Cu = K
α radiation (∼1.54 Å), The average crystallite size of the NCs was calculated based on the line broadening of the (311) peak.
The unit cell parameters of the Er-doped CoAl2O4 NCs at different Er concentrations were calculated using the following equation:20,21
|
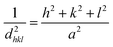 | (12) |
where
h,
k and
l are the Miller indices,
dhkl and
a are the interplanar spacing of the crystal and the lattice parameter, respectively.
dhkl was calculated using Bragg's equation:
20,21 |
nλ = 2dhkl sin θ
| (13) |
The unit cell volume of the NCs with a cubic structure was calculated using the following equation:
The crystal lattice parameters of the Er-doped CoAl2O4 NCs NCs are calculated and given in Table 1.
Table 1 The diffraction angle (2θ), lattice constants (a), cell volume (V), β, crystallite size (D), crystallite strain (ε) of NCs
Sample |
2θ (311) |
β × 10−2 (rad) |
a (Å) |
V (Å3) |
D (nm) |
ε × 10−3 |
CoAl2O4 |
36.831 |
0.575 |
8.094 |
530.360 |
25.421 |
1.364 |
CoAl2O4:Er3+0.5% |
36.712 |
0.584 |
8.095 |
530.442 |
25.034 |
1.386 |
CoAl2O4:Er3+1% |
36.654 |
0.596 |
8.097 |
530.810 |
24.505 |
1.414 |
CoAl2O4:Er3+3% |
36.530 |
0.583 |
8.100 |
531.344 |
25.065 |
1.384 |
CoAl2O4:Er3+5% |
36.473 |
0.579 |
8.107 |
532.823 |
25.235 |
1.375 |
Elemental and chemical composition analysis
XPS is a versatile analytical technique that provides valuable information regarding the elemental composition, chemical state, electronic structure, and depth profiles of materials. In our study, XPS was used to analyze the chemical states of the elements present in the surface region of the synthesized sample, namely the Er5%-doped CoAl2O4 NCs. The results are shown in Fig. 5. Fig. 5a shows typical XPS survey scans of the Er1%-doped CoAl2O4 sample. The XPS survey spectrum suggests that, apart from the original components, only traces of contaminated carbon were detected, with no evidence of any other elements. The XPS survey scan shows seven peaks corresponding to the levels of Al-2p, Al-2s, Er-4d, C-1s, O-1s, Co-2p, and Co-2s. Fig. 5b shows the high-resolution XPS spectrum of Co-2p. It has two peaks centered at 779.8 and 795.7 eV, which correspond to Co-2p3/2 and Co-2p1/2, respectively. The energy difference between the two peaks is 15.9 eV, which is a characteristic feature indicative of the presence of Co2+ ions. The Co-2p spectrum is relatively narrow and symmetrical, indicating that Co2+ occupies octahedral sites in the synthesized samples.22 Fig. 5c shows the high-resolution XPS spectrum of Al-2p. According to the study by Duan et al.,23 the Al-2p binding energies for octahedral and tetrahedral Al3+ ions are reported as 74.13 and 73.26 eV, respectively. In our study, the Al-2p binding energy peak fell within this range, suggesting the distribution of Al3+ ions among the two distinct sites within the CoAl2O4 NCs. The binding energy peak at 531.3 eV (Fig. 5d) is indexed to the O-1s level. The O-1s peak exhibits asymmetry and the binding energy of O-1s is similar to that of O in bulk CoAl2O4, as reported by Patterson et al.24 Fig. 5e shows a high-resolution Er 4d XPS spectrum. The presence of two peaks at 164.3 and 168.9 eV indicates the Erbium in the trivalent state within the Er-doped CoAl2O4 NCs.4,19
