DOI:
10.1039/D3RA07673C
(Paper)
RSC Adv., 2024,
14, 8671-8683
A prototype of ultrasensitive time-resolved fluoroimmunoassay for the quantitation of lead in plasma using a fluorescence-enhanced europium chelate label for the detection system
Received
9th November 2023
, Accepted 11th March 2024
First published on 14th March 2024
Abstract
This study describes the prototype of a novel ultra-sensitive time-resolved fluoroimmunoassay (TRFIA) for the quantification of lead (Pb) in plasma. The assay procedures were conducted in 96-microwell plates and involved the competitive binding format. The assay used a mouse monoclonal antibody, designated as 2C33, that specifically recognized the diethylenetriamine pentaacetic acid chelate of Pb (Pb-DTPA) but did not recognize Pb-free DTPA chelator. The antigen used for coating onto the inner surfaces of assay plate microwells was Pb-DTPA conjugated with bovine serum albumin protein (Pb-DTPA-BSA). The competitive binding reaction occurred between Pb-DTPA chelates, formed in the sample solutions by treating the samples with an excess DTPA, and the coated Pb-DTPA-BSA for a limited quantity of 2C33 antibody binding sites. The antigen–antibody complex formed in the plate wells was quantified by a europium-DTPA-labeled secondary antibody and a fluorescence enhancement solution. The conditions of the assay were refined, and its optimum procedures were established. The TRFIA was validated following the immunoassay validation guidelines, and all of the validation criteria were acceptable. The working range of the assay was 20–300 pg mL−1 and its limit of quantitation was 20 pg mL−1. Metals that are commonly encountered in blood plasma did not interfere with Pb in the analysis by the proposed TRFIA. The assay was applied to the quantitation of Pb in plasma samples with satisfactory accuracy and precision. The results were compared favorably with those obtained by atomic emission spectroscopy. In conclusion, the present study represents the first TRFIA for the quantitation of Pb in plasma. The assay is superior to the existing atomic spectrometric methods and other immunoassays for Pb in terms of sensitivity, convenience, and analysis throughputs. The proposed TRFIA is anticipated to effectively contribute to assessing Pb concentrations and controlling the exposure of humans to its potential toxicity.
Introduction
Pollution of the environment with toxic heavy metals is a worldwide health concern. Lead has been widely used in different industries including glass, batteries, pipes, cosmetics, toys, and paints.1 It is a persistent ubiquitous toxin in the environment and the consequences of lead contamination are a subject of both ancient interest and great contemporary concern. The WHO has identified lead as one of 10 chemicals of major public health concern needing action by governmental authorities to protect health from lead intoxication.2 Humans are exposed to lead primarily by inhalation of lead-contaminated dust and by ingestion of food, drinking water, settled indoor dust, and soil contaminated with lead. Upon indirect ingestion of lead, it primarily accumulates in the blood, soft tissues, bone, and neurons, and this accumulation may lead to many potential human health risks including anaemia, hypertension, immunotoxicity, disruption of reproductive organs, cognitive obstacles, blindness, encephalopathy, kidney failure, neurological/behavioral changes, and death.3,4
Lead exposure is estimated to account for 21.7 million years lost to disability and death worldwide, due to long-term effects on health. The WHO estimates that 30% of idiopathic intellectual disability, 4.6% of cardiovascular disease, and 3% of chronic kidney diseases can be attributed to exposure to lead.2 Young children are most susceptible and vulnerable to lead intoxication because they engage in hand-to-mouth behavior and may spend long periods in contaminated areas. Additionally, lead absorption occurs more readily in children as compared to adults and children's developing nervous system is more vulnerable to lead than adults. UNICEF estimates that 1 in 3 children (up to 800 million globally) have blood lead levels at or above 5 μg dL−1, and immediate global action is needed to address this problem.5 Most cases of lead poisoning in children with moderately elevated lead levels are asymptomatic resulting in more subtle effects such as deficits in neurobehavioral cognitive performance, attention span, and impaired learning ability. These deficits have been associated with blood lead levels as low as 10 μg dL−1.2 As a direct result, in October 2023, the Centers for Disease Control and Prevention (CDC) redefined childhood lead poisoning and recommended the use of a blood leads reference value of 3.5 μg dL−1 to identify children with blood lead level higher than most children's levels. For lead levels higher than this value, healthcare providers can use CDC's recommended actions based on blood lead level to develop a plan of action for their patients.6 Early research has focused on indirect measurement of exposure by analyzing erythrocyte protoporphyrin level as a primary screening test for the detection of lead intoxication. However, erythrocyte protoporphyrin level does not reliably identify children with blood lead levels less than 10 μg dL−1.7 Therefore, the CDC recommended that a direct assay of blood lead concentrations be used rather than indirect measurements. In addition, part of CDC plan to seek the development of lead-testing technology for assays with high sensitivity to detect low concentrations of lead in plasma. These recommendations have imposed additional technical and analytical challenges and have led to a greatly increased demand for novel, rapid analytical techniques that can be inexpensive enough to use in large-scale public health screening programs.8,9
The methods used to detect lead included graphite furnace atomic absorption spectrometry,10,11 atomic fluorescence spectrometry,12,13 and inductively coupled plasma mass spectrometry.14 These spectrometric methods offer high sensitivity and accuracy; however, they involve tedious and time-consuming sample pretreatment, use expensive and complex equipment, and need highly skilled personnel, making them impractical. Recently, several electrochemical sensors have been developed for lead ion detection.15–17 However, these sensors also face limitations due to the need for specialized materials and biological molecules, intricate design, lengthy sample preparation, and insufficient specificity. Because of these factors, more work is required to develop an alternative practical accurate, rapid, and inexpensive method to measure lead levels in blood plasma.
Immunoassays have more recently been considered for the analysis of environmental contaminants.18,19 Immunoassays are speedy, sensitive, selective, and cost-effective. In addition, they are well suited for the analysis of large numbers of samples and often obviate lengthy sample preparations. Because of these characteristics, the Human Exposure Branch of the Environmental Protection Agency (EPA) initiated a program to develop and evaluate new immunoassay methods for screening and quantitative analysis of compounds of environmental concern.20 Most environmental immunoassays are directed toward organic compounds;18,19 however, this technique applied to any analyte, including metals, if a suitable antibody can be generated.20–23
Time-resolved fluoroimmunoassay (TRFIA) is a type of immunoassay that has been developed in recent decades.24 In these assays, chelates of rare-earth lanthanide elements such as Europium and samarium are used as labels because of their intrinsic high fluorescence intensity, ability to overcome high-background interferences, and delayed relaxation time. TRFIA has been used for the detection of different molecules; however, the technique has not been described yet for lead.
To the best of our knowledge, there is no TRFIA in the available literature for measuring lead in blood plasma. The present study describes the development of a novel prototype fluorescence-enhanced TRFIA for quantitation of low concentrations of lead in plasma samples. This TRFIA used a monoclonal antibody (2C33) that recognizes the lead chelate of diethylenetriaminepentaacetic acid (Pb-DTPA) and does not recognize Pb-free DTPA chelator. The study involved two stages; the first one was the optimization of experimental operational conditions including temperature, concentration, time, and the system used for the generation and enhancement of fluorescence signals. The second stage is the validation of TRFIA in terms of its sensitivity, accuracy, precision, specificity, and application validity of the assay to plasma samples. This prototype TRFIA was able to reliably measure blood lead levels at as low concentrations as 20 pg mL−1. The ultrasensitive TRFIA established in this study is a very valuable tool for screening elevated blood lead levels. It also provides new research ideas and technical means for the ultra-sensitive detection of other small molecules and will provide the necessary way for the subsequent development of a TRFIA kit with independent property rights.
