DOI:
10.1039/D3FO03716A
(Review Article)
Food Funct., 2024,
15, 1071-1088
Fatty acid isomerism: analysis and selected biological functions
Received
2nd September 2023
, Accepted 15th December 2023
First published on 18th December 2023
Abstract
The biological functions of fatty acids and the lipids in which they are esterified are determined by their chain length, double bond position and geometry and other structural motifs such as the presence of methyl branches. Unusual isomeric features in fatty acids of human foods such as conjugated double bonds or chain branching found in dairy products, some seeds and nuts, and marine foods potentially have important effects on human health. Recent advancements in identifying fatty acids with unusual double bond positions and pinpointing the position of methyl branches have empowered the study of their biological functions. We present recent advances in fatty acid structural elucidation by mass spectrometry in comparison with the more traditional methods. The double bond position can be determined by purely instrumental methods, specifically solvent-mediated covalent adduct chemical ionization (SM-CACI) and ozone induced dissociation (OzID), with charge inversion methods showing promise. Prior derivatization using the Paternò–Büchi (PB) reaction to yield stable structures that, upon collisional activation, yield the double bond position has emerged. The chemical ionization (CI) based three ion monitoring (MRM) method has been developed to simultaneously identify and quantify low-level branched chain fatty acids (BCFAs), unattainable by electron ionization (EI) based methods. Accurate identification and quantification of unusual fatty acid isomers has led to research progress in the discovery of biomarkers for cancer, diabetes, nonalcoholic fatty liver disease (NAFLD) and atherosclerosis. Modulation of eicosanoids, weight loss and the health significance of BCFAs are also presented. This review clearly shows that the improvement of analytical capacity is critical in the study of fatty acid biological functions, and stronger coupling of the methods discussed here with fatty acid mechanistic research is promising in generating more refined outcomes.
1. Introduction
Fatty acids are the major components of all cell membranes and serve as precursors of a wide array of signaling molecules. Although most human clinical fatty acid studies focus on 10–20 common structures,1 a vast array of hydrocarbon fatty acid structures are found in nature, and each may have highly specific physiological effects.2–4 Fatty acids of human foods have predominantly cis double bonds which are methylene-interrupted when two or more are present, and are straight chain. Unusual fatty acids are isomeric to common fatty acids, such as the well-known example of dairy products with conjugated linoleic acids and branched chain fatty acids, which are isomeric to linoleic acid and straight chain fatty acids, respectively.2 Various edible although underutilized seeds such as melon, bitter melon and pomegranate seeds contain conjugated linolenic acids isomeric to both α-linolenic acid and γ-linolenic acid.5 Pine and ginkgo nuts are rich in unusual polyunsaturated fatty acids (PUFAs), pinolenic acid, sciadonic acid and juniperonic acid, just to name a few.6 The fatty acid double bond position, double bond geometry and methyl branching are major fatty acid structural motifs that determine their physiological functions. Analysis of food fatty acids can be particularly challenging, especially for foods originating from ruminants7 or marine environments.8
The most common method for fatty acid analysis is by gas chromatography/mass spectrometry (GC/MS) analysis of fatty acid methyl esters (FAMEs) derived by derivatization of any lipid.9 All fatty acyl groups esterified to any of the dozen or so lipid classes present in a typical food are converted to one form, FAMEs, similar to the digestive process that converts to non-esterified fatty acids. Although to the analytical chemist this may seem like an undesirable structural modification and compromise, it is the most effective means to reveal the underlying details of the biology specific to mammalian fatty acid biochemistry.10
The analysis of fatty acid isomers, especially double bond positional isomers and branched structures, has long been an analytical challenge. Capillary GC coupled with either flame ionization detectors (FIDs) or MS is incapable of identifying fatty acid methyl esters (FAMEs) with varying double bond positions, though it can resolve cis–trans (Z/E) isomers. The conventional means for structural identification of metabolites is to obtain purified standards that can be analyzed side-by-side with unknowns to generate matched spectra. Although this approach applies to fatty acids of major abundance, conventional fragmentation methods do not enable the identification of double bond positions: in GC/MS based analysis of fatty acid methyl esters (FAMEs), electron ionization causes double bond migration; in collisional activation, fragments characteristic of double bond positions are not obtained.11 Moreover, the number of FAME isomers for any empirical formula far exceeds the number of chemical standards that are available commercially, let alone can be practically maintained in a single laboratory. Consider for instance the number of isomers of methyl octadecenoate, represented by the chemical shorthand 18:1, where 18 is the number of carbons and 1 is the number of double bonds. This empirical formula is easily obtained from the molecular weight in MS-1. However, the double bond position can be in any of the 16 positions, from the 2–3 position to the 17–18 position. It may be cis (Z) or trans (E), and thus the number of double bond isomers is 32. Considering only single methyl chain branches, the number of chain branches extends to another 16 positions (all but COOH and CH3), providing then for 32 × 16 = 512 isomers. Di- and tri-methyls and ethyl branching are also known, adding further potential isomers. Substantial numbers of these isomers are likely to be in food mixtures, especially those that have been treated by hydrogenation or by harsh processing. Similar considerations apply to FAMEs with 2–6 double bonds, common in foods, with the number of isomers rising exponentially with the number of double bonds.
This analysis clearly shows that general approaches are needed for de novo structural analysis of FAMEs. By “de novo”, we refer to methods that reveal the structure of a FAME unequivocally with no need for genuine chemical standards. An ideal method will be rapid and produce strong diagnostic ions so that it can be applied to FAMEs of minor and trace abundance, as these may have important biological effects. Offline de novo methods have been in use since the early days of GC, first as methods such as ozone (O3) decomposition methods with subsequent GC analysis, and later by specialized derivatives (e.g. picolinyl or 4,4-dimethyl oxazoline (DMOX)) that are amenable to de novo sequencing by GC/MS where the MS is a nominal resolution quadrupole.11
These methods demonstrated the key importance of fatty acid isomers but at the cost of substantial, concentrated effort, often devoted to obtaining a sufficient quantity of analytes to yield useful signals.
Because of the cumbersome and limited nature of existing methods, our laboratory developed two general de novo sequencing methods for FAMEs that identify almost all double bond and chain branching isomers.
Early lipidomics based on the introduction of intact, unmodified lipids typically by electrospray or atmospheric pressure chemical ionization routinely ignored fatty acid isomers, a practice that limited its applicability. Since about 2015, liquid chromatography (LC)-based lipidomics has focused on de novo methods for establishing structures in acyl chains, and has at this writing reached a stage where most isomers can be identified by one or the other methods12–15 though some require offline preparation.16,17 LC and GC methods tend to be complementary.
Here, we aim to illustrate the biological functions of some unusual fatty acids isomeric to some common fatty acids, stressing the importance of employing some state-of-the-art techniques for their accurate identification, streamlining the investigation of their biological and biochemical functions, and focusing on GC methods.
2. Analytical challenges of differentiating fatty acid isomerism
2.1 Traditional gas chromatography (GC) methods
GC-FID is the most widely used method for FAME analysis; however, structure identification relies on retention time matching of authentic standards. Thus, their accuracy is directly related to column specifications such as length/polarity and the availability of authentic standards. Polar columns such as CP-Sil 88 and BPX-70 are superior in separating FAME isomers, which provides GC a clear advantage in fatty acid analysis compared to LC. Under specially designed GC conditions, such as a 200 m ionic GC column for the analysis of cis and trans fatty acid isomers in fried foods, high separation efficiency can be observed for most fatty acid isomers.18 However, coelution is still common for complex samples and MS analysis must be employed for accurate identifications.
GC-electron ionization (EI) MS data can be compared to comprehensive libraries, resulting in more confident identifications relative to GC-FID. The NIST EIMS library is a highly accessible and useful database which archives 0.3 million compounds. Unfortunately, GC-EIMS direct analysis of FAMEs similarly cannot differentiate double bond isomers. Notably, however, it can rule out many hypothetical structures based on the retention time, molecular weight, and common fragmentation.
Chemical ionization (CI) MS is also a traditional method for FAMEs. The most common CI method is a simple gas phase proton transfer, known as a “soft” ionization method because it transfers little excess energy to generate in-chain fragmentations, serving a high abundance of the molecular ion. Therefore, the method is more suitable for the analysis of low-level fatty acids. Conversion of fatty acids into pentafluorobenzyl esters and analyzed by negative CI usually significantly reduces noise compared to positive CI because of suppression of all species that do not efficiently capture electrons, thereby leading to high sensitivity. Nevertheless, none of these traditional GC methods can differentiate fatty acid isomers without special derivatization treatments.
2.2 Derivatization methods for GC-EIMS analysis
Christie has reviewed methods for structural analysis of FAMEs by GC-EIMS.19 We outline some of the more commonly used methods here.
2.2.1 DMOX.
Fatty acid derivatization into DMOX esters for fatty acid structural elucidation is still a widely employed method due to its compatibility with GC-EIMS. Two main reasons drive the appeal of DMOX to many researchers. First, GC-EIMS is widely available and relatively low cost/minimum maintenance; second, the spectra of fatty acid DMOX derivatives can be matched against an EIMS comprehensive library, such as the NIST database, streamlining the fatty acid identification process.
Some of the limitations of DMOX derivatization and EIMS analysis include labor-intensive sample pretreatment and low sensitivity of diagnostic ions. In addition, fragments from DMOX derivatives do not yield unambiguous assignment of straight chain and branched chain structures. Importantly, DMOX and similar esterification regimes are challenging for minor-abundance fatty acids in complex mixtures because retention times of specialized esters change, sometimes unexpectedly compared to FAMEs, and thus matching FA-DMOX vs. FAME was difficult. Finally, the DMOX reaction itself, if not carefully executed, can cause double bond migration.
2.2.2 Picolinyl esters.
Fatty acid picolinyl esters, upon EIMS activation, yield rich fragments including those that pinpoint double bond positions and methyl branch positions, making them more informative than the DMOX derivatization strategy. Picolinyl esters generally require column temperatures of about 50 °C higher than methyl esters, which makes the analysis of very long chain fatty acids (C ≥ 22) problematic.19 Similar to DMOX, the picolinyl ester workflow requires complicated sample preparation and does not yield high-abundance diagnostic ions indicative of double bond positions or methyl branch positions.20
2.2.3 Dimethyl disulfide.
Derivatizing FAME double bond(s) with dimethyl disulfide (DMDS) represents a convenient method to pinpoint double bond positions, with relatively high-abundance diagnostic ions specific to the erstwhile double bond positions. As a result, this method is more suitable for the discrimination of fatty acid double bond positional isomers in low abundance compared to DMOX or picolinyl ester approaches.