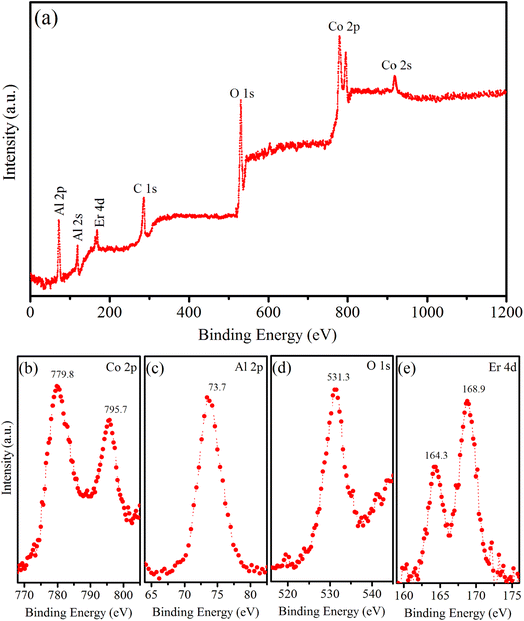 |
| Fig. 5 (a) Survey XPS spectrum of Er5%-doped CoAl2O4 NCs, (b) Co 2p, (c) Al 2p, (d) O 1s, and (e) Er 4d. | |
Absorption spectra and Judd–Ofelt analysis
To investigate the relationship between the cation distribution and optical characteristics of the NCs, we conducted measurements of the absorption spectra of the samples. The optical absorption spectra of the CoAl2O4 and Er-doped CoAl2O4 NCs were measured in the 300–1600 nm range, as shown in Fig. 6. The absorption spectra of the CoAl2O4 NCs showed three characteristic absorption peaks at 548, 595, and 638 nm, which indicate of the presence of Co2+ ions arranged in a ligand field with a 3d7 electron configuration.25 According to the literature, these three peaks are attributed to 4A2(F) → 4T1(P) transitions that arise due to the Jahn–Teller distortion of the Td structure, as indicated by ref. 26. The absorption spectra of the Er-doped CoAl2O4 NCs showed 12 peaks, corresponding to the transitions from the ground level to excited levels of Er3+ ion:14–16 4I15/2–4G9/2, 4I15/2–4G11/2, 4I15/2–2H9/2, 4I15/2–4F3/2, 4I15/2–4F5/2, 4I15/2–4F7/2, 4I15/2–2H11/2, 4I15/2–4S3/2, 4I15/2–4F9/2, 4I15/2–4I9/2, 4I15/2–4I11/2, and 4I15/2–4I13/2. These peaks were centered at 365, 383, 413, 441, 452, 497, 520, 543, 651, 797, 978, and 1540 nm, respectively.
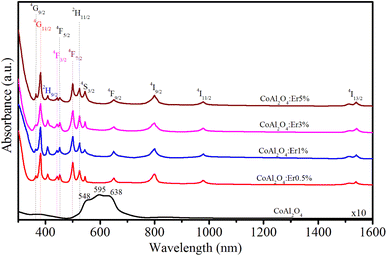 |
| Fig. 6 Absorption spectra of Er-doped CoAl2O4 NCs with varying Er concentrations. | |
The formula for estimating the intensity of a transition in RE3+ ions based on the oscillator strength from the absorption spectra is as follows:27,28
|
 | (15) |
where
α(
ν) is molar extinction coefficient at energy
ν (cm
−1). The values of
α(
ν) can be calculated from absorbance
A by using Lambert–Beer's law:
27,28where
c is concentration (mol dm
−3),
d is the optical path length (cm).
In the case of RE3+ ions, Judd–Ofelt (J–O) theory has proven to be a valuable tool for estimating both the nature of the ligand field and their radiative properties. The cornerstone of J–O theory is a set of three intensity parameters, denoted as Ωλ (λ = 2, 4, 6), which are related to the oscillator strength through the following expression:27,28
|
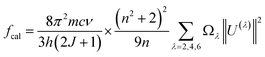 | (17) |
where
n represents the refractive index of the material,
J stands for the total angular momentum of the ground state,
Ωλ (
λ = 2, 4, 6) are the intensity parameters, and ‖
Uλ‖
2 are the squared doubly reduced matrix elements of the unit tensor operator with a rank of
λ = 2, 4, 6. These matrix elements are essentially host matrix-independent and can be found in
27. The refractive index of the CoAl
2O
4 host (
n) is approximately 1.4 and is considered constant across all wavelengths.
29 The intensity parameters for the Er
3+ ions in the CoAl
2O
4 NCs were determined using the least squares method by solving the equation
fcal =
fexp. The obtained results have been presented in
Table 2 for comparison with the values associated with other matrices.