Experimental
Instruments
The SpectraMax® M5 microplate reader Molecular Devices, LLC, (San Jose, California, USA) was used. It has multi-detection modes (absorbance, fluorescence, fluorescence polarization, time-resolved fluorescence, and chemiluminescence). The reader can read lights in the range of 200–1000 nm with 1 nm increments. The reader can adapt microplates ranging from standard 6-well to 384-well, and its 4-zone system can control temperatures up to 50 °C, allowing temperature-sensitive assays to be performed with excellent stability. The instrument also featured an internal shaker with three different speeds (low, medium, and high) for mixing solutions. The reader is controlled and operated by the SoftMax® Pro Enterprise software, provided with the reader. ELx50 automatic microplate strip washer was a product of Bio-Tek Instruments Inc. (Winooski, USA). An incubator (model MINI/18) was obtained from Genlab Ltd. (Widnes, UK). Milli-Q water purification system (Labo, Millipore Ltd., Bedford, USA). Microprocessor laboratory pH meter (Mettler-Toledo International Inc., Zürich, Switzerland). Vortex (Clifton cyclone CM1: Weston, England). Biomedical freezer (Sanyo MDF-U5312: Onoda, Japan).
Materials
Standard metal solutions (1 mg mL−1, in 2% HNO3) of atomic absorption spectroscopy grade were obtained from PerkinElmer Co. (Norwalk, CT, USA). Diethylenetriamine pentaacetic acid (DTPA) was obtained from Sigma-Aldrich Chemical Co. (St. Louis, MO, USA). [(R)-2-Amino-3-(4-isothiocyanatophenyl)propyl]-trans-(S,S)-cyclohexane-1,2-diaminepenta-acetic acid (p-SCN-Bn-CHX-A′′-DTPA) was purchased from Dojindo Laboratories (Gaithersburg, MD, USA). Ultrapure bovine serum albumin (BSA) was bought from Boehringer Mannheim Biochemicals (Indianapolis, IN, USA). 2C33 monoclonal antibody recognizing Pb-DTPA was generated by a fusion of spleen cells of an immunized BALB/c mouse with SP2/0-Ag14 myeloma cells. The antigen used for immunization was a keyhole limpet hemocyanin protein conjugated with Pb-DTPA (Pb-DTPA-KLH). The IC50 of 2C33 antibody was 5 ng mL−1, as determined by ELISA. The antibody was highly specific for Pb-DTPA chelate among many other metal-DTPA chelates. The details for the generation and characterization of 2C33 antibody have been submitted for publication elsewhere. White opaque high-binding 96-microwell plates with flat bottoms were a product of Corning/Costar, Inc. (Cambridge, MA, USA). Goat anti-mouse IgG was purchased from Sigma-Aldrich Chemical Co. (St. Louis, MO, USA). Pierce™ BCA protein assay kit was purchased from ThermoFisher Scientific Inc. (Waltham, MA USA) and used according to the manufacturer's directions. Centricon-30 filter was from Amicon, Inc. (Beverly, MA, USA). Thenoyltrifluoroacetone (TTA), tri-n-octylphosphine oxide (TOPO), and Triton X-100 surfactant were obtained from Sigma-Aldrich Chemical Co. (St. Louis, MO, USA). Human plasma samples were obtained from King Khalid University Hospital (Riyadh, Kingdom of Saudi Arabia), and were kept frozen at −20 °C until used in the analysis. Metal-free disposable pipette tips were bought from Oxford Labware, Inc. (St. Louis, MO, USA). All glassware was washed with mixed HCl/HNO3 acids and liberally rinsed with purified deionized water. All plastic wares were soaked overnight in 3 M HCl and rinsed with purified deionized water before use.
Preparation of Pb-DTPA-BSA conjugate
The conjugate of BSA protein with Pb-DTPA (Pb-DTPA-BSA) was prepared by a modification of the method previously reported.22 Briefly, a solution of p-SCN-Bn-CHX-A′′-DTPA (3 mM, in 0.1 M sodium phosphate buffer, pH 9.5) was prepared. Aliquot (1 mL) of p-SCN-Bn-CHX-A′′-DTPA solution was mixed with an equal volume of lead nitrate solution (3 mM, in 50 mM phosphate buffer, pH 9.5). BSA solution (2 mL, 20 mg mL−1, in 0.1 M sodium phosphate buffer, pH 9.5) was added. The pH of the reaction mixture was maintained at pH 9.5 for 30 min by the addition of KOH when necessary. The reactions were stirred overnight at 25 °C. The unreacted low molecular weight components (Pb and p-SCN-Bn-CHX-A′′-DTPA) were removed by buffer exchange using a Centricon-30 filter that had been treated with 100 mM DTPA solution and liberally rinsed with water before use. Protein concentrations of the conjugates were determined using BCA kit,25 and the extent of substitution of free amino groups on the protein was determined by estimation of free amino groups before and after the conjugation reaction.26
Preparation of europium-labeled IgG
The europium-labeled secondary antibody (Eu-IgG) was prepared according to the procedures described in a previous report.27 Briefly, 10 mg of goat anti-mouse IgG was dissolved in 1 mL phosphate buffer saline solution (PBS: 50 mmol L−1, pH 7.4). A solution of Eu and p-SCN-Bn-CHX-A′′-DTPA was prepared by mixing equal volumes of equimolar solutions (3 mM) of Eu3+ and p-SCN-Bn-CHX-A′′-DTPA; the solutions were prepared in phosphate buffer saline solution (PBS: 50 mmol L−1, pH 7.5). Aliquot (1 mL) of Eu- p-SCN-Bn-CHX-A′′-DTPA solution was mixed with an equal volume of IgG solution, and then the solution was stirred by magnetic agitation in darkness for 24 h at 4 °C. The reaction solution was purified from unreacted Eu chelate of p-SCN-Bn-CHX-A′′-DTPA by Centricon-30 filter using PBS solution and centrifugation. The purified concentration of Eu-IgG, as protein, was determined by BCA protein assay kit. To the purified solution of Eu-IgG, an equal volume of glycerol and BSA (2%, w/v, in PBS), and then the solution was stored at −20 °C for use in the analysis.
Pretreatment of plasma samples
Aliquot (100 μL) of plasma samples were mixed (1
:
1) with 1 M HNO3 and allowed to react for 5 min at 25 °C. The mixtures were subjected to ultrafiltration using a 3 kDa cut-off filter (Centricon-30). During centrifugation (10 min, 600 rpm, 4 °C) the filters completely retained the plasma, but the displaced metals and other small molecules passed through the filter membrane. The filtrate solutions were subsequently neutralized with KOH and their lead concentrations were determined by TRFIA.