However, there are a few limitations of the DMDS method. First, it requires a very complicated sample derivatization procedure involving the use and cleanup of iodine (I2). Second, the GC temperatures for DMDS analysis can be as high as 320 °C, exceeding the maximum temperature limit for most polar GC columns with high resolution. Third, the spectra of DMDS derivatives contain hundreds of ions, making the screening of diagnostic ions rather difficult especially for unusual polyunsaturated fatty acids.21,22
2.3 Emerging high-sensitivity, double bond derivatizing methods
2.3.1 Solvent-mediated covalent adduct chemical ionization (GC-MS).
Solvent-mediated (SM) covalent adduct chemical ionization (CACI) is a state-of-the-art technique which enables structural elucidation of fatty acids in their FAME forms, and therefore greatly simplifies the sample preparation steps and is compatible with polar GC columns with a high resolving power. The technique takes advantage of a series of spontaneous reactions between acetonitrile and double bond(s) in a FAME hydrocarbon chain in the chemical ionization source, and upon collision induced dissociation (CID) yields diagnostic ions which are representative of the double bond positions.6 The adduction reaction between the double bond in a fatty acid chain and the (1-methyleneimino)-1-ethenylium (MIE = 54; CH2
C
N+
CH2) reactive ion is presented in Fig. 1. Depending on the number and position of double bonds, further CID activation usually results in vinylic or allylic cleavage on both sides of the erstwhile double bond position. The fragmentation rules for various FAME varieties have been worked out in several publications, with monoenes, dienes, polyenes with ≥3 homoallylic double bonds, conjugated FAMEs and polyenes with non-methylene-interrupted double bonds having nuances in spectral interpretations.5–7,23–26
 |
| Fig. 1 CACI reaction forming a nitrogen containing ring and collision induced dissociation (CID) resulting in diagnostic ions pinpointing the erstwhile double bond position. | |
SM-CACI enables de novo identification of common and unusual FAMEs without the need for authentic standards. For instance, Fig. 2 presents three isomers of 18:1 and 20:1, respectively. In this example, unusual Δ5 18:1 and 20:1 can be readily distinguished from their n-9 and n-7 counterparts in sea urchin gonads (edible parts), even without commercial standards.23 18:1n-13 has an α diagnostic ion at m/z 196 corresponding to an allylic cleavage at the far side of the carboxyl group. Here the number 141 is the mass of homolytic cleavage, and when the masses of the reactive ion (1-methyleneimino)-1-ethenylium (MIE = 54; CH2
C
N+
CH2) and one hydrogen gain are accounted for, m/z 196 is observed on the SM-CACI-MS2 spectrum (Fig. 2a). Similarly, a C2–C3 cleavage characteristic of monoenes with a Δ5 double bond leads to an ω diagnostic ion at m/z 276, having transferred a H˙ to the neutral leaving group during CID. Both n-9 and n-7 isomers of 18:1 and 20:1 yield their diagnostic ion pairs allylic to both sides of the double bond, making their de novo identification the most straightforward (Fig. 2b, c, e and f). The SM-CACI-MS2 spectra for the identification of polyunsaturated FAMEs and other unusual FAMEs by SM-CACI can be found in a series of previous publications.5–7,23,24
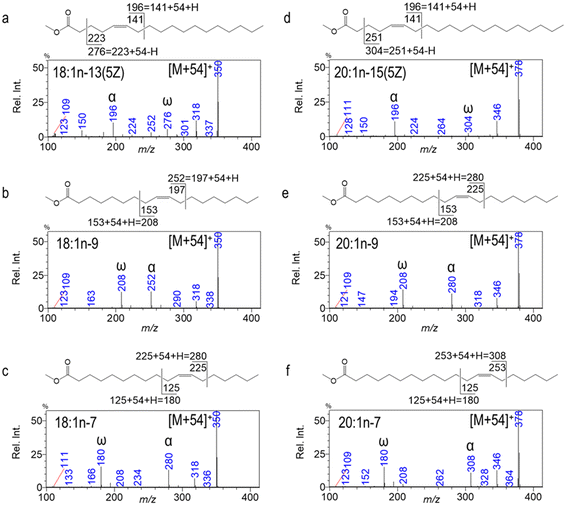 |
| Fig. 2 CACI-MS/MS spectra of unusual Δ5 18:1 and 20:1; all [M + 54] → products. Reprinted with permission from Wang et al.23 Copyright 2021 Elsevier Ltd. | |
The SM-CACI technique is currently the only online derivatization mass spectrometric method coupled with a GC system, eliminating fatty acid manual derivatization. Importantly, it is the only method which allows the separation of FAMEs on the GC column before making FAME derivatives, making it highly compatible with any analytical method established on a GC-FID, and thus requiring no further offline chemistry. Briefly, the SM-CACI technique has the following advantages compared to conventional derivatization methods such as picolinyl ester: (A) no need to develop special chromatography for new esters, i.e., higher temperatures. (B) Sample preparation is simple, and the preparation of FAMEs is the only procedure required. (C) Locating double bond positions of low-abundance fatty acids is possible due to its high diagnostic ion abundance and little other intrachain fragmentation.
2.3.2 Ozone induced dissociation (LC-MS).
Ozone induced dissociation (OzID), first established by Blanksby and coworkers, has been extremely useful for the discrimination of phospholipid double bond positional isomers. It is also invaluable for fatty acid isomer identification when an LC system is employed for their separation. Recently, a workflow involving the first derivatization of fatty acids into their N-(4-aminomethylphenyl)pyridinium (AMPP) derivatives followed by OzID activation was developed as a highly sensitive tool for fatty acid profiling and double bond position assignments.27
OzID generates very few intrachain fragments and high diagnostic ion abundance, enabling straightforward double bond position assignments. However, this online technique must be implemented on a customized MS instrument, compromising its easy access. An offline version was attempted by others28 but as it does not enable mass selection before ozonolysis, it requires either pure samples or compatible mixtures. Offline ozonolysis of mixtures gives rise to extremely complicated spectra with ozonolyzed fragments originating from all fatty acids present in the original sample. LC, however, has a major advantage that sample preparation can be simpler for fatty acids, consisting of simple hydrolysis, and has no limitations for volatilization of low or high mass fatty acids. Generally, LC equipment is considerably more costly than GC, and GC chromatographic resolution can be greater.
2.3.3 Paternò–Büchi reaction (LC-MS).
Paternò–Büchi reaction mass spectrometric analysis of lipid double bond positions was first developed by Xia and coworkers, serving as an alternative to OzID. It is also compatible with LC and generates very few intrachain fragments with high diagnostic ion abundance, enabling straightforward double bond position assignments. The method has quickly become the most popular workflow for glycerolipid double bond position assignments. It has been adopted and adapted for various analyses, mainly due to its relatively simple derivatization apparatus – a UV lamp. It can be conveniently employed as a sample pre-treatment step or implemented into the LC-MS workflow as an online derivatization approach, as demonstrated by Xia et al.29–32 and others.33
Fig. 3 presents the formation of the PB reaction adduct with 2-acetylpyridine as the derivatizing reagent. In the presence of UV light, a double bond on the acyl chain of a lipid molecule reacts with a ketone containing reagent, for instance, 2-acetylpyridine, acetone, etc., and forms an oxygen containing ring called oxetane. Upon CID activation, site-specific cleavage at the erstwhile double bond position can be observed, and the fragments are used as the tool to pinpoint the double bond location. The resulting derivative is remarkably stable and thus can be manually admitted to the MS by infusion.
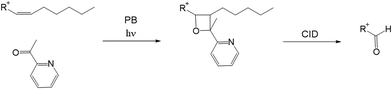 |
| Fig. 3 Formation of a Paternò–Büchi reaction adduct with 2-acetylpyridine as the derivatizing reagent and formation of an aldehyde diagnostic ion. | |
Considering its simple derivatization hardware requirements, the PB-MS/MS workflow is promising as a routine method to elucidate fatty acid isomeric features. Early work by Murphy et al. showed that the PB-MS/MS workflow did not result in highly interpretable spectra for polyunsaturated fatty acids, analyzed by their free form as negative ions.34 However, further refining of the derivatization process yields highly interpretable PB-MS/MS spectra for polyenes including docosahexaenoic acid (DHA). Simply put, this was achieved either by double derivatization of the carboxyl group (N,N-diethyl-1,2-ethanediamine) and the double bond by PB (acetone),35 or using a nitrogen containing PB reagent such as acetylpyridine,33 or otherwise promoting the formation of a lithium adduct.36 These methods allow fatty acids to be analyzed in the positive mode. While the CACI-MS/MS workflow tends to have greater sensitivity and simpler data analysis thanks to its compatibility with high resolution GC, the PB-MS/MS workflow possesses straightforward fragmentation patterns and simple mass spectra with high diagnostic ion abundances, offsetting LC's normally lower separation capacity.
The PB-MS/MS workflow was implemented for a specialized application, for quantitative analysis of deuteration levels at bis allylic positions of polyunsaturated fatty acids. In this particular case, GC-CACI-MS/MS was not possible due to the presence of companion ions (±1 Da or ±2 Da) of the CACI diagnostic ions.17,37 This special case of analysis of a unique pharmaceutical-like species illustrates the importance of detailed mass spectral interpretation and mechanism for new analytes.
2.3.4 Other double bond derivatizing methods.
There are also some other emerging derivatization methods similarly reacting with a double bond in a fatty acyl chain, yielding high-abundance diagnostic ions for convenient, highly sensitive and de novo identification of unsaturated fatty acid double bond variants, summarized in Fig. 4 along with the others. Among these is N-tosylaziridine derivatization, which replaces a double bond with a nitrogen containing cyclo-ring linked to some sulfur containing moieties. Similar to the CACI-MS/MS workflow, the positive charge remains with the nitrogen atom. During CID activation, bonds of the nitrogen containing cyclo-ring are all labile and tend to dissociate. Depending on which of the labile bonds is broken, two diagnostic ions indicating the erstwhile double bond position can be observed.16
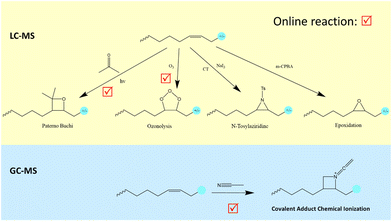 |
| Fig. 4 Emerging double bond derivatizing MS methods for fatty acid identification. | |
Epoxidation is another double bond derivatizing strategy. Epoxidation can be induced by the meta-chloroperoxybenzoic acid (m-CPBA) reaction with double bond(s) in a fatty acyl chain at room temperature.38 These two double bond derivatizing methods do not require any additional devices such as a UV lamp in the PB reaction or modifications to the MS instrument seen with CACI and OzID. However, they require sample offline pre-treatment, hampering their throughput compared to the online versions of CACI, PB and OzID methods. Fig. 4 summarizes the emerging double bond derivatizing MS methods and their compatible chromatographic coupling, which can be used to interrogate fatty acid structural information.
2.3.5 Charge switching chemistry.
Charge switching chemistry, or ion/ion charge inversion chemistry was developed by McLuckey and coworkers, an innovative method for the release of fatty acyl chains from a glycerolipid molecule or for analysis of fatty acids themselves. Without structural modifications or adduction, fatty acids can only be analyzed in the negative mode. Previously, fatty acyl chains released from a glycerolipid molecule would be detected in the negative ion mode, which yields spectra dominated by H2O and CO2 neutral loss fragments with little structural information. Charge switching chemistry takes advantage of the reaction between tris-phenanthroline magnesium dications (+2 charge) and fatty acid anions (−1 charge) resulting in a cation (+1 charge), shown in Fig. 5. This is a two-step reaction, i.e., (1) ion/ion reaction yielding a complex with two phenanthroline ligands and (2) dipolar direct current (DDC) – CID removal of one of the two remaining phenanthroline ligands. Upon ion trap CID activation of the resulting fatty acid (single) phenanthroline magnesium ligand (Fig. 5), the cation yields a spectrum with rich fragmentation, at typically all carbon–carbon bond sites. A distinct difference in intensity near the double bond site or sometimes the presence of doublets at some cleavage sites indicates the location of the double bond(s). As the degree of unsaturation increases, the spectra become complicated.39 Compared to the double bond derivatizing methods such as CACI, OzID, PB, N-tosylaziridine and epoxidation, the charge switching chemistry mass spectrometric method requires relatively in-depth expertise of the method for data interpretation. A more practical approach to exploit the merits of charge switching chemistry MS to identify fatty acid isomers involves the use of library matching, which has also been demonstrated.40 Information covering most of the charge switching chemistry advancements can be found in a previous review.41
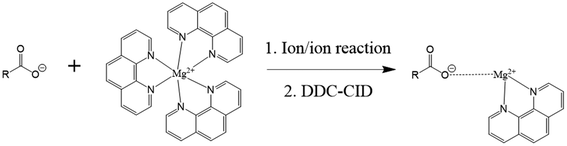 |
| Fig. 5 Two-step formation of fatty acid phenanthroline magnesium ligands. | |
2.3.6 Photodissociation and electron impact excitation of ions from organics (EIEIO).
Besides various state-of-the-art derivatization and adduction methods capable of pinpointing double bond positions, there is an alternative strategy which seeks solution from innovative dissociation technologies built within the MS instrument, substituting it for CID or other conventional dissociation mechanisms. UV photodissociation utilizes short wavelength UVC to induce light-based dissociation of fatty acids and other lipids. In a pioneering study by Klein and Brodbelt, the structure of phosphatidylcholine (PC) was elucidated including the double bond positions.42 Blanksby and coworkers adapted the principle and further demonstrated that with some suitable derivatizing reagents which are superior in absorbing the UV energy, monoene double bond positions could be revealed by manifestation of the fragmentation of an allylic bond to a double bond. Isomeric polyenes such as 18:3n-3 and 18:3n-6 could be readily distinguished by their clearly different spectra, however, straightforward localization of double bond positions is complicated by some rearrangement mechanisms around the double bond cleavages.43
Electron impact excitation of ions from organics (EIEIO), as an emerging ion fragmentation method, was developed recently. Its dissociation is also characterized by rich ion formation along the fatty acyl chain and a sharp decrease in ion abundance along the hydrocarbon chain indicates the presence of a double bond. This fragmentation pattern is reminiscent of the picolinyl ester-GC-EIMS method, with fragmentation conspicuously attenuated at the stronger double bond position. EIEIO was shown to elucidate structural elements, e.g., double bond positions and cis/trans configurations of phosphatidylcholines, triglycerides and sphingomyelins.44–46 Research is warranted to confirm that EIEIO is equally effective in determining fatty acid isomer compositions compared to methods further developed.