Table 2 fexp, fcal (×10−6) for excited levels of Er+3 ions in the Er-doped CoAl2O4 NCs
Transition from 4I15/2 to |
λ (nm) |
CoAl2O4:Er3+0.5% |
CoAl2O4:Er3+1% |
CoAl2O4:Er3+3% |
CoAl2O4:Er3+5% |
fexp |
fcal |
fexp |
fcal |
fexp |
fcal |
fexp |
fcal |
4G9/2 |
365 |
1.56 |
1.43 |
1.21 |
1.14 |
0.89 |
1.05 |
0.94 |
1.13 |
4G11/2 |
383 |
10.92 |
10.9 |
10.67 |
10.74 |
10.65 |
10.11 |
10.35 |
10.42 |
2H9/2 |
413 |
4.45 |
4.36 |
4.47 |
4.61 |
4.52 |
4.61 |
4.21 |
4.38 |
4F3/2 |
441 |
0.1 |
0.23 |
0.13 |
0.2 |
0.32 |
0.49 |
0.43 |
0.31 |
4F5/2 |
452 |
0.36 |
0.52 |
0.27 |
0.35 |
0.31 |
0.56 |
0.62 |
0.53 |
4F7/2 |
497 |
1.21 |
1.46 |
1.3 |
1.53 |
1.17 |
1.23 |
1.47 |
1.32 |
2H11/2 |
520 |
5.67 |
5.54 |
5.83 |
5.74 |
5.91 |
5.62 |
5.89 |
6.83 |
4S3/2 |
543 |
0.19 |
0.32 |
0.22 |
0.43 |
0.26 |
0.47 |
0.37 |
0.33 |
4F9/2 |
651 |
1.52 |
1.32 |
1.63 |
1.52 |
1.87 |
1.58 |
1.63 |
1.61 |
4I9/2 |
797 |
0.27 |
0.28 |
0.21 |
0.27 |
0.25 |
0.24 |
0.28 |
0.26 |
4I11/2 |
978 |
0.34 |
0.36 |
0.41 |
0.44 |
0.37 |
0.38 |
0.4 |
0.34 |
4I13/2 |
1540 |
0.96 |
0.63 |
1.24 |
1.02 |
1.27 |
1.22 |
1.08 |
1.12 |
Judd–Oflet intensity parameters (Ω2, Ω4, and Ω6) were determined from the absorption spectra using the least square fitting method. It is known that the Ω2, Ω4, and Ω6 parameters can provide valuable insights into the local coordination around RE3+ ions.30–33 The Ω2 parameter is related to the covalency of the bond between RE3+ and ligands, as well as ligand asymmetry, with larger Ω2 values indicating stronger binding. This is related to the electric-dipole transitions. Meanwhile, Ω4 and Ω6 are affected by factors such as viscosity and hardness but are almost unaffected by the local environment. Furthermore, Ω6 exhibits an inverse relationship with the covalent nature of the (Er–O) bonds. To evaluate the stimulated emission in the laser medium, a spectroscopic quality factor, denoted as R and defined as R = Ω4/Ω6, was introduced. R is important for predicting the potential performance of lasers.31,32 In Er3+-doped samples, R typically falls within the range of 0.33–2.94.30–37 This study revealed a decreasing trend in R with an increasing concentration of Er3+ ions. The J–O parameters (Ω2, Ω4, Ω6, and R) were calculated and compared with those of the other host materials as shown in Table 3. This study shows that the order of the Judd–Oflet parameters is Ω2 > Ω6 > Ω4 when the Er doping concentration changes. This trend is similar to that of other host materials doped with Er3+ ions, such as LaF3, phosphate, ErF3 (8% mol), and tellurite,30–33 but it is different from host materials doped with Er3+ ions, such as germanate, NaYF4, antimony, and LYB crystal.34–37 From the results shown in Table 3, we can see that the values of the Ωλ parameters decrease with an increase in Er concentration. This suggests that the rigidity and local symmetry of the host materials become weaker as the concentration of the Er3+ ions increases. The highest value of the Ω2 parameter, when compared with Ω4 and Ω6, suggests that the vibrational frequencies in the given samples are relatively low.31,32,34
Table 3 Judd–Ofelt parameters: Ω2, Ω4, Ω6 (×10−20 cm2) and R of Er+3 in the samples
Sample |
Ω2 |
Ω4 |
Ω6 |
R |
Trend |
Reference |
CoAl2O4:Er3+0.5% |
2.73 |
0.84 |
0.95 |
0.88 |
Ω2 >Ω6 > Ω4 |
Present work |
CoAl2O4:Er3+1% |
2.59 |
0.56 |
0.72 |
0.78 |
Ω2 >Ω6 > Ω4 |
Present work |
CoAl2O4:Er3+3% |
2.32 |
0.42 |
0.61 |
0.69 |
Ω2 >Ω6 > Ω4 |
Present work |
CoAl2O4:Er3+5% |
2.24 |
0.35 |
0.52 |
0.67 |
Ω2 >Ω6 > Ω4 |
Present work |
LaF3 |
1.27 |
0.28 |
0.63 |
0.44 |
Ω2 >Ω6 > Ω4 |
30 |
Phosphate |
3.91 |
1.97 |
2.57 |
0.76 |
Ω2 >Ω6 > Ω4 |
31 |
Tellurite |
4.93 |
1.30 |
1.31 |
0.99 |
Ω2 >Ω6 > Ω4 |
32 |
ErF3 (8%mol) |
0.61 |
0.14 |
0.43 |
0.33 |
Ω2 >Ω6 > Ω4 |
33 |
Germanate |
4.81 |
1.41 |
0.48 |
2.94 |
Ω2 >Ω4 > Ω6 |
34 |
NaYF4 |
2.16 |
1.40 |
0.64 |
2.19 |
Ω2 >Ω4 > Ω6 |
35 |
Antimony |
4.05 |
1.14 |
0.73 |
1.56 |
Ω2 >Ω4 > Ω6 |
36 |
LYB crystal |
7.67 |
1.45 |
0.82 |
1.77 |
Ω2 >Ω4 > Ω6 |
37 |
Photoluminescence spectra and CIE color coordinates
The PL spectra of the samples in the wavelength range of 450–700 nm with an excitation wavelength of 413 nm (4I15/2–2H9/2 transition) are observed in Fig. 7. For pure CoAl2O4 NCs, the emission spectrum exhibited a peak at 602 nm. This emission peak is attributed to the 2E(2G) → 4A2(4F) transition of tetrahedral Co2+ ions38 and provides clear evidence of the presence of tetrahedral Co2+ ions within the spinel structure of the CoAl2O4 NCs.39 The PL spectra of the Er-doped CoAl2O4 NCs exhibit four distinct emission peaks associated with the transitions of Er3+ ions at wavelengths of 502, 533, 548, and 678 nm. These transitions correspond to the shifts 4F7/2–4I15/2, 2H11/2–4I15/2, 4S3/2–4I15/2, and 4F9/2–4I15/2.15,40 As shown in Fig. 7, the intensity of these emission peaks increased proportionally with the concentration of Er3+. This observation confirms that no fluorescence quenching occurred in the Er-doped CoAl2O4 NCs, even when the Er ion concentration reached 5%.