Procedure of TRFIA
Microwell plates were coated with Pb-DTPA-BSA conjugate (1 μg mL−1, in PBS) for 2 h at 37 and blocked with 100 μL of BSA (2%, w/v, in PBS) for 0.5 h at 37 °C. The detailed procedures of coating and blocking were described in a previous report.28 Plasma samples were mixed with 10% volume of PBS containing DTPA (5 mM), and aliquots (50 μL) of the diluted samples were mixed (1
:
1) with 2C33 monoclonal antibody solution (0.5 μg mL−1 in PBS). From this mixture, 50 μL was transferred to each well of the assay plates, and the plates were incubated for 1 h at 37 °C. The plates were washed after the incubation, the plates were washed three times with PBS containing Tween-20 (0.05%, v/v). After washing, 50 μL of Eu-IgG solution (0.5 μg mL−1) was added to each well. The binding of Eu-IgG to the assay plate wells was allowed to proceed for 0.5 h at 37 °C. The plate wells were washed and 100 μL of fluorescence enhancement solution (FES) was added. The FES was prepared in acetate buffer (0.1 M, pH 3.2) and it consisted of TTA (2 mM), TOPO (0.1 mM), and Triton X-100 (0.2%, v/v). The plate was holed and shaken by the reader shaking system for 3 min, and then the fluorescence intensities were measured by the reader at 355 and 620 nm for excitation and emission, the data was transformed to Slide Write software, version 5.011 (Advanced Graphics Software, Inc., Rancho Santa Fe, CA, USA) for fitting by the 4-parameter equation. Standard curves for Pb(II) were obtained using the same procedure on plates of the same series. Values for IC50 were calculated using the following equation:
F = F0 + {(F0 − F1)/{1 + [Pb)]/(IC50)} |
where F is the fluorescence at a definite known concentration of Pb(II), F0 is the fluorescence in the absence of Pb, F1 is the fluorescence at the saturating concentration of Pb, and IC50 is the Pb concentration that produces a 50% inhibition of maximum fluorescence signal (F0). The concentrations of Pb in the samples were then computed by the fitting equation.
Results and discussion
Description of TRFIA
This study describes the development of ultra-sensitive TRFIA for trace determination of Pb in plasma samples. The features and steps of this TRFIA are illustrated in Fig. 1. The assay involved 4 main steps (A–D). The first step (A) involved the transformation of Pb in the sample solution to its DTPA chelates, the form recognized by the 2C33 antibody. This transformation was performed by dilution of the sample with a buffer solution containing a molar excess of metal-free DTPA. Principally, an excess of DTPA should be added to ensure the transformation of all possibly present Pb to the chelate form. The second step (B) was the competitive binding reaction between the free soluble Pb-DTPA chelates with the immobilized Pb-DTPA chelate (in the form of BSA conjugate coated on the assay wells) for a limited amount of 2C33 antibody binding sites. The third step (C) involved the binding of Eu-labeled anti-mouse IgG (Eu-IgG) to 2C33 antibody that has been bound to the immobilized Pb-DTPA-BSA conjugate. The fourth step (D) involved the development of enhanced fluorescence signals by the addition of the fluorescence enhancement solution (FES) and the formation of the fluorescent product. The fluorescence signals were measured and plotted as a function of the corresponding Pb concentrations in the original sample solution. The 4-parameter equation fit of the data was used for calculating the Pb concentrations in the sample solutions.
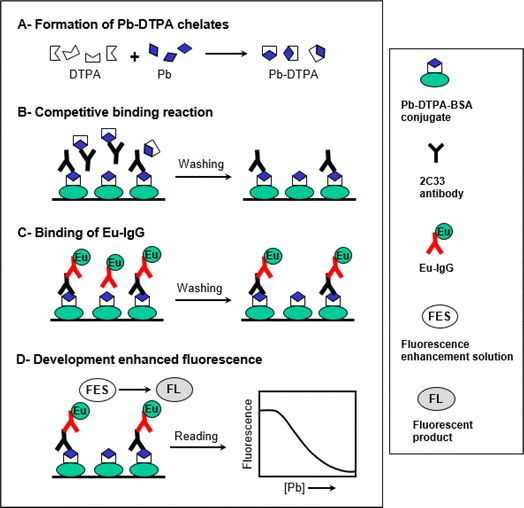 |
| Fig. 1 A schematic diagram for the proposed TRFIA for Pb. | |
Characterization of Pb-DTPA-BSA conjugate
The preparation of Pb-DTPA-BSA conjugate is illustrated in Fig. 2. The bifunctional chelator, p-SCN-Bn-CHX-A′′-DTPA, was first used to form Pb-DTPA chelate via its pentaacetic acetic acid groups. This chelate was coupled via the reaction of the isothiocyanate group of p-SCN-Bn-CHX-A′′-DTPA with the amino group residues on BSA protein. The p-SCN-Bn-CHX-A′′-DTPA is a modified derivative of DTPA that incorporates an isothiocyanate, benzyl, and cyclohexyl groups. While both p-SCN-Bn-CHX-A′′-DTPA and DTPA are capable of forming chelates with metal ions via their pentaacetic acetic acid groups, p-SCN-Bn-CHX-A′′-DTPA offers the additional functionality and reactivity provided by the benzyl isothiocyanate group through which a protein molecule can be linked producing DTPA-protein conjugate. The use of DTPA instead would lead to chelating Pb and forming Pb-DTPA chelates; however, the chelate could not be linked to BSA protein. To ascertain the formation of the Pb-DTPA-BSA conjugate, a colorimetric reaction involving amino group residues of BSA was conducted on a definite concentration of the conjugate and the same concentration of unconjugated BSA protein. The reaction was carried out as described in a previous report.26 The relative decrease in the absorbance (%) was attributed to the substitution of amino groups in forming the conjugate. The degree of conjugation of BSA with Pb-DTPA was found to be 36.4%. It is wise to mention that this degree of conjugation was very reasonable for the use of the conjugate as a capturing agent in the assay. This assumption was supported by a previous study that demonstrated the successful use of protein conjugate at a lower extent (20.6%).21
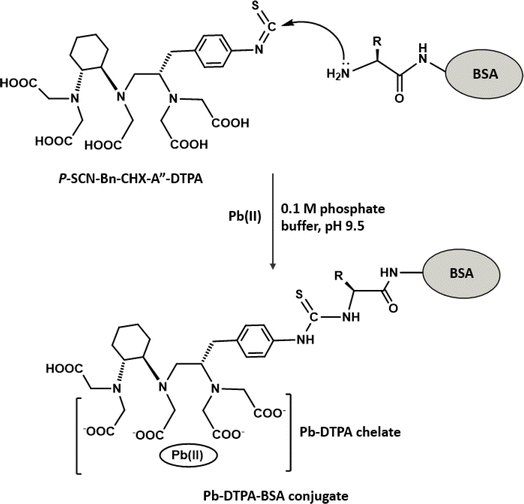 |
| Fig. 2 The preparation of Pb-DTPA-BSA conjugate. P-SCN-Bn-CHX-A′′-DTPA is [(R)-2-amino-3-(4-isothiocyanatophenyl)propyl]-trans-(S,S)-cyclohexane-1,2-diaminepentaacetic acid. BSA is bovine serum albumin. | |
Preparation and characterization of Eu-IgG
The labelling of the second anti-mouse IgG was performed by following the procedures used for the preparation of Pb-DTPA-BSA conjugate; however, IgG was used instead of BSA. The Eu-labelled IgG molecules were purified from the small remaining unreacted components (Eu-DTPA chelates) by passing through a membrane filter that passed the small components and retained the Eu-IgG molecules. After, the successful preparation of Eu-IgG, the fluorescence spectra (excitation and emission) of its solution were recorded by the SpectraMax M5 multifunctional microplate reader to determine the optimal excitation and emission wavelengths for measuring in the proposed TRFIA. The spectra revealed that the maximum excitation and emission wavelengths were 355 and 620 nm, respectively, and the detection delay time was 400 μs.