2.4 Identification of branched chain fatty acids (BCFAs)
2.4.1 Traditional picolinyl ester method by EIMS.
BCFAs are common in certain foods,47,48 bacteria,49 as well as in some human tissue, notably skin.50 Unlike for the double bond position, GC-EIMS provides information about branch points, though often the data are ambiguous.51 Derivatization of fatty acids into picolinyl esters and analyzed by conventional GC-EIMS is the earliest method for effective identification of saturated BCFAs from their straight chain counterparts. Dating back to the 1980s, Harvey used picolinyl esters as derivatives to determine the monomethyl branch position along a fatty acid picolinyl ester chain.20 Later Hierro et al. identified the anteiso-17:0 BCFA in ginkgo nuts taking advantage of the robust analytical capability of picolinyl esters.52Fig. 6 shows the positions of cleavage leading to high-abundance diagnostic ions, i.e. m/z 318 and m/z 346 for the identification of iso-17:0 and m/z 304 and m/z 332 for the identification of the anteiso-17:0 BCFA derivatized into picolinyl esters. Using anteiso-17:0 for illustration, there is no cleavage between the two respective cleavage positions due to the presence of a methyl branch at the butan-2-yl terminal position (negligible levels of m/z 318 in its spectrum).
 |
| Fig. 6 Diagnostic ions for the identification of iso-17:0 and anteiso-17:0 BCFAs derivatized into picolinyl esters by GC-EIMS. | |
2.4.2 Direct FAME analysis by EIMS/MS.
Inspired by the work of Zirroli and Murphy,53 Ran-Ressler et al. demonstrated that when tandem MS was available, a similar fragmentation pattern could be observed for anteiso-17:0 and other BCFA in their direct FAME form.51Fig. 7 presents the pair of high-abundance diagnostic ions m/z 227 and m/z 255 for the straightforward identification of anteiso-17:0 by GC-EIMS/MS. Identical to what is observed for the picolinyl ester analysis by GC-EIMS, the 14 Da (CH2) interruption is missing between m/z 227 and m/z 255 (negligible levels of m/z 241) for EIMS/MS analysis.
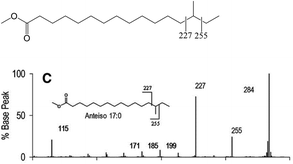 |
| Fig. 7 EIMS/MS spectrum and diagnostic ions for the identification of the anteiso-17:0 FAME. Source: Ran-Ressler et al.51/Elsevier/CC BY 4.0. The fragmentation patterns and intensities of diagnostic ions near the methyl branch position are similar to the picolinyl ester derivatization method (cf. 2.4.1). | |
2.4.3 Three ion monitoring (MRM) of low-level BCFAs.
While picolinyl ester analysis by EIMS and direct FAME analysis by EIMS/MS are both effective methods of localizing methyl branch positions, the former requires laborious derivatization efforts and special chromatography as mentioned above, and the latter has a compromised sensitivity due to strong fragmentation in both hard EI ionization and collisionally activated dissociation stages. We recently developed a chemical ionization (CI) based methodology termed “three ion monitoring” as a routine analytical workflow for samples containing BCFAs of various chain lengths. The CI method works directly towards FAMEs, and can identify and quantify trace-level BCFAs due to a sensitivity boost by a soft CI ionization method and an MRM data collecting mode.54Fig. 8 shows that BCFA iso form, anteiso form and straight form can be readily distinguished by the relative abundance of [M-43]+ and [M-57]+ ions. Thanks to the uniform response factors of branched chain FAMEs and straight chain FAMEs yielded by this CI method, quantitative analysis can be conducted without the need for rare BCFA standards.55
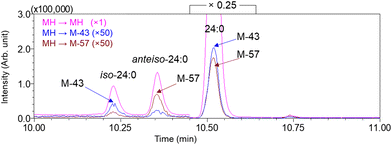 |
| Fig. 8 Iso-24:0 is characterized by high [M-43]+ (blue trace) in contrast to high [M-57]+ (brown trace) in anteiso-24:0. Straight chain 24:0 has both ions at similar levels. Reprinted with permission from Wang et al.54 Copyright 2020 American Chemical Society. | |
2.4.4
De novo analysis of monounsaturated BCFAs.
Having introduced a couple of effective methods of saturated BCFA identification, the complete structural elucidation of monounsaturated BCFAs was a very challenging task due to the existence of two positional variants along their hydrocarbon chain, i.e., the uncertain positions of a methyl branch and a double bond. Monounsaturated BCFAs are uniquely found in human surface lipids (sebaceous gland excretion) and their unambiguous identification is important for the study of human skin functionality and dermatology, such as the function of the fatty acid desaturase 2 (FADS2) gene in skin integrity and health.50 We recently developed a two-pronged approach to address this challenge. CACI-MS/MS analysis would establish the point of unsaturation while the methyl branch structure is unresponsive to the method. Another analysis, EIMS/MS, could discriminate straight, iso and anteiso monounsaturated BCFA methyl esters (BCFAMEs) by a modified method compared to saturated BCFAMEs, due to the influence exerted by the double bond on the EIMS/MS spectra. Ratios of [M-32]+/[M-43]+ are exploited to differentiate straight and iso structures while a strong [M-29]+ indicates the presence of a methyl branch at the antepenultimate position (anteiso).24
2.4.5 Charge switching chemistry for cyclopropane fatty acids and BCFAs.
Charge switching chemistry was proven to be a useful tool to characterize cyclopropane and methyl branch structures in fatty acids and fatty acyl chains of a glycerolipid. A few subtle differences in ions could be exploited to distinguish a cyclopropane and a double bond at the same position along a hydrocarbon chain. Although it has not been confirmed, a list of expected mass values of neutral loss for varying cyclopropane positions could be used for cyclopropane fatty acid identifications.56 With respect to BCFAs, charge switching chemistry has limited resolving capability of the anteiso BCFA from iso to straight chains, but not between iso and straight chains. However, it is still a major advancement in BCFA fingerprinting within a larger glycerolipid molecule, allowing top-down lipid structural analysis.14
Traditional ESI-based lipidomics with little to no fatty acyl structure elucidation has been widely applied to investigate biomarkers of health and disease. The methods described here for fatty acid and fatty acyl identification now enable full structural analysis of an ever-widening array of intact lipids as well as complex fatty acid structures. In parallel, these techniques have been applied to the discovery of novel fatty acid structures and/or unusual fatty acid isomers indicative of disease states. We briefly review recent results that depend on the unambiguous detection of unusual isomers in disease states to illustrate the power of these techniques.
3. Fatty acid isomerism in cancer
3.1 Anti-cancer effects of fatty acids
Cancer can be thought of as runaway cell replication. The synthesis of fatty acids including PUFAs is a major structural requirement for cell proliferation, though abnormalities in fatty acid biosynthetic genes can lead to unusual structures.57 Metabolism is altered toward glycolytic production of energy (ATP) and away from the electron transport chain, a phenomenon known as the Warburg effect.58 Various unsaturated fatty acid isomers have been studied in relation to cancer where food fatty acids modify carcinogenesis or appear to be a product of cancer cells.
3.1.1 Conjugated linoleic acids.
Conjugated linoleic acids (CLAs) are a group of fatty acids with a conjugated double bond system, with rumenic acid (9Z,11E-18:2) naturally found in dairy food. However, supplements of CLAs are synthesized by isomerization of linoleic acid which contains a mixture of various CLA isomers with 9Z,11E-18:2 and 10E,12Z-18:2 dominating. A wide range of animal and in vitro studies have suggested the anti-cancer effects of CLAs, including breast,59–61 colon,62–65 stomach,66,67 prostate63,68–70 and liver cancers.71 However, there is significant controversy about which of the CLA isomers exert these anti-cancer effects. For example, 9Z,11E but not the 10E,12Z-18:2 isomer of CLA has a positive effect on prostate cancer;70 on the other hand, another study showed that the 10E,12Z-18:2 isomer has a cytotoxic effect on rat hepatoma.71
Evidence from human clinical trials is scarce. One study found that the administration of CLA to breast cancer patients had some inhibitory effects on fatty acid synthesis. However, not all the measured parameters pointed to such a correlation and a mere number of 23 participants were enrolled, rendering its finding questionable.59 Another study showed some beneficial effects of CLA supplementation on inflammatory factors and matrix metalloproteinase (MMP) enzymes in a small cohort of rectal cancer patients.72
As of now, there is insufficient human trial data confirming any therapeutic effects of CLAs on various cancers. Some stronger correlation of CLAs with anticarcinogenic properties, e.g., against colorectal cancer was attributed to dairy intake where CLAs may only serve as a biomarker;73 however, another study did not find any correlation between CLA rich dairy food and breast cancer incidence.74
3.1.2 Conjugated linolenic acids (CLnAs).
Similar to the CLA situation, the suggested anticarcinogenic properties of CLnAs are also largely based on animal and in vitro studies. Dietary seed oils rich in conjugated linolenic acids (CLnAs) from bitter melon and pomegranate, although with the major CLnAs being isomers, were both found to inhibit colon cancer.75,76 Cancer cell death and tumor growth inhibition were observed for α-eleostearic acid (9Z,11E,13E-18:3)77 and jacaric acid (8Z,10E,12Z-18:3) was found to induce leukemia and adenocarcinoma (DLD-1) cell death.78,79 The absence of clinical trials investigating the effects of CLnAs against cancer development prevents the conclusion of its effectiveness. One human study showed that punicic acid (9Z,11E,13Z-18:3) could be metabolically converted into rumenic acid (9Z,11E-18:3), which might partially explain the mechanisms of CLnA in suppressing cancer growth.80
3.1.3 Ximenynic acid.
Ximenynic acid is an 18 carbon enynic acid predominantly found in the seed oils of the Santalaceae, Olacaceae, and Opiliaceae families. Ximenynic acid inhibited the growth of HepG2 cells by suppressing COX-1 gene expression.81 The health effects of ximenynic acid are very limited, and more on its positive roles in regulating insulin resistance and diabetes will be discussed in section “4.1 Diabetes”.
3.2 Shift of fatty acid pathways in cancer cells
3.2.1 Isomeric 18:1(Δ11) as cancer cell markers.
The advent of precision lipidomics around the localization of lipid double bond positions has contributed to the advancement of research in various fields, including the study of cancers. In 2016, Xia's group demonstrated the ability of an emerging double bond derivatizing MS method to distinguish normal and cancerous mouse breast tissues by the abundance of 18:1(Δ11).30 Although the method could not show whether 18:1(11Z) or 18:1(11E) was elevated in cancerous mouse breast tissues, it prompted a wave of studies investigating the correlation between isomeric lipid species and cancers. Human breast cancer tissues were analyzed by the same research group and showed significant differences in the concentrations of isomeric phospholipids containing 18:1(Δ9) and 18:1(Δ11) fatty acyl chains.82 Another group of researchers used a different double bond derivatizing method to interrogate phospholipids containing 18:1(Δ9) and 18:1(Δ11) fatty acyl chains, also from human breast cancer tissues and human breast healthy tissues.83 Although these studies did not result in completely consistent findings, probably due to very small sample sizes (n < 10), some isomers, e.g. PC 18:1–18:1 had consistently higher 18:1(Δ11) for cancerous tissues among the one mouse and two human breast cancer tissue studies.30,82,83 A more comprehensive study then compared human breast cancer tissues, human plasma from type-2 diabetes patients and human lung cancer tissues to their healthy counterparts. The study concluded that double bond positional isomers led to the differentiation of human breast cancer tissues and human plasma from type-2 diabetes patients from their normal samples; when sn-isomers were also taken into account, human lung cancer tissues could also be screened.29
3.2.2 Sapienic acid.
Sapienic acid (16:1n-10) is almost uniquely found in human skin surface lipids. As a product of the 6-desaturase enzyme FADS2, its biosynthesis competes with n-6 and n-3 PUFA biosynthesis, especially γ-linolenic acid (18:3n-6) and stearidonic acid (18:4n-3).84 As predicted from those in vitro results,85 sapienic acid was detected in cancer cells including murine hepatocellular carcinomas (HCCs), and primary human liver and lung carcinomas, indicating that palmitic acid (16:0) was outcompeting PUFAs for membrane fatty acid biosynthesis during rapid proliferation.86 Based on this extraordinary finding, another research group showed that two human prostatic adenocarcinoma cell lines of different aggressiveness preferentially synthesized sapienic acid.87 The researchers found that sapienic acid and its C18 elongated product at 6–8% and 3–6% (wt/wt of total fatty acids), respectively. As a result, sapienic acid has become a spotlight in the cancer research field, providing insights into the developmental mechanisms of cancer cells and a breakthrough point for biomarker discovery in the prognosis of various diseases. These high levels of fatty acids with unusual double bond positions highlight their prospect in all aspects of cancer research. Recently, Xia's group used the double bond derivatizing method they developed to investigate n-10 fatty acids in human breast cancer cells. Due to the excellent isomers resolving power of their method, they unambiguously demonstrated that sapienic acid and other n-10 fatty acids were significantly enriched in more than a dozen aminophospholipid species from a breast cancer cell line than a normal breast cell line, corroborating the findings by previous researchers.88 These studies surely open up a new line of cancer research, serving as proof of concepts with preliminary data due to their limited numbers of samples (n < 10).