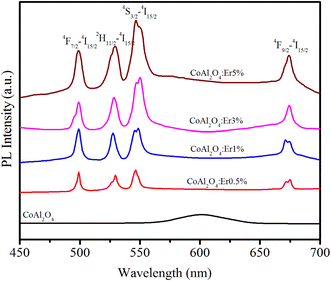 |
| Fig. 7 PL spectra of Er-doped CoAl2O4 nanocrystals with varying Er concentrations. | |
The emission characteristics of a substance are commonly determined using the CIE chromaticity coordinates. Utilizing the emission spectra resulting from the excitation at 413 nm, the color coordinate diagram of the Er-doped CoAl2O4 NCs was estimated and is illustrated in Fig. 8. The corresponding (x, y) coordinates are listed in Table 4. In the case of the pure CoAl2O4 NCs, the luminescence spectrum encompasses emission bands within the red-orange region, as depicted in Fig. 8. For the Er-doped CoAl2O4 NCs, the emission color shifted strongly toward the blue and yellow regions. It can be seen that the emission color of the Er-doped CoAl2O4 NCs changes insignificantly when the Er concentration increases from 0.5–5%. The correlated color temperature (CCT) (K) of a material was determined using the following formula:41
|
CCT = −449n3 + 3525n2 − 6823n + 5520.33
| (18) |
where
n is calculated using the expression:
n = (
x −
xe)/(
y −
ye) with
xe = 0.332 and
ye = 0.186. The calculated CCT values for the samples are listed in
Table 4. These CCT values correspond to neutral white light as perceived by the human visual system.
42
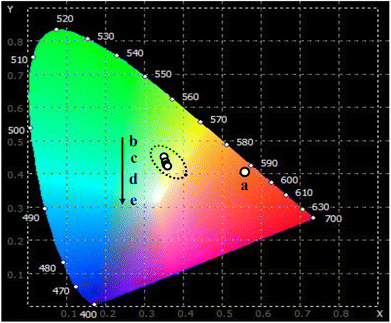 |
| Fig. 8 The CIE color coordinates diagram of CoAl2O4:x%Er3+ NCs: x = 0 (a), 0.5 (b), 1.0 (c), 3.0 (d), and 5.0 (e) with λexc = 413 nm. | |
Table 4 The chromaticity coordinates (x, y) and the correlated color temperature (CCT) for CoAl2O4:x%Er3+ (x = 0–5%) NCs
Sample |
x |
y |
CCT |
CoAl2O4 |
0.560 |
0.400 |
1709 |
CoAl2O4:0.5%Er3+ |
0.358 |
0.463 |
4911 |
CoAl2O4:1.0%Er3+ |
0.360 |
0.423 |
4763 |
CoAl2O4:3.0%Er3+ |
0.365 |
0.422 |
4634 |
CoAl2O4:5.0%Er3+ |
0.361 |
0.420 |
4728 |
Upconversion fluorescence
Up-conversion (UC) emission is a photophysical phenomenon in which a material absorbs two or more lower-energy photons and subsequently emits a single higher-energy photon. The UC luminescence phenomenon has garnered significant attention from numerous researchers due to its multiple promising applications in fields such as bio-imaging, display technology, solar cells, medical diagnostics, and photodynamic therapy. Fig. 9 shows the up-conversion PL spectra of the Er-doped CoAl2O4 NCs (obtained at λexc = 978 nm) in the spectral region of 450–700 nm.