Optimization of TRFIA conditions
Selection of Pb-DTPA-BSA and 2C33 concentrations. In the proposed TRFIA (Fig. 1), Pb was quantified by its ability to competitively inhibit the binding of 2C33 antibody to the immobilized Pb-DTPA-BSA conjugates. Accordingly, the amount of 2C33 antibody should be limited relative to the particular amount of the immobilized Pb-DTPA-BSA conjugate to achieve an effective competition and ultimately develop a highly sensitive assay. To determine the best pair of concentrations of Pb-DTPA-BSA conjugate and 2C33 antibody, a checkerboard titration was carried out. A series of non-competitive reactions were performed using varying concentrations of Pb-DTPA-BSA conjugate and 2C33 antibody. The results of checkerboard titration revealed that Pb-DTPA-BSA conjugate at concentrations of ≤1 μg mL−1 was limited at all the tested concentrations of 2C33 antibody (Fig. 3A). To select the most appropriate concentrations from these concentrations, a separate set of competitive experiments was conducted, and the IC50 value was determined in each case. The obtained IC50 values were plotted as a function of both Pb-DTAP-BSA and 2C33 antibody (Fig. 3B). It was generally noticed that the IC50 decreases as the 2C33 antibody concentration decreases at a fixed concentration of Pb-DTPA-BSA conjugate. Likewise, IC50 decreases as the concentration of Pb-DTPA-BSA conjugate decreases at a fixed concentration of 2C33 antibody. The smallest IC50 values (50 ± 5 pg mL−1) were achieved when the concentrations of Pb-DTPA-BSA conjugate were ≤1 μg mL−1, and those of 2C33 antibody were 0.25 and 0.5 μg mL−1 (Fig. 3B). The IC50 values obtained when the 2C33 antibody was 0.25 μg mL−1 were not reproducible. Therefore, the subsequent experiments were conducted using conjugate and antibody at a concentration of 0.5 μg mL−1 versus a Pb-DTPA-BSA conjugate at a concentration if 1 μg mL−1.
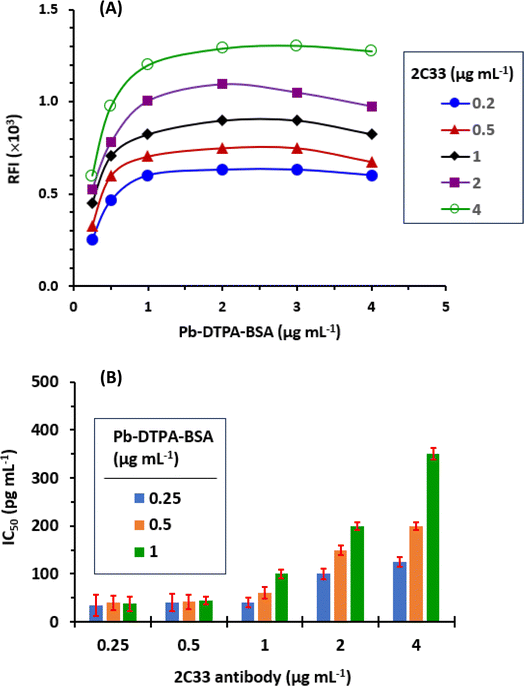 |
| Fig. 3 The checkerboard titration of varying concentrations of Pb-DTPA-BSA conjugate versus different concentrations of 2C33 antibody (A) and the relation of IC50 values with the concentration of conjugate and antibody (B). | |
Coating of Pb-DTPA-BSA and blocking the plate wells. To establish the best incubation time and temperature required for the coating of Pb-DTPA-BSA onto the wells of the assay plates, 50 μL of Pb-DTPA-BSA solution (1 μg mL−1) was dispensed into each well of the assay plate. The plates were incubated under different conditions: overnight (defined as 8 h) at 4 °C (in the refrigerator), at room temperature (25 °C) for 3 h, and at 37 °C (in a thermostatically controlled incubator) for 3 h, and then the plates were manipulated as usual. The results of this study are presented in Fig. 4A. These results indicated that the incubation for overnight at 4 °C gives incomplete coating when compared with the incubations at room temperature (25 °C) for 3 h and at 37 °C. The absorbance value obtained when coating was conducted for overnight at 4 °C was 0.3 ± 0.05 compared with 0.5 ± 0.07 and 0.6 ± 0.09 when the coating was conducted at 25 °C and at 37 °C for 3 h, respectively. Therefore, the coating in the refrigerator at 4 °C was ruled out from further study. The effect of coating time at room temperature (25 °C) and 37° was investigated by coating at these temperatures for varying periods. The results of these experiments are presented in Fig. 4B, from which it was found that the optimum coating of Pb-DTPA-BSA was achieved when the plate was incubated for 2 h at 37 °C.
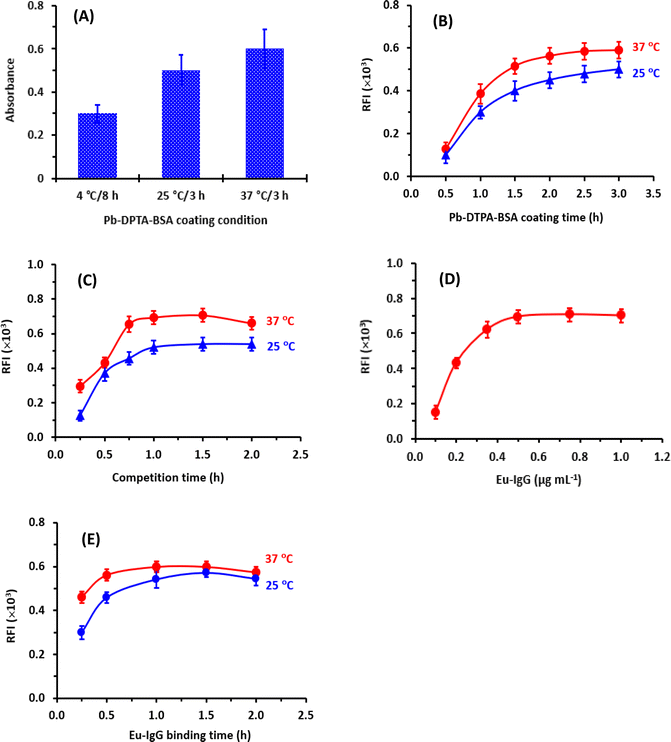 |
| Fig. 4 The results of studying of coating time and temperature of Pb-DTPA-BSA conjugate on the assay plates (A), the time required for optimum coating of Pb-DTPA-BSA at 25 °C and 37 °C (B), the time of competitive binding reaction between the coated Pb-DTPA-BSA and free Pb-DTPA (in sample solution) with 2C33 antibody (C), concentration of Eu-IgG (D), and time of binding of Eu-IgG at 25 °C and at 37 °C (E). | |
After coating Pb-DTPA-BSA onto the assay plate wells, the remaining protein-binding sites remaining available on the surface of the wells should be blocked with high protein concentration. In a previous study,21 BSA at a concentration of 2% (w/v) was proved as an efficient blocking agent; therefore, it was selected, and the same blocking conditions were employed in the present study. These conditions were 100 μL of BSA solution 2% (w/v) and the incubation was carried out for 0.5 h at 37 °C.
Competitive binding reaction. The results obtained from studying the proper time for competitive binding reaction between Pb-DTPA, in the sample solution, and 2C33 antibody revealed that the effective competition needs 1 h at 37 °C to reach equilibrium (Fig. 4C). At these conditions, constant and reproducible IC50 value was obtained. Longer time did not decrease the IC50 value (i.e., did not enhance the assay sensitivity).