3.2.3 Fatty acid desaturase (FADS) abnormalities in cancer and fatty acid changes.
The intriguing changes of fatty acid composition in cancer biomasses are correlated with the changes of activities by some genes, for example, those responsible for introducing double bonds to specific locations, i.e., stearoyl-CoA desaturase (SCD) and fatty acid desaturase 2 (FADS2). The functionality of the FADS2 gene is deranged in many cancers.57 In MCF7 human breast cancer cells, FADS2 activity is absent, suppressing the biosynthesis of the normal eicosanoid precursor arachidonic acid.89 The absence of FADS2 unmasked the synthesis of various unusual fatty acids via the FADS1 directed pathways in vitro. In vivo, 3 of 9 human breast tumor samples contained sciadonic acid 20:3(5Z,11Z,14Z), a fatty acid synthesized by FADS1 desaturation of 20:2n-6 and rarely found in any normal human tissues.90 With the assistance of isomer-resolved lipidomics, i.e. OzID, Blanksby's group found a wide range of unusual fatty acids including n-8, n-10, and n-12 monounsaturates biosynthesized by FADS2 in prostate cancer cell lines and tissues. These unusual fatty acids such as 18:1n-10 could be further desaturated by FADS1 mediated pathways to form 18:2n-10 by inserting a Δ5 double bond.91
4. Fatty acid isomerism in non-cancerous diseases
4.1 Diabetes
The probe of diabetes lipid biomarkers has been a constant endeavor, with isomer-resolving or double bond derivatizing methods exhibiting great promise. OzID is one of the first methods developed and thus its inventors investigated this issue dating back to 2012. In the study, the researchers identified that some TAG species, especially those with n-7 and n-9 double bond positions had significantly different relative abundances between normal and diabetic plasma samples.92 Xia's group found that the ratios of C
C isomers enjoyed less variations between individuals, and could possibly serve as a better tool than their individual abundances. They showed that one type of phosphatidylethanolamine, PE 16:0_18:1 could be used as a biomarker for type-2 diabetes when their Δ9 and Δ11 isomers were resolved and quantified.82 In their continued work, they concluded that discriminations of plasma samples from normal and diabetic patients could be made by analyzing a dozen C
C location isomers or, alternatively, 22 sn-position isomers.29
Recently, ximenynic acid was found to be beneficial for diabetes by regulating the liver inflammation related interference to the insulin signaling pathway and intestinal microbiota in rats. Pro-inflammatory factors including IL-6, IL-1β and TNF-α, and the JNK/NF-κB inflammatory signaling pathway were all inhibited. While the PI3K/AKT insulin signaling pathway was promoted by ximenynic acid rich sandalwood seed oil.93,94
4.2 Nonalcoholic fatty liver disease (NAFLD)
Some studies found a link between the ratios of C
C isomers and nonalcoholic fatty liver disease (NAFLD). Specifically, the ratios of 18:1n-9/18:1n-7 and 18:3n-3/18:3n-6 could discriminate control and NAFLD mouse liver samples.35 In contrast to serving as biomarkers for NAFLD, another study showed the preventive effects of 18:3n-6 against it owing to the fatty acid's roles in lipid metabolism and autophagy.95
4.3 Atherosclerosis
Research into unusual fatty acid isomer roles or their relative abundance in atherosclerosis has been mainly focused on CLA. For reference, den Hartigh extensively reviewed this area.96 In brief, the review analyzed the findings of various animal studies and confirmed the positive efficacy of CLA against atherosclerosis. In a recent publication, the same group demonstrated the underlying mechanisms of CLA's atheroprotective effects, attributed to increasing high-density lipoprotein (HDL) particle concentration, HDL anti-inflammatory potential, and promoting beneficial effects on cholesterol efflux.97
5. Eicosanoid modulation
5.1 Sciadonic acid and arachidonic acid
Sciadonic acid, or 20:3(5Z,11Z,14Z), is an unusual fatty acid isomer of 20:3(8Z,11Z,14Z), which is a precursor of arachidonic acid, 20:4(5Z,8Z,11Z,14Z). Sciadonic acid has long been known to replace circulating arachidonic acid.98 There has been interest in using sciadonic acid to modulate eicosanoid production and thus modulate many physiological responses including the immune system. Research has been focused on the interactions between sciadonic acid and prostaglandin E2 (PGE2). Supplementation of sciadonic acid decreased the production of a pro-inflammatory mediator, PGE2, by 4–29% in mouse macrophages, presumably due to the inhibition of inducible nitric oxide synthase (iNOS) and cyclooxygenase-2 (COX-2).99 In a follow-up, the same authors corroborated their initial findings in a murine microglial BV-2 cell model, and extended the eicosanoid modulatory effects to two other non-methylene-interrupted fatty acids, i.e. 18:3(5Z,9Z,12Z) and 20:3(7Z,11Z,14Z).100 Using an epithelial cell line, some scholars found that during pathogenic yeast infection, sciadonic acid supplementation could inhibit the production of PGE2 without changing the production of ω-3 fatty acid metabolites which could counteract the normal inflammatory process necessary to combat infections.101
6. Weight loss and conjugated fatty acids
6.1 Conjugated linoleic acids
Besides anticancer effects, another research front line of CLAs is around weight loss. Park and coworkers examined the effects of CLAs on mice body composition, and found a reduction in fat deposition and an increase in lipolysis in adipocytes.102 Also, they tested the respective effects of common isomeric CLAs, i.e., 9Z,11E-18:2 and 10E,12Z-18:2, and found that only 10E,12Z-18:2 could reduce body fat in mice. The weight loss effect was found to be linked to heparin-releasable lipoprotein lipase (HR-LPL) activity.103 In a continued study by the same authors, they showed that only 10E,12Z-18:2 and its alcohol form could inhibit the HR-LPL activity among a wide range of structural variants.104 Due to the scarcity of human clinical trials and even fewer reporting positive outcomes, one review by Fuke et al. did not explicitly indicate CLA's efficacy on human weight loss.105 However, another review by den Hartigh suggested a positive link between 10E,12Z-18:2 and obesity, based on the evidence from preclinical trials and a few clinical trials finding either fat mass reduction or weight loss.96
6.2 Conjugated linolenic acids
The weight-losing effects of CLnA (18:3) may share a similar chemical and physiological mechanism with a CLA (18:2). Calendic acid (8E,10E,12Z-18:3) shares the same 10E,12Z structure with 10E,12Z-18:2, with an additional trans double bond at the C8 position. Calendic acid was found to have some weaker effects towards body fat reduction than 10E,12Z-18:2.106 The CLnA was found to reduce perirenal and epididymal adipose tissue weight to a greater extent than the CLA.107 However, jacaric acid (8Z,10E,12Z-18:3) which also contains the 10E,12Z structure, was found to have no effects on body fat reduction.108
7. BCFAs and health
BCFAs have been found in microbial lipids at high levels a long time ago, but are also present in other organisms including the nematode Caenorhabditis elegans (C. elegans) and mammals.109 Ruminant fats, along with some seafood and Asian fermented foods, are BCFA-rich food sources for mammals, which have been well documented in a previous review.110 BCFAs from the diet undergo de novo syntheses in organisms from bacteria to mammals and exhibit diverse health effects. Among various BCFA isomerism, the monomethyl BCFA (mmBCFA), especially long-chain ones, attracted the most attention in recent decades, due to their close involvements in metabolic regulation, anti-inflammation, anti-cancer, weight control, as well as neuroprotection and cardioprotection, as summarized in previous reviews.111–113 Thus, here we aim to emphasize the significance of BCFAs in two important but not well reviewed aspects: the essential role in C. elegans development and the special support to human skin functions.
7.1 BCFAs are essential for C. elegans
The nematode C. elegans is a common model used in human nutritional studies for evaluating diet-related aging and longevity, obesity and the molecular basis of nutrition.114 It was found in 2004 that mmBCFA C15iso and C17iso were essential for C. elegans growth and development, since suppressing their biosynthesis via ELO-5 and ELO-6 could arrest C. elegans larva development, and this situation could be reversed by giving BCFA supplements.115 Another study showed that C17iso and its downstream metabolite glycosphingolipid could restore the C. elegans larva development by activating intestinal and neural mTORC1 under dietary amino acid deficiency.116 C17iso was also found to be incorporated into phospholipids to ensure the membrane integrity of the endoplasmic reticulum for lipid droplet growth in C. elegans.117 Besides, the existence of the branched side chain in mmBCFA contributes to the much lower melting point compared to the normal straight chain fatty acid with the same carbon number and saturation degree, which increases the membrane fluidity in multiple organisms. A study demonstrated decreased membrane fluidity induced by high-glucose stress in C. elegans, and showed that mmBCFA could rescue C. elegans in a high-glucose environment, suggesting the critical role of mmBCFAs in adaption to elevated glucose conditions.118
7.2 BCFAs support human skin function
Previous research identified BCFAs in human blood, adipose tissues, brain and skin.112,115 Notably, BCFA isomerism showed both great richness and diversity, especially in skin, and connective tissues including skin sebaceous glands (SGs) and eyelid meibomian glands (MGs), as well as their secretion products such as vernix caseosa covering the newborn skin. An early paper established that the human skin fatty acid profile is unique compared to that of internal organs in the desaturation degree and fatty acid isomerism: more than 97.1% of human skin fatty acids are saturated or monounsaturated; and among them, saturated and monounsaturated BCFAs (SBCFAs and MUBCFAs) account for 5.4% and 6.6% of total fatty acids, respectively, and accumulate mainly in wax esters (WEs) of skin lipids.119 In particular, MUBCFAs with both double bond and methyl side chains were detected and presumed to assist with human sebum fluidity so as to support normal skin function, such as enhancing tolerance to extreme environmental temperatures.50
Several clinical studies have associated the unique skin BCFA profile with human skin function or disease pathogenesis. For example, a cross-sectional analytical study conducted in Nigeria in 2021 observed significantly lower levels of the anteiso-even chain BCFA with 12–18 carbons and higher levels of the normal straight chain SFA with 15–17 carbons in the facial sebum lipid profile of the acne group compared to the non-acne group.120 Another earlier study also found the opposite change tendency of the BCFA and straight chain FA with aging: the abundance of sebum WE iso-16:1 decreased from infancy to around 20-year-old, and then increased until around 50-year-old, whereas straight chain 16:1 followed a completely reversed course.121 A pilot study designed for applying skin sebum lipidomics in neurodegenerative disease discovery showed that both even chain and odd chain BCFAs in sebum were negatively correlated with the BMIs in both healthy and Alzheimer's disease/Parkinson's disease subjects.122
Meibum is a lipid-based substance synthesized in the meibomian glands of the human eyelids, excreted on to surface of the tear film to reduce evaporation. Abnormalities in meibomian gland function cause dry eye. A series of studies showed that meibum BCFA from C16–C26 in both iso- and anteiso-forms were significantly increased, while SFAs especially C16 and C18 were decreased, in meibomian gland dysfunction (MGD) patients.123 The accumulation of BCFAs in MGD appeared to be compensation for tear film fluidity as an adaption to tear loss.124
Vernix caseosa, or vernix in short, is secreted by newborn SG during the 3rd trimester of gestation, covering the skin of a full-term baby during delivery, and providing as high as 29% of BCFAs for the fetus GI tract.125,126 The function of vernix BCFAs has been summarized thoroughly in previous reviews.111,113 In short, vernix BCFAs exhibited the ability to alleviate intestinal inflammation in both in vitro and in vivo models,127,128 which indicated its potential in modulating a newborn immune system, though more research is warranted to expand this area. All the aforementioned functions of unusual fatty acid isomers are summarized in Table 1.
