DOI:
10.1039/D3SC05121H
(Edge Article)
Chem. Sci., 2023,
14, 14092-14099
1,2-Disubstituted bicyclo[2.1.1]hexanes as saturated bioisosteres of ortho-substituted benzene†
Received
28th September 2023
, Accepted 25th October 2023
First published on 27th October 2023
Abstract
Bicyclo[2.1.1]hexanes have been synthesized, characterized, and biologically validated as saturated bioisosteres of the ortho-substituted benzene ring. The incorporation of the 1,2-disubstituted bicyclo[2.1.1]hexane core into the structure of fungicides boscalid (BASF), bixafen (Bayer CS), and fluxapyroxad (BASF) gave saturated patent-free analogs with high antifungal activity.
Introduction
The benzene ring is a basic structural unit in chemistry, and we learn about it in school. It is the most popular ring in natural products1 and bioactive compounds.2,3
The ortho-substituted benzene ring, in particular, is found in the structure of more than three hundred drugs and agrochemicals (Fig. 1).4,5 For example, the well-known drug aspirin contains an ortho-substituted benzene ring. Recently, we discovered that 1,5-disubstituted bicyclo[2.1.1]hexanes and their oxa-containing analogs can mimic the ortho-substituted benzene ring in bioactive compounds (Fig. 1).6 These scaffolds were synthesized as a mixture of two diastereomers. Some of them were subsequently separated by crystallization or column chromatography. In many cases, however, the separation of diastereomers failed, and the desired products were not obtained.
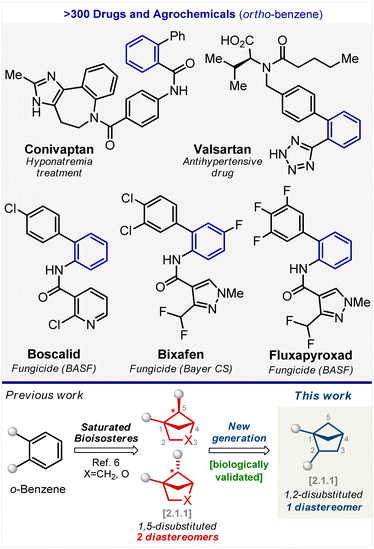 |
| Fig. 1 Bicyclo[2.1.1]hexanes as saturated bioisosteres of the ortho-substituted benzene ring: state of the art. | |
Pleasingly, these studies were well received by the scientific community, and the groups of Glorius,7 Brown,8 Procter,9 Li,10 Wang,11 and Studer12 subsequently developed alternative approaches to di- and poly-substituted bicyclo[2.1.1]hexanes based on the functionalization of bicyclo[1.1.0]butanes.13–15
In this work, we have synthesized, characterized, and biologically validated 1,2-disubstituted bicyclo[2.1.1]hexanes as a new generation of saturated bioisosteres of ortho-substituted benzenes. These structural cores exist as single diastereomers. Bicyclo[2.1.1]hexanes were incorporated into the structure of fungicides boscalid (BASF), bixafen (Bayer CS), and fluxapyroxad (BASF) to provide saturated patent-free analogs with high antifungal activity.
Results and discussion
Design
Benzene (C6H6; M.W. = 78) is an aromatic molecule. Among the conformationally rigid bi(poly)cyclic saturated scaffolds, – bicyclo[1.1.1]pentane,16 bicyclo[3.1.1]heptane,17 cubane,18 and bicyclo[2.1.1]hexane (Fig. 2), – only the latter has the same number of carbon atoms and a similar molecular weight (C6H10; M.W. = 82; Fig. 2b).19,20 Three types of substitution patterns in bicyclo[2.1.1]pentane can potentially mimic the ortho-benzene ring geometry: 1,2-, 1,5-, and 2.3- (Fig. 2b). While two latter cores exist as two diastereomers, the 1,2-disubstituted bicyclo[2.1.1]pentanes exist as only one diastereomer (Fig. 2). Moreover, this scaffold was recently proposed to mimic the ortho-substituted benzene ring; however, biological validation of this hypothesis was not made.9 In this work, we report a practical approach to 1,2-disubstituted bicyclo[2.1.1]hexanes and show with biological experiments that this core indeed can act as a bioisostere of the ortho-substituted benzene ring in bioactive compounds.