Concentration and binding of Eu-IgG. The best concentration of Eu-IgG was studied, and it was found to be 0.5 μg mL−1 (Fig. 4D). The most appropriate conditions (time and temperature) for its binding were investigated and the optimum binding was achieved for 0.5 at 37 °C (Fig. 4E).
Fluorescence enhancement conditions. Lanthanide chelates of DTPA are extremely stable, and we used Eu-DTPA derivatives conjugated to 2C33 antibody as a tracer in the present TRFIA. For enhancement of fluorescence in TRFIA, different fluorescence the enhancement solutions (FES) were proposed.29 The most recommended solutions based on a β-diketone (thenoyltrifluoroacetone, TTA) in a buffer solution containing a water-protecting agent (tri-n-octylphosphine oxide, TOPO), and a detergent (Triton X-100). The concentrations of these components were studied, and the results are given in Fig. 5. The best concentrations of TTA, TOPO, and Triton X-100 were 2 mM, 0.1 mM, and 0.2% (v/v), respectively (Fig. 5A–C, respectively). The effect of the pH of the FES solution on the induced fluorescence intensity was investigated in the range of 2–8, and it was found that the maximum signals were obtained when the pH values were between 4 and 5 (Fig. 5D), and the pH of 4.5 was used for the subsequent experiments.
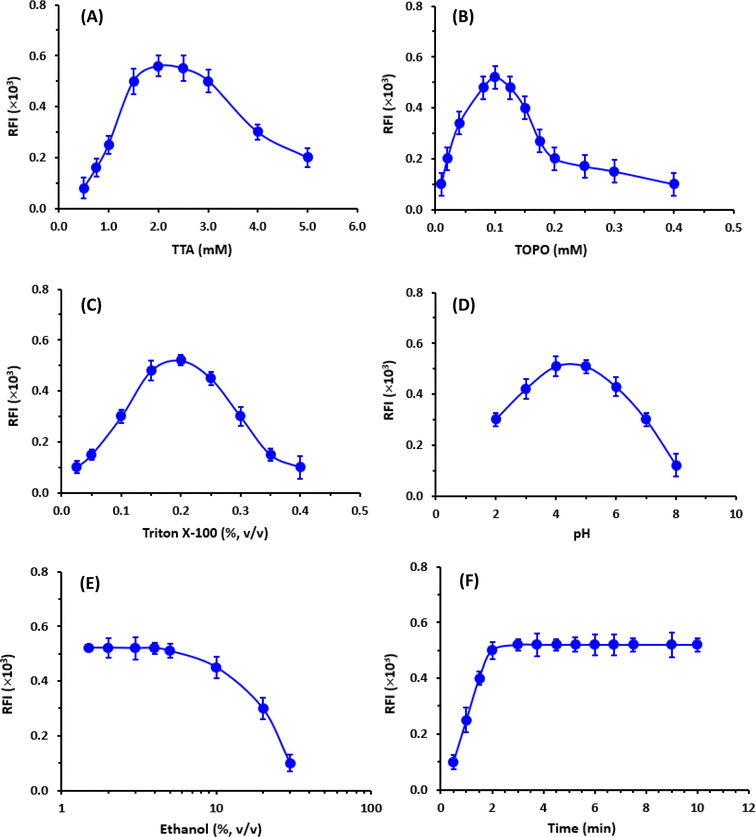 |
| Fig. 5 The effects of different variables on the performance of fluorescence enhancement solution (FES). These conditions were the TTA concentration (A), TOPO concentration (B), Triton X-100 concentration (C), pH of solution (D), ethanol concentration in the solution (E), and the fluorescence development time (F). | |
The enhancers (TTA and TOPO) must first be pre-dissolved in an organic solvent (ethanol or dimethylformamide) because they are typically difficult to dissolve in aqueous buffer systems. Ethanol was recommended for dissolving these enhancers before the dilutions of their solutions to the optimum concentrations in a buffer solution.29 Hence, the influence of ethanol concentration in the EFS on the fluorescence intensity was tested in the range of 1.5 to 30% (v/v). It was found that the presence of ethanol in the EFS (up to 5%) had no adverse effect on the fluorescence intensity (Fig. 5E).
To establish the optimum time for induction of the highest fluorescence intensity, the fluorescence development was monitored at room temperature for 10 min. The optimum time at which the highest fluorescence intensity was obtained was 2 min and remained stable for the further time up to 10 min (Fig. 5F). For obtaining reading with better precision, measurements in the subsequent experiments were taken after 3 min.
A summary of the variables affecting the TRFIA are summarized in Table 1.
Table 1 Summary for optimum parameters and conditions of TRFIA for Pb in plasma
Parameter/condition |
Optimum value |
FES: the fluorescence enhancement solution was prepared in acetate buffer (0.1 M, pH 4.5) and it consisted of TTA (2 mM), TOPO (0.1 mM) and Triton X-100 (0.2%, v/v). |
Coating Pb-DTPA-BSA conc. (μg mL−1) |
1 |
Coating time (h)/temperature (°C) |
2/37 |
BSA concentration for blocking (%, w/v) |
2 |
Blocking with BSA: time (min)/temperature (°C) |
0.5/37 |
Concentration of DTPA (mM) |
5 |
2C33 antibody concentration (μg mL−1) |
0.5 |
Eu-IgG concentration (μg mL−1) |
0.5 |
Binding of Eu-IgG: time (h)/temperature (°C) |
0.5/37 |
FES: time (min)/temperature (°C)a |
3/25 |
Measuring wavelength (excitation/emission, nm) |
355/620 |
Validation of TRFIA
The performance of TRFIA was assessed by its validation following the guidelines of the validation of immunoassays for bioanalysis.30
Calibration curve and sensitivity. The calibration curve was generated using atomic absorption grade Pb at a concentration range of 1–5000 pg mL−1 (Fig. 6A). The working range of the assay at Pb concentration giving 90–10% of the maximum binding antibody was 20–300 ng mL−1. The limits of detections (LOD) and quantitation (LOQ), defined as the lowest concentration of Pb that could be distinguishable from zero concentration ± 3.3 and 10 SD, respectively, were determined according to the guidelines of the immunoassay validation.30,31 Based on the triplicate measurements, the LOD and LOQ were 6.6 and 20 pg mL−1, respectively. This achieved LOQ value is much lower than the maximum allowable concentrations of Pb in plasma (35 ng mL−1) which is recommended by the CDC.7
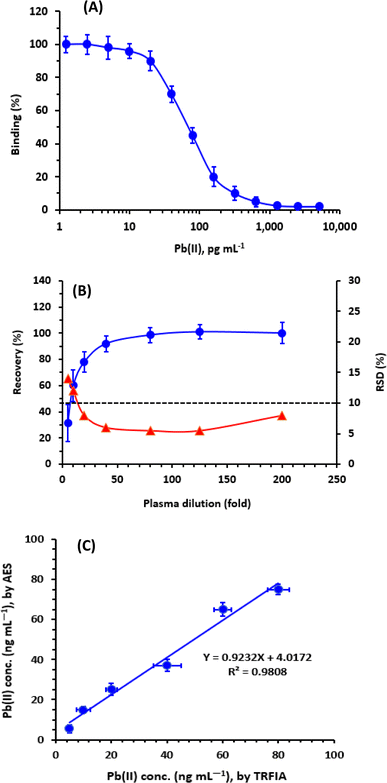 |
| Fig. 6 The binding calibration curve of TRFIA for measurement of lead, as Pb(II), in plasma (A), the effect of plasma matrix (B); the results are presented as recovery (●) and RSD (▲), and the correlation of the measured concentrations by TRFIA with those by atomic emission spectrometry, AES (C). | |
Selectivity of TRFIA. The selectivity of 2C33 antibody for measurement of Pb, as its DTPA chelate, was proved during the selection of hybridoma clone producing the 2C33 antibody. While the selectivity of any immunoassay is also influenced by the procedures variables such as the concentrations of coated antigen and antibody. Therefore, it was essential to re-evaluate the selectivity of the proposed TRFIA under its optimized conditions for the measurement of Pb among the other metals commonly encountered in plasma. A set of competitive assays was conducted by using different metals (given in Table 2) over a wide range of concentrations, and the IC50 values were determined for all. The cross-reactivity of each metal was calculated using the formula:
Cross reactivity (%) = IC50 [Pb]/IC50 [metal] × 100 |
Table 2 The selectivity of TRFIA for measurement of Pb in plasma
Metal |
IC50a (pg mL−1) |
Cross reactivityb (%) |
IC50 is the concentration of metal-DTPA complex which inhibits 50% of the maximum RFI in the TRFIA. Calculated as IC50 [Pb]/IC50 [metal] × 100. Values are the mean of triplicate determinations. Not determined because it was more than 1000 pg mL−1. |
Lead, Pb |
47c |
100c |
Zinc |
750 |
6.2 |
Nickel |
1850 |
2.5 |
Mercury |
4500 |
1.0 |
Cadmium |
NDd |
ND |
Manganese |
ND |
ND |
Magnesium |
ND |
ND |
Iron |
ND |
ND |
Calcium |
ND |
ND |
Copper |
ND |
ND |
Chromium |
ND |
ND |
Selenium |
ND |
ND |
The obtained IC50 values and cross-reactivities of all the tested metals are given in Table 2. The values indicated that the proposed TRFIA was highly selective for Pb because the highest observed cross reactivity was 6.2% for zinc. The presence of other metals, even those present in high concentrations in plasma such as calcium, iron, copper, chromium, and selenium31 did not interfere with Pb in the present TRFIA.