Table 1 Summarized functions of unusual fatty acid isomers
Unusual fatty acid isomers |
Biological functions |
Type of study |
References |
Conjugated linoleic acid (CLA) |
Anticancer (breast cancer) |
Clinical |
McGowan et al.59 |
Anticancer (breast cancer) |
Cell lines |
Rakib et al.60 |
Anticancer (breast cancer) |
Animal |
Futakuchi et al.61 |
Anticancer (colon cancer) |
Animal |
(1) Bassaganya-Riera et al.62 |
|
|
(2) Shiraishi et al.65 |
Anticancer (colon cancer) |
Cell lines |
(1) Lau et al.63 |
|
|
(2) Soel et al.64 |
Anticancer (stomach cancer) |
Animal |
(1) Chen et al.66 |
|
|
(2) Ha et al.67 |
Anticancer (prostate cancer) |
Cell lines |
(1) Lau et al.63 |
|
|
(2) Kim et al.69 |
|
|
(3) Song et al.70 |
Anticancer (prostate cancer) |
Animal |
Cesano et al.68 |
Anticancer (liver cancer) |
Animal |
Yamasaki et al.71 |
Anticancer (rectal cancer) |
Clinical |
Mohammadzadeh et al.72 |
Atheroprotective |
Animal |
Vaisar et al.97 |
Atheroprotective |
— |
Review by den Hartigh96 |
Weight loss or fat mass reduction |
Animal |
(1) Park et al.102 |
|
|
(2) Park et al.103 |
Weight loss or fat mass reduction |
— |
Review by den Hartigh96 |
Conjugated linolenic acid (CLnA) |
Anticancer (colon cancer) |
Animal |
(1) Kohno et al.76 |
|
|
(2) Kohno et al.75 |
Anticancer (ferroptotic cell death) |
Cell lines |
Beatty et al.77 |
Anticancer (leukemia cell death) |
Cell lines |
Liu et al.78 |
Anticancer (adenocarcinoma cell death) |
Cell lines |
Shinohara et al.79 |
Conversion into rumenic acid |
Clinical |
Yuan et al.80 |
Weight loss or fat mass reduction |
Animal |
(1) Chardigny et al.106 |
|
|
(2) Koba et al.107 |
Ximenynic acid |
Anticancer (inhibits the growth of HepG2 cells) |
Cell lines |
Cai et al.81 |
Diabetes (ameliorate insulin resistance) |
Animal |
(1) Gao et al.93 |
|
|
(2) Zhang et al.94 |
18:1n-7 or 18:1(Δ11) |
Biomarker for mouse breast cancer tissues |
Animal |
Ma et al.30 |
Biomarker for human breast cancer tissues |
Human tissues |
(1) Zhang et al.82 |
|
|
(2) Kuo et al.83 |
Biomarker for human breast cancer tissues, human plasma from type-2 diabetes patients |
Human tissues |
Cao et al.29 |
Biomarker for type-2 diabetes |
Human plasma |
(1) Stahlman et al.92 |
|
|
(2) Zhang et al.82 |
Biomarker for nonalcoholic fatty liver disease (NAFLD) |
Animal |
Xu et al.35 |
Sapienic acid or 16:1n-10 |
Biomarker for mouse liver cancer, human liver cancer and lung cancer |
Animal and human tissues |
Vriens et al.86 |
Biomarker for human prostatic adenocarcinoma |
Cell lines |
Ferreri et al.87 |
Biomarker for human breast cancer cells |
Cell lines |
Lin et al.88 |
Biomarker for prostate cancer cell lines and tissues |
Cell lines |
Young et al.91 |
18:1n-10 |
Biomarker for prostate cancer cell lines and tissues |
Cell lines |
Young et al.91 |
Sciadonic acid or 20:3(5Z,11Z,14Z) |
Biomarker for human breast cancer |
Human tissues |
Park et al.90 |
Replace circulating arachidonic acid |
Cell lines |
Tanaka et al.98 |
Eicosanoid modulation |
Cell lines |
(1) Chen et al.99 |
|
|
(2) Chen et al.100 |
|
|
(3) Ells et al.101 |
γ-Linolenic acid or 18:3n-6 |
Inhibit nonalcoholic fatty liver disease (NAFLD) |
Cell lines |
Liang et al.95 |
Branched chain fatty acid (BCFA) |
Essential role in C. elegans development |
C. elegans culture |
(1) Kniazeva et al.115 |
|
|
(2) Zhu et al.116 |
|
|
(3) Zhang et al.117 |
|
|
(4) Vieira et al.118 |
Biomarkers for acne |
Clinical |
Okoro et al.120 |
Biomarkers for meibomian gland dysfunction (MGD) |
Clinical |
(1) Joffre et al.123 |
|
|
(2) Joffre et al.124 |
Intestinal health |
Cell lines |
Yan et al.127 |
Intestinal health |
Animal |
Ran-Ressler et al.128 |
8. Conclusion
Unusual fatty acid isomers can be derived from specific human foods such as dairy, seeds, nuts and seafood. However, research on these fatty acids is relatively limited partly due to their similarity to their common isomeric counterparts and the inability to differentiate them on a de novo basis. The double bond position and methyl branch structure are key features for fatty acids imparting their biological functions. The interplay of advances in lipidomics and lipid functionality has led to their co-development through technological and theoretical iterations. Mass spectrometric methods capable of localizing double bond positions and methyl branch positions provide the necessary support for the functional discovery of unusual fatty acid isomers, which represent mechanism-related biomarkers for cancer, diabetes, nonalcoholic fatty liver disease (NAFLD) and atherosclerosis; modulation of eicosanoids; effects of weight loss and fat mass reduction; the essentiality of BCFAs to the growth of C. elegans; and BCFA's roles in the integrity and health of skin and gut. As methods become more widely adopted, the biological effects of unusual isomeric fatty acids will emerge, with implications for foods and disease states. Adoption of these structure specific or equivalent analytical workflows will enable a new era in fatty acid and glycerolipid biology similar to the adoption of gas chromatography.
Author contributions
Donghao Wang, Zhen Wang and J. Thomas Brenna: conceptualization, design and drafting of the manuscript. Donghao Wang and Tingxiang Yang: generating figures/tables & editing. All authors approved the final version for submission.
Conflicts of interest
The authors declare no conflicts of interest.
References
- J. T. Brenna, M. Plourde, K. D. Stark, P. J. Jones and Y. H. Lin, Best practices for the design, laboratory analysis, and reporting of trials involving fatty acids, Am. J. Clin. Nutr., 2018, 108(2), 211–227 CrossRef PubMed.
- R. G. Jensen, The composition of bovine milk lipids: January 1995 to December 2000, J. Dairy Sci., 2002, 85(2), 295–350 CrossRef CAS PubMed.
- K. W. Lee, H. J. Lee, H. Y. Cho and Y. J. Kim, Role of the conjugated linoleic acid in the prevention of cancer, Crit. Rev. Food Sci. Nutr., 2005, 45(2), 135–144 CrossRef CAS PubMed.
- F. Jia, M. Cui, M. T. Than and M. Han, Developmental Defects of Caenorhabditis elegans Lacking Branched-chain alpha-Ketoacid Dehydrogenase Are Mainly Caused by Monomethyl Branched-Chain Fatty Acid Deficiency, J. Biol. Chem., 2015, 291(6), 2967–2973 CrossRef PubMed.
- D. H. Wang, Z. Wang, K. P. Le, J. R. Cortright, H. G. Park, H. J. Tobias and J. T. Brenna, Potentially High Value Conjugated Linolenic Acids (CLnA) in Melon Seed Waste, J. Agric. Food Chem., 2019, 67(37), 10306–10312 CrossRef CAS PubMed.
- D. H. Wang, Z. Wang, J. R. Cortright, K. P. Le, L. Liu, K. S. D. Kothapalli and J. T. Brenna, Identification of Polymethylene-Interrupted Polyunsaturated Fatty Acids (PMI-PUFA) by Solvent-Mediated Covalent Adduct Chemical Ionization Triple Quadrupole Tandem Mass Spectrometry, Anal. Chem., 2020, 92(12), 8209–8217 CrossRef CAS PubMed.
- D. H. Wang, Z. Wang, R. Chen and J. T. Brenna, Characterization and Semiquantitative Analysis of Novel Ultratrace C10–24 Monounsaturated Fatty Acid in Bovine Milkfat by Solvent-Mediated Covalent Adduct Chemical Ionization (CACI) MS/MS, J. Agric. Food Chem., 2020, 68(28), 7482–7489 CrossRef CAS PubMed.
- C. J. Wolyniak, J. T. Brenna, K. J. Murphy and A. J. Sinclair, Gas chromatography-chemical ionization-mass spectrometric fatty acid analysis of a commercial supercritical carbon dioxide lipid extract from New Zealand green-lipped mussel (Perna canaliculus), Lipids, 2005, 40(4), 355–360 CrossRef CAS PubMed.
- J. T. Brenna, Fatty acid analysis by high resolution gas chromatography and mass spectrometry for clinical and experimental applications, Curr. Opin. Clin. Nutr. Metab. Care, 2013, 16(5), 548–554 CrossRef CAS PubMed.
- J. T. Brenna and K. S. D. Kothapalli, New understandings of the pathway of long-chain polyunsaturated fatty acid biosynthesis, Curr. Opin. Clin. Nutr. Metab. Care, 2022, 25(2), 60–66 CrossRef CAS PubMed.
-
D. H. Wang and J. T. Brenna, Fatty Acids: Structural and Quantitative Analysis, in Mass Spectrometry for Lipidomics: Methods and Applications, ed. M. Holcapek and K. Ekroos, Wiley Online Library, New York, 2023 Search PubMed.
- R. A. Harris, J. C. May, C. A. Stinson, Y. Xia and J. A. McLean, Determining Double Bond Position in Lipids Using Online Ozonolysis Coupled to Liquid Chromatography and Ion Mobility-Mass Spectrometry, Anal. Chem., 2018, 90(3), 1915–1924 CrossRef CAS PubMed.
- D. L. Marshall, H. T. Pham, M. Bhujel, J. S. Chin, J. Y. Yew, K. Mori, T. W. Mitchell and S. J. Blanksby, Sequential Collision- and Ozone-Induced Dissociation Enables Assignment of Relative Acyl Chain Position in Triacylglycerols, Anal. Chem., 2016, 88(5), 2685–2692 CrossRef CAS PubMed.
- C. E. Randolph, C. H. Beveridge, S. Iyer, S. J. Blanksby, S. A. McLuckey and G. Chopra, Identification of Monomethyl Branched-Chain Lipids by a Combination of Liquid Chromatography Tandem Mass Spectrometry and Charge-Switching Chemistries, J. Am. Soc. Mass Spectrom., 2022, 33(11), 2156–2164 CrossRef CAS PubMed.
- C. E. Randolph, S. J. Blanksby and S. A. McLuckey, Toward Complete Structure Elucidation of Glycerophospholipids in the Gas Phase through Charge Inversion Ion/Ion Chemistry, Anal. Chem., 2020, 92(1), 1219–1227 CrossRef CAS PubMed.
- B. Zhang, Y. Wang, B. W. Zhou, J. Cheng, Q. Xu, L. Zhang, T. Q. Sun, J. Zhang and Y. L. Guo, Chloramine-T-Enabled Mass Spectrometric Analysis of C horizontal lineC Isomers of Unsaturated Fatty Acids and Phosphatidylcholines in Human Thyroids, Anal. Chem., 2022, 94(16), 6216–6224 CrossRef CAS PubMed.
- D. H. Wang, H. G. Park, Z. Wang, R. J. S. Lacombe, V. V. Shmanai, A. V. Bekish, K. Schmidt, M. S. Shchepinov and J. T. Brenna, Toward Quantitative Sequencing of Deuteration of Unsaturated Hydrocarbon Chains in Fatty Acids, Anal. Chem., 2021, 93(23), 8238–8247 CrossRef CAS PubMed.
- C. Tyburczy, P. Delmonte, A. R. Fardin-Kia, M. M. Mossoba, J. K. Kramer and J. I. Rader, Profile of trans fatty acids (FAs) including trans polyunsaturated FAs in representative fast food samples, J. Agric. Food Chem., 2012, 60(18), 4567–4577 CrossRef CAS PubMed.