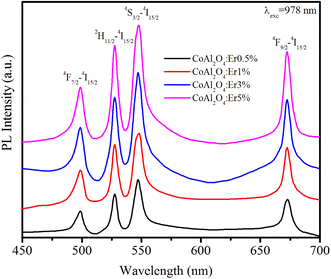 |
| Fig. 9 The up-conversion emission spectra of Er-doped CoAl2O4 nanocrystals with varying Er concentrations, recorded at λexc = 978 nm. | |
The Er3+ ions in the ground state were excited and transitioned to the 4I11/2 state. The energy transfer process occurs between Er3+ ions according to the following equation:
|
4I11/2 + 4I11/2 → 4I15/2 + 4F7/2
| (19) |
This energy transfer process causes the Er3+ ions to transition to the 4F7/2 excited state. The ions in the 4F7/2 state recover to the ground state and emit light from violet to the infrared region.43 The peak positions of all the observed emission bands were almost unchanged compared to the observations in Fig. 7 (λexc = 413 nm). The most intense emission peaks are observed at 533 nm (2H11/2–4I15/2) and 548 nm (4S3/2 → 4I15/2).
The UC phenomenon has been explained by several physical mechanisms such as Auger recombination, two-photon absorption, two-step two-photon absorption, and the thermal excitation of surface states. To gain a deeper understanding of the UC mechanism, we investigated the power-dependent luminescence. Fig. 10 displays the UC emission spectra of Er5%-doped CoAl2O4 NCs when excited at 978 nm with varying excitation power (from 0.05 to 5 mW).
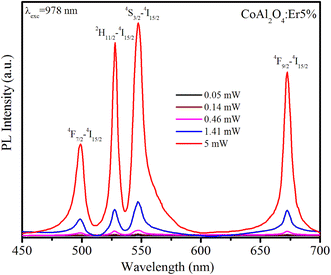 |
| Fig. 10 Upconversion emission spectra of Er5%-doped CoAl2O4 nanocrystals when excited at 978 nm (4I15/2–4I11/2) with varying excitation power from 0.05 to 5 mW. | |
As the excitation power increased, the emission intensity of Er3+ increased. The relationship between the up-conversion luminescence intensity (Iuc) and excitation power (P) can be determined using the following formula:44
where
Iuc is the fluorescence intensity of the Er
3+ ions,
P is the excitation power, and
n is the number of photons required to generate the excited state. The dependence of ln
Iuc on ln
P for these transitions is shown in
Fig. 11. These experimental data can be well-fitted linearly, and the obtained
n values are 1.9, 1.85, 1.93, and 1.77 for
4F
7/2–
4I
15/2,
2H
11/2–
4I
15/2,
4S
3/2–
4I
15/2, and
4F
9/2–
4I
15/2 transitions, respectively. These obtained
n values are approximately equal to 2 indicating that the UC mechanism of these transitions is the result of a two-photon absorption process.
44–46 The two-photon absorption mechanism was also used to explain the upconversion fluorescence phenomenon in Er
3+ and Yb
3+-codoped YBO
3, and Er
3+-doped YbOCl samples.
45 The UC mechanism can be attributed to the saturation phenomenon in which the
n values of the transitions were significantly smaller (0.86–1.58) than the theoretical values.
46
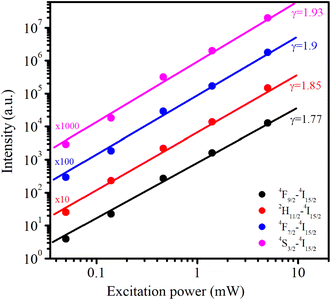 |
| Fig. 11 The dependence of upconversion emission intensity on excitation power (from 0.05 to 5 mW) under 978 nm excitation for Er1%-doped CoAl2O4 nanocrystals. | |
The two-photon absorption mechanism of UC emission is explained by the diagram in Fig. 12. Initially, Er3+ ions absorb photons, transitioning from the ground level 4I15/2 to the 4I11/2 level. Subsequently, the absorption of the second photon raises it from 4I11/2 level to 4F7/2 level. From 4F7/2 state, it recovers to 4I15/2 state by emitting a photon with a wavelength of 502 nm and recovers the multiphonon to the 2H11/2, 4S3/2, and 4F9/2 states. These transitions produce emission peaks at 533, 548, and 678 nm corresponding to 2H11/2–4I15/2, 4S3/2–4I15/2, and 4F9/2–4I15/2 transitions (located in the blue and red light regions). It's worth noting that the 4S3/2 excited level possesses a longer intrinsic lifetime than the 2H11/2 level, increasing the probability of non-radiative transitions. Therefore, the observed results suggest that relaxation from the 4F7/2 state to the 4S3/2 state is the most favored transition.47
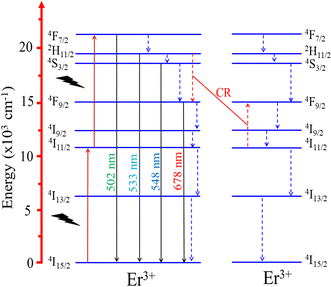 |
| Fig. 12 Energy level diagrams of Er3+, showing possible energy transfer processes in the Er-doped CoAl2O4 NCs. | |
Decay time curves of the 4S3/2–4I15/2 transition (548 nm)