It is wise to state that the favored affinity of the 2C33 antibody to Pb-DTPA chelate among the chelates of other metals was attributed to the following facts. Previous studies proved the ability of monoclonal antibodies to bind to metal chelates, even of the same chelator, with high affinity and specificity.32 The high specificity of monoclonal antibodies towards specific metal chelates is attributed to their unique three-dimensional structures and the specific interactions they form with the chelates. It is widely understood that when a chelator binds to a metal, it creates a chelate by coordinating with the metal through its functional chelating groups. This chelate can exhibit a distinct geometrical structure and arrangement of atoms, even with the same chelator. These structural variances can influence the binding interactions between the metal chelate and the monoclonal antibody. Typically, monoclonal antibodies recognize their targets including metal chelates via a combination of electrostatic interactions, hydrogen bonding, van der Waals forces, and hydrophobic interactions. These interactions dictate the antibody's binding affinity and selectivity. As a result, even minor structural differences in the metal chelate, such as variations in coordination geometry or the presence of additional ligands, can lead to different recognition patterns by the monoclonal antibody. Moreover, the differences in affinities of antibodies towards metal chelates are influenced by the varying affinities of metals towards specific amino acid side chains that contain atoms such as sulfur, nitrogen, and oxygen. Antibodies that possess these amino acids in their complementary regions are likely to exhibit distinct abilities in binding to metal chelates. Conclusively, the differential recognition of different metal chelates, derived from the same chelator, by monoclonal antibodies is primarily attributed to the variations in three-dimensional structures and interactions between the metal ion complexes and the binding site of the antibody.32
Accuracy and precision. The accuracy of the proposed TRFIA was assessed by recovery studies. Known amounts of atomic absorption grade Pb were spiked into plasma samples. Each sample was subsequently treated and analyzed for Pb content by TRFIA, as described in the Experimental section. The measured concentrations were used for calculating the recovery using the formula:
Recovery (%) = measured Pb concentration/spiked Pb concentration × 100. |
The recovery values ranged from 95.8% to 104.2% (Table 2), indicating the high accuracy of the TRFIA.
The precisions (intra- and inter-assay) were determined at three different levels of Pb concentrations, low medium, and high (Table 3). The TRFIA gave satisfactory results over all the tested levels of Pb concentration; the coefficients of variations (CV) did not exceed 6.5% (Table 3).
Table 3 The accuracy and precision of the proposed TRFIA for Pb at different concentration levels
Taken Pb conc. (pg mL−1) |
Intra-assay |
Inter-assay |
Measured Pb conc. (pg mL−1 ± SD) |
Recovery (% ± CV) |
Measured Pb conc. (pg mL−1 ± SD) |
Recovery (% ± CV) |
Values are coefficient of variation (%); mean of 3 determinations. |
25 |
25.4 ± 1.4 |
101.5 ± 5.5a |
25.6 ± 1.6 |
102.4 ± 6.4 |
75 |
72.9 ± 2.4 |
97.2 ± 3.2 |
73.95 ± 3.6 |
98.6 ± 4.8 |
150 |
156.3 ± 9.2 |
104.2 ± 6.1 |
143.7 ± 9.8 |
95.8 ± 6.5 |
A summary of the validation parameters of the proposed TRFIA is given in Table 4.
Table 4 Summary for analytical performances of TRFIA for measurement of Pb in plasma
Parameter |
Value |
Working range (pg mL−1) |
20–300 |
Correlation coefficient (r) |
0.9986 |
LOD (pg mL−1) |
6.6 |
LOQ (pg mL−1) |
20 |
Accuracy (recovery, %) |
95.8–104.5 |
Intra-assay precision (CV, %) |
3.2–6.1 |
Inter-assay precision (CV, %) |
4.8–6.5 |
Effect of plasma matrix and assay applicability
The effect of plasma matrix on the reliability of the TRFIA for quantifying Pb in plasma samples was assessed. For conducting the experiments, blank plasma samples were spiked with Pb at a concentration of 10 μg dL−1 (100 ng mL−1). These samples were then treated and diluted as described above; the dilution folds ranged from 5 to 200-folds. The analysis was performed using TRFA, and the results were evaluated. The obtained data (Fig. 6B) demonstrated that the recovery values increased as the plasma dilution increased up to a 40-folds dilution. Beyond this point, the recovery values reached a level of approximately 100%. Furthermore, the RSD values decreased with increasing dilution. The lower recovery values and higher precision (lower RSD values) observed at low plasma dilutions were attributed to the impact of mass transport and mobility limitations, which are commonly observed in immune binding within viscous samples (e.g., plasma).33–35 Based on these findings, it was required to dilute plasma samples more than 50-folds before conducting the analysis to avoid false-positive results. It is important to note that the proposed TRFIA exhibits exceptional high sensitivity at the picogram level, allowing for the dilution of real clinical plasma specimens by several thousand-folds to achieve Pb concentrations within the working range of the TRFIA.
Analysis of plasma samples by TRFIA and comparison with ICP-AES
In a series of experiments, plasma samples were spiked with varying concentrations of Pb, and the samples were independently analyzed for their Pb contents by both TRFIA and atomic emission spectrometry (AES). The spiked concentrations were selected according to the sensitivity limit of AES; however, these samples were diluted to attain the Pb concentrations in the sensitivity range of TRFIA. The results obtained by TRFIA were plotted as a function of those measured by AES. The results were statistically compared, and it was found that concentrations measured by TRFIA correlated very well with those measured by AES (Fig. 6B). Linear regression analysis of the results yielded a linear equation: Y = 4.0172 + 0.9232 X (r = 0.9808), where Y is the Pb concentrations measured by AES, X is the Pb concentrations measured by TRFIA, and r is the correlation coefficient of linear relation.