- W. W. Christie, Gas chromatography-mass spectrometry methods for structural analysis of fatty acids, Lipids, 1998, 33(4), 343–353 CrossRef CAS PubMed.
- D. Harvey, Picolinyl esters as derivatives for the structural determination of long chain branched and unsaturated fatty acids, Biomed. Mass Spectrom., 1982, 9(1), 33–38 CrossRef CAS.
- S. Liao and Y. Huang, Preferential formation of mono–dimethyl disulfide adducts for determining double bond positions of poly–unsaturated fatty acids, J. Am. Oil Chem. Soc., 2022, 99(4), 279–288 CrossRef CAS.
- S. Shibamoto, T. Murata and K. Yamamoto, Determination of Double Bond Positions and Geometry of Methyl Linoleate Isomers with Dimethyl Disulfide Adducts by GC/MS, Lipids, 2016, 51(9), 1077–1081 CrossRef CAS PubMed.
- D. H. Wang, Z. Wang, X. Li, S. Martinez, G. James, M. S. Rahman and J. T. Brenna, Unusual polymethylene-interrupted, Delta5 monounsaturated and omega-3 fatty acids in sea urchin (Arbacia punctulata) from the Gulf of Mexico identified by solvent mediated covalent adduct chemical ionization mass spectrometry, Food Chem., 2022, 371, 131131 CrossRef CAS PubMed.
- Z. Wang, D. H. Wang, H. G. Park, H. J. Tobias, K. S. D. Kothapalli and J. T. Brenna, Structural Identification of Monounsaturated Branched Chain Fatty Acid Methyl Esters by Combination of Electron Ionization and Covalent Adduct Chemical Ionization Tandem Mass Spectrometry, Anal. Chem., 2019, 91(23), 15147–15154 CrossRef CAS PubMed.
- C. K. Van Pelt and J. T. Brenna, Acetonitrile chemical ionization tandem mass spectrometry to locate double bonds in polyunsaturated fatty acid methyl esters, Anal. Chem., 1999, 71(10), 1981–1989 CrossRef CAS PubMed.
-
D. H. Wang and J. Thomas Brenna, Fatty Acids: Structural and Quantitative Analysis, in Mass Spectrometry for Lipidomics, 2023, pp. 291–315 Search PubMed.
- B. L. J. Poad, D. L. Marshall, E. Harazim, R. Gupta, V. R. Narreddula, R. S. E. Young, E. Duchoslav, J. L. Campbell, J. A. Broadbent, J. Cvacka, T. W. Mitchell and S. J. Blanksby, Combining Charge-Switch Derivatization with Ozone-Induced Dissociation for Fatty Acid Analysis, J. Am. Soc. Mass Spectrom., 2019, 30(10), 2135–2143 CrossRef CAS PubMed.
- C. Sun, Y. Y. Zhao and J. M. Curtis, A study of the ozonolysis of model lipids by electrospray ionization mass spectrometry, Rapid Commun. Mass Spectrom., 2012, 26(8), 921–930 CrossRef CAS PubMed.
- W. Cao, S. Cheng, J. Yang, J. Feng, W. Zhang, Z. Li, Q. Chen, Y. Xia, Z. Ouyang and X. Ma, Large-scale lipid analysis with C=C location and sn-position isomer resolving power, Nat. Commun., 2020, 11(1), 375 CrossRef CAS PubMed.
- X. Ma, L. Chong, R. Tian, R. Shi, T. Y. Hu, Z. Ouyang and Y. Xia, Identification and quantitation of lipid C=C location isomers: A shotgun lipidomics approach enabled by photochemical reaction, Proc. Natl. Acad. Sci. U. S. A., 2016, 113(10), 2573–2578 CrossRef CAS PubMed.
- X. Xie, J. Zhao, M. Lin, J. L. Zhang and Y. Xia, Profiling of Cholesteryl Esters by Coupling Charge-Tagging Paterno-Buchi Reaction and Liquid Chromatography-Mass Spectrometry, Anal. Chem., 2020, 92(12), 8487–8496 CrossRef CAS PubMed.
- X. Ma and Y. Xia, Pinpointing double bonds in lipids by Paterno-Buchi reactions and mass spectrometry, Angew. Chem., Int. Ed., 2014, 53(10), 2592–2596 CrossRef CAS PubMed.
- P. Esch and S. Heiles, Charging and Charge Switching of Unsaturated Lipids and Apolar Compounds Using Paterno-Buchi Reactions, J. Am. Soc. Mass Spectrom., 2018, 29(10), 1971–1980 CrossRef CAS PubMed.
- R. C. Murphy, T. Okuno, C. A. Johnson and R. M. Barkley, Determination of Double Bond Positions in Polyunsaturated Fatty Acids Using the Photochemical Paterno-Buchi Reaction with Acetone and Tandem Mass Spectrometry, Anal. Chem., 2017, 89(16), 8545–8553 CrossRef CAS PubMed.
- S. L. Xu, B. F. Wu, M. Oresic, Y. Xie, P. Yao, Z. Y. Wu, X. Lv, H. Chen and F. Wei, Double Derivatization Strategy for High-Sensitivity and High-Coverage Localization of Double Bonds in Free Fatty Acids by Mass Spectrometry, Anal. Chem., 2020, 92(9), 6446–6455 CrossRef CAS PubMed.
- L. Chong, R. Tian, R. Shi, Z. Ouyang and Y. Xia, Coupling the Paterno-Buchi (PB) Reaction With Mass Spectrometry to Study Unsaturated Fatty Acids in Mouse Model of Multiple Sclerosis, Front. Chem., 2019, 7, 807 CrossRef CAS PubMed.
- D. H. Wang, D. Vidovic, A. I. McKay, T. Darwish, H. G. Park, S. M. Garza, S. W. Shields, J. S. Brodbelt, Z. Wang, R. J. S. Lacombe, V. V. Shmanai, I. L. Lysenko, A. V. Bekish, K. Schmidt, C. Redfield, J. T. Brenna and M. S. Shchepinov, Quantitative High-Field NMR- and Mass Spectrometry-Based Fatty Acid Sequencing Reveals Internal Structure in Ru-Catalyzed Deuteration of Docosahexaenoic Acid, Anal. Chem., 2022, 94(38), 12971–12980 CrossRef CAS PubMed.
- Y. Feng, B. Chen, Q. Yu and L. Li, Identification of Double Bond Position Isomers in Unsaturated Lipids by m-CPBA Epoxidation and Mass Spectrometry Fragmentation, Anal. Chem., 2019, 91(3), 1791–1795 CrossRef CAS PubMed.
- C. E. Randolph, D. J. Foreman, S. K. Betancourt, S. J. Blanksby and S. A. McLuckey, Gas-Phase Ion/Ion Reactions Involving Tris-Phenanthroline Alkaline Earth Metal Complexes as Charge Inversion Reagents for the Identification of Fatty Acids, Anal. Chem., 2018, 90(21), 12861–12869 CrossRef CAS PubMed.
- C. E. Randolph, D. J. Foreman, S. J. Blanksby and S. A. McLuckey, Generating Fatty Acid Profiles in the Gas Phase: Fatty Acid Identification and Relative Quantitation Using Ion/Ion Charge Inversion Chemistry, Anal. Chem., 2019, 91(14), 9032–9040 CrossRef CAS PubMed.
- C. E. Randolph, S. J. Blanksby and S. A. McLuckey, Enhancing detection and characterization of lipids using charge manipulation in electrospray ionization-tandem mass spectrometry, Chem. Phys. Lipids, 2020, 232, 104970 CrossRef CAS PubMed.
- D. R. Klein and J. S. Brodbelt, Structural Characterization of Phosphatidylcholines Using 193 nm Ultraviolet Photodissociation Mass Spectrometry, Anal. Chem., 2017, 89(3), 1516–1522 CrossRef CAS PubMed.
- V. R. Narreddula, B. I. McKinnon, S. J. P. Marlton, D. L. Marshall, N. R. B. Boase, B. L. J. Poad, A. J. Trevitt, T. W. Mitchell and S. J. Blanksby, Next-generation derivatization reagents optimized for enhanced product ion formation in photodissociation-mass spectrometry of fatty acids, Analyst, 2021, 146(1), 156–169 RSC.
- T. Baba, J. L. Campbell, J. C. Le Blanc and P. R. Baker, Structural identification of triacylglycerol isomers using electron impact excitation of ions from organics (EIEIO), J. Lipid Res., 2016, 57(11), 2015–2027 CrossRef CAS PubMed.
- T. Baba, J. L. Campbell, J. C. Y. Le Blanc and P. R. S. Baker, Distinguishing Cis and Trans Isomers in Intact Complex Lipids Using Electron Impact Excitation of Ions from Organics Mass Spectrometry, Anal. Chem., 2017, 89(14), 7307–7315 CrossRef CAS PubMed.
- J. L. Campbell and T. Baba, Near-complete structural characterization of phosphatidylcholines using electron impact excitation of ions from organics, Anal. Chem., 2015, 87(11), 5837–5845 CrossRef CAS PubMed.
- R. R. Ran-Ressler, S. Bae, P. Lawrence, D. H. Wang and J. T. Brenna, Branched-chain fatty acid content of foods and estimated intake in the USA, Br. J. Nutr., 2014, 112(4), 565–572 CrossRef CAS PubMed.
- D. H. Wang, Y. Yang, Z. Wang, P. Lawrence, R. W. Worobo and J. T. Brenna, High levels of branched chain fatty acids in natto and other Asian fermented foods, Food Chem., 2019, 286, 428–433 CrossRef CAS PubMed.
- T. Kaneda, Iso- and anteiso-fatty acids in bacteria: biosynthesis, function, and taxonomic significance, Microbiol. Rev., 1991, 55(2), 288–302 CrossRef CAS PubMed.
- Z. Wang, H. G. Park, D. H. Wang, R. Kitano, K. S. D. Kothapalli and J. T. Brenna, Fatty acid desaturase 2 (FADS2) but not FADS1 desaturates branched chain and odd chain saturated fatty acids, Biochim. Biophys. Acta, Mol. Cell Biol. Lipids, 2020, 1865(3), 158572 CrossRef CAS PubMed.
- R. R. Ran-Ressler, P. Lawrence and J. T. Brenna, Structural characterization of saturated branched chain fatty acid methyl esters by collisional dissociation of molecular ions generated by electron ionization, J. Lipid Res., 2012, 53(1), 195–203 CrossRef CAS PubMed.
- M. T. G. Hierro, G. Robertson, W. W. Christie and Y. G. Joh, The fatty acid composition of the seeds ofGinkgo biloba, J. Am. Oil Chem. Soc., 1996, 73(5), 575–579 CrossRef CAS.
- J. A. Zirrolli and R. A. Murphy, Low-Energy Tandem Mass Spectrometry of the Molecular Ion Derived from Fatty Acid Methyl Esters: A Novel Method for Analysis of Branched-Chain Fatty Acids, J. Am. Soc. Mass Spectrom., 1993, 4, 223–229 CrossRef CAS PubMed.
- D. H. Wang, Z. Wang, R. Chen, K. S. D. Kothapalli and J. T. Brenna, Very Long-Chain Branched-Chain Fatty Acids in Chia Seeds: Implications for Human Use, J. Agric. Food Chem., 2020, 68(47), 13871–13878 CrossRef CAS PubMed.
- D. H. Wang, Z. Wang and J. T. Brenna, Gas Chromatography Chemical Ionization Mass Spectrometry and Tandem Mass Spectrometry for Identification and Straightforward Quantification of Branched Chain Fatty Acids in Foods, J. Agric. Food Chem., 2020, 68(17), 4973–4980 CrossRef CAS PubMed.
- C. E. Randolph, D. M. Shenault, S. J. Blanksby and S. A. McLuckey, Localization of Carbon-Carbon Double Bond and Cyclopropane Sites in Cardiolipins via Gas-Phase Charge Inversion Reactions, J. Am. Soc. Mass Spectrom., 2021, 32(2), 455–464 CrossRef CAS PubMed.
- K. S. D. Kothapalli, H. G. Park, N. S. L. Kothapalli and J. T. Brenna, FADS2 function at the major cancer hotspot 11q13 locus alters fatty acid metabolism in cancer, Prog. Lipid Res., 2023, 92, 101242 CrossRef CAS PubMed.