Time-resolved PL measurements provide insight into the decay times of the luminescence process. Fig. 13 shows the decay curves of the 4S3/2–4I15/2 transition (548 nm) for the four samples doped with Er3+, λex = 413 nm. The emission of Er3+ ions in host materials can be attributed to various recombination mechanisms or energy transfer processes. The lifetimes of the 4S3/2–4I15/2 transition of the samples were determined by analyzing the PL decay curve, which was fitted using a bi-exponential function:48 |
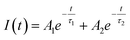 | (21) |
where Ai represents the magnitude and τi denotes the lifetime of the ith component, which is known to be highly influenced by the structure, elemental composition, size, and morphology of the NCs.49 The average lifetime 〈τ〉 is determined based on Ai and τi according to the following equation:50 |
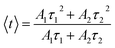 | (22) |
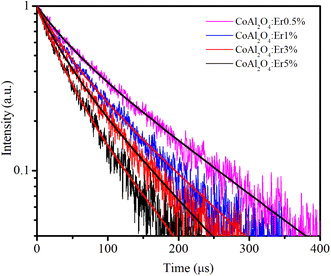 |
| Fig. 13 Decay time curves of Er-doped CoAl2O4 NCs measured at 548 nm (4S3/2–4I15/2), using an EPL-405 in the FLS1000. Measurement parameters: repetition rate = 200 kHz, excitation pulse width = 1 μs, λex = 413 nm, Δλex = 5 nm, resolution = 10 ns per channel. | |
The fitting time constants for the 4S3/2–4I15/2 transition are presented in Table 5.
Table 5 Lifetime decay constants and fitness of curves
Sample |
τ1 (μs) |
A1 |
τ2 (μs) |
A2 |
〈τ〉 (μs) |
R2 |
0.5% |
39.69 |
0.342 |
135.45 |
0.658 |
122.79 |
0.9954 |
1% |
35.53 |
0.416 |
109.83 |
0.584 |
95.92 |
0.9949 |
3% |
32.53 |
0.465 |
95.56 |
0.535 |
81.17 |
0.9948 |
5% |
32.48 |
0.673 |
105.7 |
0.327 |
77.33 |
0.9944 |
A continuous decrease in the average decay lifetime of the 4S3/2–4I15/2 transition at 548 nm was observed as the concentration of Er3+ increased. This finding suggests that the additional decay channels, which accelerate the reduction in the lifetime of the 4S3/2 excited state, become more influential at higher concentrations of Er3+. This phenomenon may be attributed to the heightened impact of nonradiative processes, energy migration among neighboring Er3+ ions, the presence of quenching centers, such as OH- groups in the glass host matrix, and cross-relaxation (CR), as illustrated in Fig. 12 (the red dashed line). The CR process plays a crucial role in efficiently compensating for the loss of excited energy at the 4F9/2 level through nonradiative relaxation, particularly at relatively large concentrations of Er3+.
Conclusion
Er-doped CoAl2O4 NCs with sizes of approximately 30–40 nm were successfully synthesized using the co-precipitation method. The effect of Er doping concentration on the color, structure, and optical properties of the CoAl2O4 NCs was studied. The XRD pattern shows that the CoAl2O4 and Er-doped CoAl2O4 NCs have a spinel structure and do not exhibit any secondary phases. The absorption spectra of the Er-doped CoAl2O4 NCs showed 12 characteristic absorption peaks of Er ions at 365, 383, 413, 441, 452, 497, 520, 543, 651, 797, 978, and 1540 nm, respectively. The Ωλ parameters were calculated and their values decreased with an increase in Er concentration. This suggests that the rigidity and local symmetry of the CoAl2O4 host materials became weaker as the concentration of Er3+ ions increased. The highest value of the Ω2 parameter suggests that the vibrational frequencies of the given samples are relatively low. The PL spectra of the Er-doped CoAl2O4 NCs showed four characteristic emission peaks at 502, 533, 548, and 678 nm originating from the f–f transition in Er3+ ions. Up-conversion PL spectra for Er-doped CoAl2O4 NCs were obtained using an excitation wavelength of 978 nm, with excitation powers ranging from 0.05 to 5 mW. The dependence of Iuc on Pn with n is approximately 2 indicating that the UC mechanism is the result of a two-photon absorption process. The decay time of the 4S3/2–4I15/2 transition decreased as the Er3+ concentration increased. The CR process plays an important role in reducing the energy loss at the 4F9/2 level through non-radiative relaxation, especially at high Er3+ concentrations. The research results obtained show that these Er-doped CoAl2O4 NCs are promising candidates for photonic and color printing applications. The creation of these novel ceramic pigments carries both scientific importance and practical utility.