The high value of correlation coefficient (r) and slope (approximately 1) revealed the reliability of the results obtained by TRFIA and confirmed the successful applicability of TRFIA for the measurement of Pb in plasma samples.
Advantages of TRFIA
The proposed TRFIA offered several advantages; these advantages are summarized in the following points. TRFIA, being based on the measurement of enhanced fluorescent signals of fluorescent Eu-chelate label, is ultra-sensitive allowing for the accurate measurement of Pb at very low concentrations (20 pg mL−1). The TRFIA offered excellent selectivity, enabling the specific measurement of Pb in the presence of other metals encountered in plasma at high concentrations. The assay is considered a high-throughput technique that enables the simultaneous analysis of multiple samples in laboratories. The assay is cost-effective as it uses very small samples and cheap commercially available reagents. Additionally, the assay did not involve tedious and time-consuming extraction and/or pre-concentration procedures for the pretreatment of plasma samples prior to analysis.
Conclusion
In this study, TRFIA was developed to measure Pb in blood plasma. The assay employed a time-resolved detection mode, which effectively reduced interference and improved measurement accuracy. A novel fluorescent chelate (Eu-DTPA)-labeled IgG was synthesized and used as a detection tracer, resulting in significantly increased fluorescence intensity. This enhancement in fluorescence intensity greatly improved the sensitivity of the assay. Under optimized conditions, the assay achieved an IC50 value of 47 pg mL−1 and LOQ of 20 pg mL−1. The sensitivity of this TRFIA was much higher than that of the previously established immunoassays for Pb,36–39 even those involved fluorescence38 or chemiluminescence39 signals for detection. Compared to instrumental analysis (e.g., AES), this TRFIA significantly reduced the use of organic solvents and simplified the plasma samples pretreatment. The established TRFIA was successfully applied to the analysis of plasma samples, and the results were verified by correlation with the AES. In conclusion, the developed TRFIA is a new ultrasensitive, high throughput, rapid, and cost-effective approach for large-scale screening and monitoring of Pb levels in plasma samples.
Abbreviations
Pb | Lead |
TRFIA | Time-resolved fluoroimmunoassay |
DTPA | Diethylenetriamine pentaacetic acid |
BSA | Bovine serum albumin |
2C33 | Mouse monoclonal antibody anti-Pb-DTPA chelate |
CDC | Centers for disease control and prevention |
EPA | Environmental protection agency |
WHO | World health organization |
EPA | U.S. environmental protection agency |
p-SCN-Bn-CHX-A′′-DTPA | [(R)-2-Amino-3-(4-isothiocyanatophenyl)propyl]-trans-(S,S)-cyclohexane-1,2-diaminepenta-acetic acid |
Eu-IgG | Anti-mouse IgG conjugated with Eu-DTPA chelate |
PBS | Phosphate buffer saline |
TTA | Thenoyltrifluoroacetone |
TOPO | Tri-n-octylphosphine oxide |
FES | Fluorescence enhancement solutions |
AES | Atomic emission spectroscopy |
LOD | Limit of detection |
LOQ | Limit of quantitation |
Conflicts of interest
The authors report no conflicts of interest for this work.
Acknowledgements
The authors extend their appreciation to the Researchers Supporting Project Number (RSPD2024R944), King Saud University, Riyadh, Saudi Arabia, for funding this research work.
References
- J. Molera, T. Pradell, N. Salvadó and M. Vendrell-Saz, Interactions between clay bodies and lead glazes, J. Am. Ceram. Soc., 2001, 84, 1120–1128 CrossRef CAS.
- WHO, Almost 1 Million People Die Every Year Due to Lead Poisoning, with More Children Suffering Long-Term Health Effects, available from, https://www.who.int/news/item/23-10-2022-almost-1-million-people-die-every-year-due-to-lead-poisoning--with-more-children-suffering-long-term-health-effects#:%7E:text=Each_year%2C_an_estimated_1,toxicity_to_the_reproductive_organ, accessed on Nov. 6, 2023 Search PubMed.
- M. Szczyglowska, M. Bodnar, J. Namiesnik and P. Konieczka, The use of vegetables in the biomonitoring of cadmium and lead pollution in the environment, Crit. Rev. Anal. Chem., 2014, 44, 2–15 CrossRef CAS PubMed.
- E. Sang Yong, L. Young-Sub, L. Seul-Gi, S. Mi-Na, C. Byung-Sun, K. Yong-Dae, L. Ji-Ae, H. Myung-Sil, K. Ho-Jang and K. Yu-Mi, Lead, mercury, and cadmium exposure in the Korean general population, J. Korean Med. Sci., 2018, 33, e9 CrossRef PubMed.
- UNICEF for Every Child, The Toxic Truth Children's Exposure to Lead Pollution Undermines a Generation of Future Potential, available from, https://www.unicef.org/reports/toxic-truth-childrens-exposure-to-lead-pollution-2020, accessed on Nov. 6, 2023 Search PubMed.
- CDC: Centers for Disease Control and Prevention, Childhood Lead Poisoning Prevention, available from, https://www.cdc.gov/nceh/lead/overview.html#:%7E:text=CDC_uses_a_blood_lead,at_or_above_the_BLRV, accessed on Nov. 6, 2023 Search PubMed.
- NIH: National Library of Medicine, Measuring Lead Exposure in Infants, Children, and Other Sensitive Populations: Methods for Assessing Exposure to Lead, available from, https://www.ncbi.nlm.nih.gov/books/NBK236457/, accessed on Nov. 6, 2023 Search PubMed.
- ATSDR: Agency for Toxic Substances and Disease Registry, Lead Toxicity: Clinical Assessment – Diagnostic Tests and Imaging, available from, https://www.atsdr.cdc.gov/csem/leadtoxicity/diagnostic_testing.html, accessed on Nov. 6, 2023 Search PubMed.
- NIH: National Library of Medicine, WHO Guideline for Clinical Management of Exposure to Lead: Diagnosis of Lead Poisoning, available from, https://www.ncbi.nlm.nih.gov/books/NBK575274/, accessed on Nov. 6, 2023 Search PubMed.
- E. J. Pacer, C. D. Palmer and P. J. Parsons, Determination of lead in blood by graphite furnace atomic absorption spectrometry with Zeeman background correction: improving a well-established method to support a lower blood lead reference value for children, Spectrochim. Acta, Part B, 2022, 190, 106324 CrossRef CAS.
- M. Atasoy, Development of a new sensitive method for lead determination by platinum-coated tungsten-coil hydride generation atomic absorption spectrometry, ACS Omega, 2023, 8, 22866–22875 CrossRef CAS PubMed.
- B. Beltran, L. O. Leal, L. Ferrerb and V. Cerd`, Determination of lead by atomic fluorescence spectrometry using an automated extraction/preconcentration flow system, J. Anal. At. Spectrom., 2015, 30, 1072–1079 RSC.
- B. G. Beltrán, L. O. Leal, L. Ferrer and V. Cerdà, Determination of lead in complex sample matrices by atomic fluorescence spectrometry: optimisation of online hydride generation, Int. J. Environ. Anal. Chem., 2015, 95, 1054–1065 Search PubMed.