- M. V. Liberti and J. W. Locasale, The Warburg Effect: How Does it Benefit Cancer Cells?, Trends Biochem. Sci., 2016, 41(3), 211–218 CrossRef CAS PubMed.
- M. M. McGowan, B. L. Eisenberg, L. D. Lewis, H. M. Froehlich, W. A. Wells, A. Eastman, N. B. Kuemmerle, K. M. Rosenkrantz, R. J. Barth Jr., G. N. Schwartz, Z. Li, T. D. Tosteson, B. B. Beaulieu Jr. and W. B. Kinlaw, A proof of principle clinical trial to determine whether conjugated linoleic acid modulates the lipogenic pathway in human breast cancer tissue, Breast Cancer Res. Treat., 2013, 138(1), 175–183 CrossRef CAS PubMed.
- M. A. Rakib, W. S. Lee, G. S. Kim, J. H. Han, J. O. Kim and Y. L. Ha, Antiproliferative Action of Conjugated Linoleic Acid on Human MCF-7 Breast Cancer Cells Mediated by Enhancement of Gap Junctional Intercellular Communication through Inactivation of NF- kappa B, J. Evidence-Based Complementary Altern. Med., 2013, 2013, 429393 Search PubMed.
- M. Futakuchi, J. L. Cheng, M. Hirose, N. Kimoto, Y. M. Cho, T. Iwata, M. Kasai, S. Tokudome and T. Shirai, Inhibition of conjugated fatty acids derived from safflower or perilla oil of induction and development of mammary tumors in rats induced by 2-amino-1-methyl-6-phenylimidazo[4,5-b]pyridine (PhIP), Cancer Lett., 2002, 178(2), 131–139 CrossRef CAS PubMed.
- J. Bassaganya-Riera, M. Viladomiu, M. Pedragosa, C. De Simone and R. Hontecillas, Immunoregulatory
mechanisms underlying prevention of colitis-associated colorectal cancer by probiotic bacteria, PLoS One, 2012, 7(4), e34676 CrossRef CAS PubMed.
- D. S. Lau and M. C. Archer, The 10t,12c isomer of conjugated linoleic acid inhibits fatty acid synthase expression and enzyme activity in human breast, colon, and prostate cancer cells, Nutr. Cancer, 2010, 62(1), 116–121 CrossRef CAS PubMed.
- S. M. Soel, O. S. Choi, M. H. Bang, J. H. Yoon Park and W. K. Kim, Influence of conjugated linoleic acid isomers on the metastasis of colon cancer cells in vitro and in vivo, J. Nutr. Biochem., 2007, 18(10), 650–657 CrossRef CAS PubMed.
- R. Shiraishi, R. Iwakiri, T. Fujise, T. Kuroki, T. Kakimoto, T. Takashima, Y. Sakata, S. Tsunada, Y. Nakashima, T. Yanagita and K. Fujimoto, Conjugated linoleic acid suppresses colon carcinogenesis in azoxymethane-pretreated rats with long-term feeding of diet containing beef tallow, J. Gastroenterol., 2010, 45(6), 625–635 CrossRef CAS PubMed.
- B. Q. Chen, Y. B. Xue, J. R. Liu, Y. M. Yang, Y. M. Zheng, X. L. Wang and R. H. Liu, Inhibition of conjugated linoleic acid on mouse forestomach neoplasia induced by benzo (a) pyrene and chemopreventive mechanisms, World J. Gastroenterol., 2003, 9(1), 44–49 CrossRef CAS PubMed.
- Y. L. Ha, J. Storkson and M. W. Pariza, Inhibition of benzo(a)pyrene-induced mouse forestomach neoplasia by conjugated dienoic derivatives of linoleic acid, Cancer Res., 1990, 50(4), 1097–1101 CAS.
- A. Cesano, S. Visonneau, J. A. Scimeca, D. Kritchevsky and D. Santoli, Opposite effects of linoleic acid and conjugated linoleic acid on human prostatic cancer in SCID mice, Anticancer Res., 1998, 18(3A), 1429–1434 CAS.
- E. J. Kim, H. K. Shin, J. S. Cho, S. K. Lee, M. H. Won, J. W. Kim and J. H. Park, trans-10,cis-12 conjugated linoleic acid inhibits the G1-S cell cycle progression in DU145 human prostate carcinoma cells, J. Med. Food, 2006, 9(3), 293–299 CrossRef CAS PubMed.
- H. J. Song, A. A. Sneddon, S. D. Heys and K. W. Wahle, Induction of apoptosis and inhibition of NF-kappaB activation in human prostate cancer cells by the cis-9, trans-11 but not the trans-10, cis-12 isomer of conjugated linoleic acid, Prostate, 2006, 66(8), 839–846 CrossRef CAS PubMed.
- M. Yamasaki, E. Nishida, S. Nou, H. Tachibana and K. Yamada, Cytotoxity of the trans10,cis12 isomer of conjugated linoleic acid on rat hepatoma and its modulation by other fatty acids, tocopherol, and tocotrienol, In Vitro Cell. Dev. Biol.: Anim., 2005, 41(7), 239–244 CrossRef CAS PubMed.
- M. Mohammadzadeh, E. Faramarzi, R. Mahdavi, B. Nasirimotlagh and M. Asghari Jafarabadi, Effect of conjugated linoleic acid supplementation on inflammatory factors and matrix metalloproteinase enzymes in rectal cancer patients undergoing chemoradiotherapy, Integr. Cancer Ther., 2013, 12(6), 496–502 CrossRef CAS PubMed.
- S. C. Larsson, L. Bergkvist and A. Wolk, High-fat dairy food and conjugated linoleic acid intakes in relation to colorectal cancer incidence in the Swedish Mammography Cohort, Am. J. Clin. Nutr., 2005, 82(4), 894–900 CrossRef CAS PubMed.
- L. E. Voorrips, H. A. Brants, A. F. Kardinaal, G. J. Hiddink, P. A. van den Brandt and R. A. Goldbohm, Intake of conjugated linoleic acid, fat, and other fatty acids in relation to postmenopausal breast cancer: the Netherlands Cohort Study on Diet and Cancer, Am. J. Clin. Nutr., 2002, 76(4), 873–882 CrossRef CAS PubMed.
- H. Kohno, Y. Yasui, R. Suzuki, M. Hosokawa, K. Miyashita and T. Tanaka, Dietary seed oil rich in conjugated linolenic acid from bitter melon inhibits azoxymethane-induced rat colon carcinogenesis through elevation of colonic PPARgamma expression and alteration of lipid composition, Int. J. Cancer, 2004, 110(6), 896–901 CrossRef CAS PubMed.
- H. Kohno, R. Suzuki, Y. Yasui, M. Hosokawa, K. Miyashita and T. Tanaka, Pomegranate seed oil rich in conjugated linolenic acid suppresses chemically induced colon carcinogenesis in rats, Cancer Sci., 2004, 95(6), 481–486 CrossRef CAS PubMed.
- A. Beatty, T. Singh, Y. Y. Tyurina, V. A. Tyurin, S. Samovich, E. Nicolas, K. Maslar, Y. Zhou, K. Q. Cai, Y. Tan, S. Doll, M. Conrad, A. Subramanian, H. Bayir, V. E. Kagan, U. Rennefahrt and J. R. Peterson, Ferroptotic cell death triggered by conjugated linolenic acids is mediated by ACSL1, Nat. Commun., 2021, 12(1), 2244 CrossRef CAS PubMed.
- W. N. Liu and K. N. Leung, Apoptosis- and differentiation-inducing activities of jacaric acid, a conjugated linolenic acid isomer, on human eosinophilic leukemia EoL-1 cells, Oncol. Rep., 2014, 32(5), 1881–1888 CrossRef CAS PubMed.
- N. Shinohara, T. Tsuduki, J. Ito, T. Honma, R. Kijima, S. Sugawara, T. Arai, M. Yamasaki, A. Ikezaki, M. Yokoyama, K. Nishiyama, K. Nakagawa, T. Miyazawa and I. Ikeda, Jacaric acid, a linolenic acid isomer with a conjugated triene system, has a strong antitumor effect in vitro and in vivo, Biochim. Biophys. Acta, 2012, 1821(7), 980–988 CrossRef CAS PubMed.
- G. Yuan, A. J. Sinclair, C. Xu and D. Li, Incorporation and metabolism of punicic acid in healthy young humans, Mol. Nutr. Food Res., 2009, 53(10), 1336–1342 CrossRef CAS PubMed.
- F. Cai, J. Li, Y. Liu, Z. Zhang, D. S. Hettiarachchi and D. Li, Effect of ximenynic acid on cell cycle arrest and apoptosis and COX-1 in HepG2 cells, Mol. Med. Rep., 2016, 14(6), 5667–5676 CrossRef CAS PubMed.
- W. Zhang, D. Zhang, Q. Chen, J. Wu, Z. Ouyang and Y. Xia, Online photochemical derivatization enables comprehensive mass spectrometric analysis of unsaturated phospholipid isomers, Nat. Commun., 2019, 10(1), 79 CrossRef CAS PubMed.
- T. H. Kuo, H. H. Chung, H. Y. Chang, C. W. Lin, M. Y. Wang, T. L. Shen and C. C. Hsu, Deep Lipidomics and Molecular Imaging of Unsaturated Lipid Isomers: A Universal Strategy Initiated by mCPBA Epoxidation, Anal. Chem., 2019, 91(18), 11905–11915 CrossRef CAS PubMed.
- H. G. Park, K. S. D. Kothapalli, W. J. Park, C. DeAllie, L. Liu, A. Liang, P. Lawrence and J. T. Brenna, Palmitic acid (16:0) competes with omega-6 linoleic and omega-3 a-linolenic acids for FADS2 mediated Delta6-desaturation, Biochim. Biophys. Acta, 2016, 1861(2), 91–97 CrossRef CAS PubMed.
- M. T. Snaebjornsson and A. Schulze, Tumours use a metabolic twist to make lipids, Nature, 2019, 566(7744), 333–334 CrossRef CAS PubMed.
- K. Vriens, S. Christen, S. Parik, D. Broekaert, K. Yoshinaga, A. Talebi, J. Dehairs, C. Escalona-Noguero, R. Schmieder, T. Cornfield, C. Charlton, L. Romero-Perez, M. Rossi, G. Rinaldi, M. F. Orth, R. Boon, A. Kerstens, S. Y. Kwan, B. Faubert, A. Mendez-Lucas, C. C. Kopitz, T. Chen, J. Fernandez-Garcia, J. A. G. Duarte, A. A. Schmitz, P. Steigemann, M. Najimi, A. Hagebarth, J. A. Van Ginderachter, E. Sokal, N. Gotoh, K. K. Wong, C. Verfaillie, R. Derua, S. Munck, M. Yuneva, L. Beretta, R. J. DeBerardinis, J. V. Swinnen, L. Hodson, D. Cassiman, C. Verslype, S. Christian, S. Grunewald, T. G. P. Grunewald and S. M. Fendt, Evidence for an alternative fatty acid desaturation pathway increasing cancer plasticity, Nature, 2019, 566(7744), 403–406 CrossRef PubMed.
- C. Ferreri, A. Sansone, S. Buratta, L. Urbanelli, E. Costanzi, C. Emiliani and C. Chatgilialoglu, The n-10 Fatty Acids Family in the Lipidome of Human Prostatic Adenocarcinoma Cell Membranes and Extracellular Vesicles, Cancers, 2020, 12(4), 900 CrossRef CAS PubMed.
- Q. Lin, P. Li, M. Fang, D. Zhang and Y. Xia, Deep Profiling of Aminophospholipids Reveals a Dysregulated Desaturation Pattern in Breast Cancer Cell Lines, Anal. Chem., 2022, 94(2), 820–828 CrossRef CAS PubMed.
- W. J. Park, K. S. Kothapalli, P. Lawrence and J. T. Brenna, FADS2 function loss at the cancer hotspot 11q13 locus diverts lipid signaling precursor synthesis to unusual eicosanoid fatty acids, PLoS One, 2011, 6(11), e28186 CrossRef CAS PubMed.
- H. G. Park, J. Y. Zhang, C. Foster, D. Sudilovsky, D. A. Schwed, J. Mecenas, S. Devapatla, P. Lawrence, K. S. D. Kothapalli and J. T. Brenna, A rare eicosanoid precursor analogue, sciadonic acid (5Z,11Z,14Z-20:3), detected in vivo in hormone positive breast cancer tissue, Prostaglandins, Leukotrienes Essent. Fatty Acids, 2018, 134, 1–6 CrossRef CAS PubMed.