Conflicts of interest
There are no conflicts to declare.
Acknowledgements
This research is funded by Ministry of Education and Training of Vietnam under grant number B2023-TNA-08. Assoc. Prof. N. T. Binh would like to thank for the support from International Center for Physics of Institute of Physics (project ICP2023.11).
References
- S. K. Pradhan, B. Dalal, A. Sarkar and S. K. De, Phys. Chem. Chem. Phys., 2019, 21, 842 RSC.
- H. Xuanmeng, F. Wang, H. Liu, L. Niu and X. Wang, J. Am. Ceram. Soc., 2018, 101, 2578–2588 CrossRef.
- H. R. Hedayati, et al., Dyes Pigm., 2015, 113, 588–595 CrossRef CAS.
- M. Gaudonn, L. C. Robertson, E. Lataste, M. Duttine, M. Ménétrier and A. Demourgues, Ceram. Int., 2014, 40, 5201–5207 CrossRef.
- J. Chandradassa, M. Balasubramanianb and K. H. Kim, J. Alloys Compd., 2010, 506, 395–399 CrossRef.
- Y. Tong, H. Zhang, S. Wang, Z. Chen and B. Bian, J. Nanomater., 2016, 4169673 Search PubMed.
- Y. Wang, S. Wang, X. Yu, S. Tang, S. Han and L. Yang, Optik, 2020, 210, 164508 CrossRef CAS.
- S. Kanithan, N. A. Vignesh, S. Baskar, S. Nagaraja, M. Abbas, A. Aabid and M. Baig, Materials, 2022, 15, 8180 CrossRef CAS PubMed.
- A. Irshad, et al, Phys. B, 2022, 636, 413873 CrossRef CAS.
- X. Wang, X. Tan, S. Xu, F. Liu, B. A. Goodman and W. Deng, J. Lumin., 2020, 219, 116896 CrossRef CAS.
- S. K. Maurya, R. Kushawaha, S. P. Tiwari, A. Kumar, K. Kumar and J. C. G. E. Da Silva, Mater. Res. Express, 2019, 6, 086211 CrossRef CAS.
- K. Smits, A. Sarakovskis, L. Grigorjeva, D. Millers and J. Grabis, J. Appl. Phys., 2014, 115, 213520 CrossRef.
- D. Wang, B. Xu, K. Zou, M. Sun, G. Dong and J. Liu, Opt. Mater., 2018, 83, 124 CrossRef CAS.
- C. Li, B. Dong, C. Ming and M. Lei, Sens. Basel, 2007, 7, 2652–2659 CrossRef CAS PubMed.
- N. X. Ca, N. T. Hien, X. Fan, P. V. Do, V. H. Yen, P. V. Hao, L. K. Quynh, T. T. T. Huong and V. X. Quang, RSC Adv., 2023, 13, 27292–27302 RSC.
- H. Tan, S. Xie, J. Xu, N. Li, C. Zhang, L. Xu and J. Zheng, Sci. Adv. Mater., 2017, 9, 2223–2233 CrossRef CAS.
- G. S. Yi, H. C. Lu, S. Y. Zhao, G. Yue, W. J. Yang, D. P. Chen and L. H. Guo, Nano Lett., 2004, 4, 2191–2219 CrossRef CAS.
- C. M. Docio, J. J. Reinosa, A. D. Campo and J. F. Fernandez, J. Alloys Compd., 2019, 779, 244–254 CrossRef.
- R. D. Shannon, Acta Crystallogr., Sect. A: Cryst. Phys., Diffr., Theor. Gen. Crystallogr., 1976, 32, 751–767 CrossRef.
- N. X. Ca, N. D. Vinh, S. Bharti, P. M. Tan, N. T. Hien, V. X. Hoa, Y. Peng and P. V. Do, J. Alloys Compd., 2021, 883, 160764 CrossRef CAS.
- R. Jenkins and R. L. Snyder, Diffraction Theory, John Wiley & Sons, Inc, 1996, pp. 47–95, ISBN:9780471513391 Search PubMed.
- X. Peng, J. Cheng, J. Yuan, N. Jin, J. Kang, Y. Hou and Q. Zhang, Adv. Appl. Ceram., 2017, 117, 1–9 Search PubMed.
- X. Duan, M. Pan, F. Yu and D. Yuan, J. Alloys Compd., 2011, 509, 1079–1083 CrossRef CAS.
- T. A. Patterson, J. C. Carver, D. E. Leyden and D. M. Hercules, J. Phys. Chem., 1976, 80, 1702 Search PubMed.