- B. Ewa and W. Barbara, Quantitative aspects of inductively coupled plasma mass spectrometry, Philos. Trans. R. Soc., A, 2016, 374, 20150369 CrossRef PubMed.
- G. Zhao, X. Wang, G. Liu and N. T. D. Thuy, A disposable and flexible electrochemical sensor for the sensitive detection of heavy metals based on a one-step laser-induced surface modification: a new strategy for the batch fabrication of sensors, Sens. Actuators, B, 2022, 350, 130834 CrossRef CAS.
- M. Tarapoulouzi, V. Ortone and S. Cinti, Heavy metals detection at chemometrics-powered electrochemical (bio)sensors, Talanta, 2022, 244, 123410 CrossRef CAS PubMed.
- X. Liu, Y. Yao, Y. Ying and J. Ping, Recent advances in nanomaterial-enabled screen-printed electrochemical sensors for heavy metal detection, TrAC, Trends Anal. Chem., 2019, 115, 187–202 CrossRef CAS.
- A. Oubiña, B. B. Serra, P. B. Carrasco, R. Galve, J. Gascon, F. Iglesias and M.-P. Marco, Immunoassays for environmental analysis, in Techniques and Instrumentation in Analytical Chemistry, 2000, vol. 21, pp. 287–339 Search PubMed.
- B. Lesnik, Immunoassay techniques in environmental analyses, in Encyclopedia of Analytical Chemistry, ed. R. A. Meyers, John Wiley & Sons Ltd, Chichester, 2000, pp. 3–19 Search PubMed.
- EPA: United States Environmental Protection Agency, Field Analytical Methods: Advanced Field Monitoring Methods Development and Evaluation of New and Innovative Technologies that Support the Site Characterization and Monitoring Requirements of the Superfund Program, available from, https://cfpub.epa.gov/si/si_public_record_report.cfm?dirEntryId=56146%26Lab=NERL, accessed on Nov. 6, 2023 CrossRef; Y. Wang, C. Zhang and F. Liu, Antibody developments for metal ions and their applications, Food Agric. Immunol., 2020, 31, 1079–1103 CrossRef.
- I. A. Darwish, Z. Wang, R. J. Darling and N. Z. Alzoman, Development of two highly sensitive and selective sensor-assisted fluorescence immunoassays for trace determination of copper residues in food samples, RSC Adv., 2023, 13, 29195 RSC.
- I. A. Darwish, Z. Wang and R. J. Darling, Development and comparative evaluation of two highly sensitive immunosensor platforms for trace determination of copper ions in drinking water using a monoclonal antibody specific to copper-EDTA complex, Molecules, 2023, 28, 7017 CrossRef CAS PubMed.
- L. Jiang, P. Wang, Y. Shu, P. Jin, L. Xu, C. Xu and L. Guo, A colloidal gold immunoassay strip assay for cadmium detection in oilfield chemicals, Analyst, 2023, 148, 4166–4173 RSC.
- L. I. Yuan, M. A. O. Xiang, H. U. Jun, H. Yang and H. Wang, Application progress of time-resolved fluorescence in immunoassay, J. Public Health Prev. Med., 2021, 6, 111–116 Search PubMed.
- ThermoFisher Scientific, Pierce™ BCA Protein Assay Kits, available from, https://www.thermofisher.com/order/catalog/product/23225, accessed on Nov. 6, 2023 Search PubMed.
- A. F. Habeeb, Determination of free amino groups in proteins by trinitrobenzenesulfonic acid, Anal. Biochem., 1999, 14, 328–336 CrossRef PubMed.
- X. Chen, K. Zhou, Z. Xiang, X. Zhou, Y. Wang, J. Hong, B. Huang, Y. Qin and H. Fang, Establishment and clinical application of time-resolved immunofluorescence assay of lipoprotein-associated phospholipase A2, Anal. Biochem., 2022, 648, 114674 CrossRef CAS PubMed.
- M. M. Hamidaddin, H. AlRabiah and I. A. Darwish, Development and comparative evaluation of two immunoassay platforms for bioanalysis of crizotinib: a potent drug used for the treatment of non-small cell lung cancer, Talanta, 2019, 201, 217–225 CrossRef CAS PubMed.
- K. R. Blomberg, V.-M. Mukkala, H. H. O. Hakala, P. H. Mäkinen, M. U. Suonpää and I. A. Hemmilä, A dissociative fluorescence enhancement technique for one-step time-resolved immunoassays, Anal. Bioanal. Chem., 2011, 99, 1677–1682 CrossRef PubMed.
- J. W. Findlay, W. C. Smith, J. W. Lee, G. D. Nordblom, I. Das, B. S. DeSilva, M. N. Khan and R. R. Bowsher, Validation of immunoassays for bioanalysis: a pharmaceutical industry perspective, J. Pharm. Biomed. Anal., 2000, 21, 1249–1273 CrossRef CAS PubMed.
- A. Cesbron, E. Saussereau, L. Mahieu, I. Couland, M. Guerbet and J.-P. Goullé, Profile of whole blood and plasma in a series of 106 healthy volunteers, J. Anal. Toxicol., 2013, 37, 401–405 CrossRef CAS PubMed.
- P. Chakrabarti, F. M. Hatcher, R. C. Blake II, P. A. Ladd and D. A. Blake, Enzyme immunoassay to determine heavy metals using antibodies to specific metal-EDTA complexes: optimization and validation of an immunoassay for soluble indium, Anal. Biochem., 1994, 217, 70–75 CrossRef CAS PubMed.
- W. Kusnezow, Y. V. Syagailo, S. Ruffer, K. Klenin, W. Sebald, J. D. Hoheisel, C. Gauer and I. Goychuk, Kinetics of antigen binding to antibody microspots: strong limitation by mass transport to the surface, Proteomics, 2006, 6, 794–803 CrossRef CAS PubMed.
- W. Kusnezow, Y. V. Syagailo, I. Goychuk, J. D. Hoheisel and D. G. Wild, Antibody microarrays: the crucial impact of mass transport on assay kinetics and sensitivity, Expert Rev. Mol. Diagn., 2006, 6, 111–124 CrossRef CAS PubMed.
- K. V. Klenin, W. Kusnezow and J. Langowski, Kinetics of protein binding in solid-phase immunoassays: theory, J. Chem. Phys., 2005, 122, 214715 CrossRef PubMed.
- I. M. Mandappaa, A. Ranjinia, D. J. Hawarea, M. N. Manjunatha and H. K. Manonmani, Immunoassay for the detection of lead ions in environmental water samples, Int. J. Environ. Anal. Chem., 2012, 92, 334–343 CrossRef.
- I. M. Mandappa, A. Ranjini, D. J. Haware and H. K. Manonmani, Immunoassay for lead ions: analysis of spiked food samples, J. Immunoassay Immunochem., 2014, 35, 1–11 CrossRef CAS PubMed.
- D. K. Johnson, S. M. Combs, J. D. Parsen and M. E. Jolley, Lead analysis by anti-chelate fluorescence polarization immunoassay, Environ. Sci. Technol., 2002, 36, 1042–1047 CrossRef CAS PubMed.
- L. Xu, X.-Y. Suo, Q. Zhang, X.-P. Li, C. Chen and X.-Y. Zhang, ELISA and chemiluminescent enzyme immunoassay for sensitive and specific determination of lead (II) in water, food and feed samples, Foods, 2020, 9, 305 CrossRef CAS PubMed.
|
This journal is © The Royal Society of Chemistry 2024 |