- R. S. E. Young, A. P. Bowman, E. D. Williams, K. D. Tousignant, C. L. Bidgood, V. R. Narreddula, R. Gupta, D. L. Marshall, B. L. J. Poad, C. C. Nelson, S. R. Ellis, R. M. A. Heeren, M. C. Sadowski and S. J. Blanksby, Apocryphal FADS2 activity promotes fatty acid diversification in cancer, Cell Rep., 2021, 34(6), 108738 CrossRef CAS PubMed.
- M. Stahlman, H. T. Pham, M. Adiels, T. W. Mitchell, S. J. Blanksby, B. Fagerberg, K. Ekroos and J. Boren, Clinical dyslipidaemia is associated with changes in the lipid composition and inflammatory properties of apolipoprotein-B-containing lipoproteins from women with type 2 diabetes, Diabetologia, 2012, 55(4), 1156–1166 CrossRef CAS PubMed.
- X. Gao, H. Zhang, K. Li, Y. Shi, X. Guo, L. Wang and D. Li, Sandalwood seed oil improves insulin sensitivity in high-fat/high-sucrose diet-fed rats associated with altered intestinal microbiota and its metabolites, Food Funct., 2021, 12(20), 9739–9749 RSC.
- H. Zhang, X. Gao, K. Li, Y. Liu, D. S. Hettiarachichi, B. Sunderland and D. Li, Sandalwood seed oil ameliorates hepatic insulin resistance by regulating the JNK/NF-kappaB inflammatory and PI3K/AKT insulin signaling pathways, Food Funct., 2021, 12(5), 2312–2322 RSC.
- Y. Liang, Z. Zhang, J. Tu, Z. Wang, X. Gao, K. Deng, M. A. El-Samahy, P. You, Y. Fan and F. Wang, gamma-Linolenic Acid Prevents Lipid Metabolism Disorder in Palmitic Acid-Treated Alpha Mouse Liver-12 Cells by Balancing Autophagy and Apoptosis via the LKB1-AMPK-mTOR Pathway, J. Agric. Food Chem., 2021, 69(29), 8257–8267 CrossRef CAS PubMed.
- L. J. den Hartigh, Conjugated Linoleic Acid Effects on Cancer, Obesity, and Atherosclerosis: A Review of Pre-Clinical and Human Trials with Current Perspectives, Nutrients, 2019, 11(2), 370 CrossRef CAS PubMed.
- T. Vaisar, S. Wang, M. Omer, A. D. Irwin, C. Storey, C. Tang and L. J. den Hartigh, 10,12-Conjugated linoleic acid supplementation improves HDL composition and function in mice, J. Lipid Res., 2022, 63(8), 100241 CrossRef CAS PubMed.
- T. Tanaka, J. Morishige, T. Takimoto, Y. Takai and K. Satouchi, Metabolic characterization of sciadonic acid (5c,11c,14c-eicosatrienoic acid) as an effective substitute for arachidonate of phosphatidylinositol, Eur. J. Biochem., 2001, 268(18), 4928–4939 CrossRef CAS PubMed.
- S. J. Chen, W. C. Huang, T. T. Yang, J. H. Lu and L. T. Chuang, Incorporation of sciadonic acid into cellular phospholipids reduces pro-inflammatory
mediators in murine macrophages through NF-kappaB and MAPK signaling pathways, Food Chem. Toxicol., 2012, 50(10), 3687–3695 CrossRef CAS PubMed.
- S. J. Chen, L. T. Chuang, J. S. Liao, W. C. Huang and H. H. Lin, Phospholipid Incorporation of Non-Methylene-Interrupted Fatty Acids (NMIFA) in Murine Microglial BV-2 Cells Reduces Pro-Inflammatory Mediator Production, Inflammation, 2015, 38(6), 2133–2145 CrossRef CAS PubMed.
- R. Ells, J. L. Kock, J. Albertyn, A. Hugo and C. H. Pohl, Sciadonic acid modulates prostaglandin E2 production by epithelial cells during infection with C. albicans and C. dubliniensis, Prostaglandins Other Lipid Mediators, 2012, 97(1–2), 66–71 CrossRef CAS PubMed.
- Y. Park, K. J. Albright, W. Liu, J. M. Storkson, M. E. Cook and M. W. Pariza, Effect of conjugated linoleic acid on body composition in mice, Lipids, 1997, 32(8), 853–858 CrossRef CAS PubMed.
- Y. Park, J. M. Storkson, K. J. Albright, W. Liu and M. W. Pariza, Evidence that the trans-10,cis-12 isomer of conjugated linoleic acid induces body composition changes in mice, Lipids, 1999, 34(3), 235–241 CrossRef CAS PubMed.
- Y. Park, J. M. Storkson, W. Liu, K. J. Albright, M. E. Cook and M. W. Pariza, Structure-activity relationship of conjugated linoleic acid and its cognates in inhibiting heparin-releasable lipoprotein lipase and glycerol release from fully differentiated 3T3-L1 adipocytes, J. Nutr. Biochem., 2004, 15(9), 561–568 CrossRef CAS PubMed.
- G. Fuke and J. L. Nornberg, Systematic evaluation on the effectiveness of conjugated linoleic acid in human health, Crit. Rev. Food Sci. Nutr., 2017, 57(1), 1–7 CrossRef CAS PubMed.
- J. M. Chardigny, O. Hasselwander, M. Genty, K. Kraemer, A. Ptock and J. L. Sebedio, Effect of conjugated FA on feed intake, body composition, and liver FA in mice, Lipids, 2003, 38(9), 895–902 CrossRef CAS PubMed.
- K. Koba, A. Akahoshi, M. Yamasaki, K. Tanaka, K. Yamada, T. Iwata, T. Kamegai, K. Tsutsumi and M. Sugano, Dietary conjugated linolenic acid in relation to CLA differently modifies body fat mass and serum and liver lipid levels in rats, Lipids, 2002, 37(4), 343–350 CrossRef CAS PubMed.
- J. Miranda, A. Fernández-Quintela, I. Churruca, J. Ayo, C. García-Marzo, R. Dentin and M. P. Portillo, The presence of the trans-10, cis-12 sequence does not have a body fat-lowering effect on jacaric acid, a conjugated linolenic acid isomer, Food Chem., 2011, 129(1), 21–27 CrossRef CAS.
- V. M. Taormina, A. L. Unger, M. R. Schiksnis, M. Torres-Gonzalez and J. Kraft, Branched-Chain Fatty Acids-An Underexplored Class of Dairy-Derived Fatty Acids, Nutrients, 2020, 12(9), 2875 CrossRef CAS PubMed.
- G. Dąbrowski and I. Konopka, Update on food sources and biological activity of odd-chain, branched and cyclic fatty acids––A review, Trends Food Sci. Technol., 2022, 119, 514–529 CrossRef.
- P. Gozdzik, F. Magkos, T. Sledzinski and A. Mika, Monomethyl branched-chain fatty acids: Health effects and biological mechanisms, Prog. Lipid Res., 2023, 90, 101226 CrossRef CAS PubMed.
- N. A. Yehia, K. Z. H. Lai, Z. Semnani-Azad, S. Blanco Mejia, R. P. Bazinet, J. L. Beaudry and A. J. Hanley, Association of branched chain fatty acids with cardiometabolic disorders in humans: a systematic review, Nutr. Rev., 2023, 81(2), 180–190 CrossRef PubMed.
- H. Lu, Z. Wang, B. Cao, F. Cong, X. Wang and W. Wei, Dietary sources of branched-chain fatty acids and their biosynthesis, distribution, and nutritional properties, Food Chem., 2023, 431, 137158 CrossRef PubMed.
- B. Chakravarty, The evolving role of the Caenorhabditis elegans model as a tool to advance studies in nutrition and health, Nutr. Res., 2022, 106, 47–59 CrossRef CAS PubMed.
- M. Kniazeva, Q. T. Crawford, M. Seiber, C. Y. Wang and M. Han, Monomethyl branched-chain fatty acids play an essential role in Caenorhabditis elegans development, PLos Biol., 2004, 2(9), E257 CrossRef PubMed.
- M. Zhu, F. Teng, N. Li, L. Zhang, S. Zhang, F. Xu, J. Shao, H. Sun and H. Zhu, Monomethyl branched-chain fatty acid mediates amino acid sensing upstream of mTORC1, Dev. Cell, 2021, 56(22), 3171 CrossRef CAS PubMed.
- J. Zhang, Y. Hu, Y. Wang, L. Fu, X. Xu, C. Li, J. Xu, C. Li, L. Zhang, R. Yang, X. Jiang, Y. Wu, P. Liu, X. Zou and B. Liang, mmBCFA C17iso ensures endoplasmic reticulum integrity for lipid droplet growth, J. Cell Biol., 2021, 220(11), e202102122 CrossRef CAS PubMed.
- A. F. C. Vieira, M. A. Xatse, H. Tifeki, C. Diot, A. J. M. Walhout and C. P. Olsen, Monomethyl branched-chain fatty acids are critical for Caenorhabitis elegans survival in elevated glucose conditions, J. Biol. Chem., 2022, 298(2), 101444 CrossRef CAS PubMed.
- N. Nicolaides, Skin Lipids: Their Biochemical Uniqueness: Unlike internal organs, the skin biosynthesizes and excretes unusual fat soluble substances, Science, 1974, 186(4158), 19–26 CrossRef CAS PubMed.
- O. E. Okoro, A. Adenle, M. Ludovici, M. Truglio, F. Marini and E. Camera, Lipidomics of facial sebum in the comparison between acne and non-acne adolescents with dark skin, Sci. Rep., 2021, 11(1), 16591 CrossRef CAS PubMed.
- A. Yamamoto, S. Serizawa, M. Ito and Y. Sato, Effect of aging on sebaceous gland activity and on the fatty acid composition of wax esters, J. Invest. Dermatol., 1987, 89(5), 507–512 CrossRef CAS PubMed.
- S. Briganti, M. Truglio, A. Angiolillo, S. Lombardo, D. Leccese, E. Camera, M. Picardo and A. Di Costanzo, Application of Sebum Lipidomics to Biomarkers Discovery in Neurodegenerative Diseases, Metabolites, 2021, 11(12), 819 CrossRef CAS PubMed.
- C. Joffre, M. Souchier, S. Gregoire, S. Viau, L. Bretillon, N. Acar, A. M. Bron and C. Creuzot-Garcher, Differences in meibomian fatty acid composition in patients with meibomian gland dysfunction and aqueous-deficient dry eye, Br. J. Ophthalmol., 2008, 92(1), 116–119 CrossRef CAS PubMed.
- C. Joffre, M. Souchier, L. Leclere, B. Buteau, S. Gregoire, G. Lizard, T. Montange, N. Acar, A. Bron, C. Creuzot-Garcher, Y. Diebold and L. Bretillon, Branched-chain fatty acids, increased in tears of blepharitis patients, are not toxic for conjunctival cells, Br. J. Ophthalmol., 2009, 93(10), 1391–1395 CrossRef CAS PubMed.
- K. Nishijima, M. Yoneda, T. Hirai, K. Takakuwa and T. Enomoto, Biology of the vernix caseosa: A review, J. Obstet. Gynaecol. Res., 2019, 45(11), 2145–2149 CrossRef PubMed.
- R. R. Ran-Ressler, S. Devapatla, P. Lawrence and J. T. Brenna, Branched chain fatty acids are constituents of the normal healthy newborn gastrointestinal tract, Pediatr. Res., 2008, 64(6), 605–609 CrossRef CAS PubMed.
- Y. Yan, Z. Wang, D. Wang, P. Lawrence, X. Wang, K. S. Kothapalli, J. Greenwald, R. Liu, H. G. Park and J. T. Brenna, BCFA-enriched vernix-monoacylglycerol reduces LPS-induced inflammatory markers in human enterocytes in vitro, Pediatr. Res., 2018, 83(4), 874–879 CrossRef CAS PubMed.
- R. R. Ran-Ressler, L. Khailova, K. M. Arganbright, C. K. Adkins-Rieck, Z. E. Jouni, O. Koren, R. E. Ley, J. T. Brenna and B. Dvorak, Branched chain fatty acids reduce the incidence of necrotizing enterocolitis and alter gastrointestinal microbial ecology in a neonatal rat model, PLoS One, 2011, 6(12), e29032 CrossRef CAS PubMed.
|
This journal is © The Royal Society of Chemistry 2024 |