- S. P. Radhika, K. J. Sreeram and B. U. Nair, J. Adv. Ceram., 2012, 1, 301–309 CrossRef CAS.
- C. R. Bamford, Phys. Chem. Glasses, 1962, 3, 189 CAS.
- P. V. Do, N. X. Ca, L. D. Thanh, N. V. Nghia and T. T. C. Thuy, Phys. Chem. Chem. Phys., 2020, 22, 27590 RSC.
- N. M. Khaidukov, V. X. Quang, U. T. D. Thuy, L. D. Thanh, V. P. Tuyen, N. X. Ca and P. V. Do, J. Lumin., 2021, 237, 118201 CrossRef CAS.
- O. Ruiz, F. Sanmiguel, A. Tolosa and N. Alcón, https://www.qualicer.org/recopilatorio/ponencias/pdfs/2012087.pdf.
- R. Reisfeld and C. K. Jorgensen, Lasers and Excited States of Rare Earths, Springer-Verlag, 1977, vol. 1, ISBN978-3-642-66698-8 Search PubMed.
- A. Langar, C. Bouzidi, H. Elhouichet and M. Ferid, J. Lumin., 2014, 148, 249–255 CrossRef CAS.
- I. Jlassi, H. Elhouichet, M. Ferid and C. Barthou, J. Lumin., 2010, 130, 2394–2401 CrossRef CAS.
- A. Racu, M. Stef, G. Buse, I. Nicoara and D. Vizman, Materials, 2021, 14, 4221 CrossRef CAS PubMed.
- X. Zou and T. J. Izumitani, Non-Cryst. Solids, 1993, 162, 68 CrossRef CAS.
- H. Fan, L. Xinran, C. Rongrong, L. Yanzhou, M. Yaohua, R. Maalej and Y. Yanmin, J. Rare Earths, 2017, 35, 964 CrossRef.
- M. Hamzaoui, M. T. Soltani, M. Baazouzi, B. Tioua, Z. G. Ivanova, R. Lebullenger, M. Poulain and J. Zavadil, Phys. Status Solidi B, 2012, 249, 2213–2221 CrossRef CAS.
- Y. W. Zhao, X. H. Gong, Y. J. Chen, L. X. Huang, Y. F. Lin, G. Zhang, Q. G. Tan, Z. D. Luo and Y. D. Huang, Appl. Phys. B, 2007, 88, 51 CrossRef CAS.
- K. Agilandeswari and A. Ruban Kumar, AIP Conf. Proc., 2015, 1665, 120022–120024 CrossRef.
- L. Weizhong, Q. Qiu, F. Wang, S. Wei, B. Liu and Z. Luo, Ultrason. Sonochem., 2010, 17, 793–801 CrossRef PubMed.
- H. Zanane, et al., Opt. Mater., 2020, 100, 109640 CrossRef CAS.
- C. S. McCamy, Color Res. Appl., 1992, 17, 142 CrossRef.
- P. M. Tan, N. X. Ca, N. T. Hien, H. T. Van, P. V. Do, L. D. Thanh, V. H. Yen, V. P. Tuyen, Y. Peng and P. T. Tho, Phys. Chem. Chem. Phys., 2020, 22, 6266 RSC.
- Y. Tang, C. Wu, Y. Song, Y. Zheng and K. Zhao, Ceram. Int., 2018, 44, 12909–12916 CrossRef CAS.
- J. Zhang and F. Qian, Dalton Trans., 2020, 49, 10949–10957 RSC.
- H. Lim, J. Won, S. Wi, S. Jang, J. S. Chung and Y. S. Lee, Solid State Sci., 2021, 117, 106616 CrossRef CAS.
- G. Gorni, J. J. Velazquez, M. Kochanowicz, D. Dorosz, R. Balda, J. Fernandez, A. Duran and M. J. Pascual, RSC Adv., 2019, 9, 31699 RSC.
- E. R. Andresen, G. Bouwmans, S. Monneret and H. Rigneault, Opt. Express, 2013, 21, 20713–20721 CrossRef PubMed.
- T. Fujii, K. Kodaira, O. Kawauchi, N. Tanaka, H. Yamashita and M. Anpo, J. Phys. Chem. B, 1997, 101, 10631 CrossRef CAS.
- N. T. Hien, T. T. K. Chi, N. D. Vinh, H. T. Van, L. D. Thanh, P. V. Do, V. P. Tuyen and N. X. Ca, J. Lumin., 2020, 217, 116822 CrossRef CAS.
- E. I. Madirov, V. A. Konyushkin, A. N. Nakladov, P. P. Fedorov, T. Bergfeldt, D. Busko, I. A. Howard, B. S. Richards, S. V. Kuznetsov and A. J. Turshatov, Mater. Chem. C, 2021, 9, 3493–3503 RSC.
|
This journal is © The Royal Society of Chemistry 2024 |