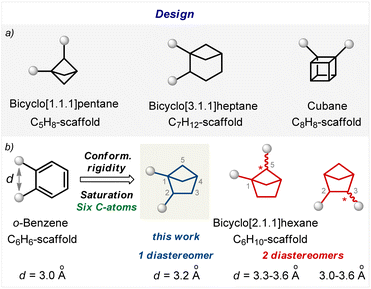 |
| Fig. 2 Design of saturated bioisosteres of the ortho-substituted benzenes. (a) Disubstituted bicyclo[1.1.1]pentanes, bicyclo[3.1.1]heptanes, and cubanes. (b) Disubstituted bicyclo[2.1.1]hexanes. | |
Synthesis
Despite numerous studies on the topic,7–15 we needed a practical approach to bicyclo[2.1.1]hexanes with only two substituents (two exit vectors) at the 1- and 2-positions of the core (Scheme 1) without additional (poly)substitution at other positions. Moreover, one substituent should be (hetero)aromatic, and another one should be the carboxylic group, which is needed for the subsequent modifications of the core via amide coupling.21,22
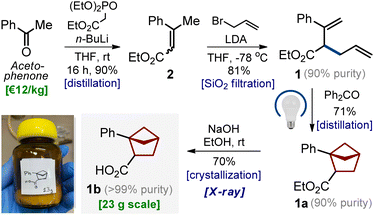 |
| Scheme 1 Gram-scale synthesis of bicyclo[2.1.1]hexane 1b from acetophenone. | |
In light of our previous experience,6 we wondered if diene 1 (easily obtained from acetophenone, please see Scheme 1) would undergo an intramolecular photocycloaddition into the desired bicyclo[2.1.1]hexane core. Direct irradiation of diene 1 in acetonitrile at different wavelengths gave only traces of products (entries 1–4, Table 1). Irradiation with a broad wavelength mercury lamp gave the target product in 35% yield along with the formation of unidentified side products (entry 5). We also tried available organic ketones for the triplet sensitization of the styrene moiety. Cleaner formation of the desired bicyclo[2.1.1]hexane 1a was observed (entries 6–10). The best yield of 76% was obtained with benzophenone (entry 8), whereas thioxanthone also worked well (entry 7). Among all tested solvents (entries 11–14), the best result was obtained in acetonitrile. Without irradiation, the reaction did not take place (entry 15).
Table 1 Optimization of the synthesis of bicyclo[2.1.1]hexane 1a
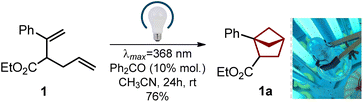
|
Entry |
Conditions |
Yielda,b (%) |
100 mmol scale.
1H NMR yield (CH2Br2 as an internal standard).
Isolated yield after column chromatography. See the ESI for details. n.r.: no reaction.
|
1 |
450 nm, CH3CN |
n.r. |
2 |
368 nm, CH3CN |
<10 |
3 |
313 nm, CH3CN |
<10 |
4 |
254 nm, CH3CN |
<20 |
5 |
Broad wavelength Hg lamp, CH3CN |
35 |
6 |
368 nm, CH3CN, acetophenone |
43 |
7 |
368 nm, CH3CN, thioxanthone |
54 |
8 |
368 nm, CH3CN, benzophenone |
(82)76c |
9 |
368 nm, CH3CN, (p-NO2C6H4)2CO |
31 |
10 |
368 nm, CH3CN, (p-Me0C6H4)2CO |
62 |
11 |
368 nm, CH2Cl2 Ph2CO |
47 |
12 |
368 nm, Me2CO, Ph2CO |
45 |
13 |
368 nm, PhMe, Ph2CO |
31 |
14 |
368 nm, EtOAc, Ph2CO |
43 |
15 |
In the dark, rt |
n.r. |
Scalable synthesis
The entire optimized synthetic protocol is shown in Scheme 1. It was important for us to elaborate on a modular method that would provide bicyclo[2.1.1]hexanes employing only available and inexpensive starting materials. The synthesis started from acetophenone. The Horner–Wadsworth–Emmons reaction of acetophenone smoothly gave alkene 2 in 90% yield after distillation. Treatment of the latter with LDA in THF at −78 °C followed by the addition of allyl bromide gave diene 1 in 81% yield (ca. 90% purity). The compound contained ca. 10% of the isomeric diene, as the undesired alkylation at the methyl group also took place (please see the ESI,† p. S5–S6). An analytically pure sample of diene 1 was obtained by purification with HPLC. In the next step, however, we used the crude material. An intramolecular photocycloaddition of diene 1 proceeded smoothly on scale to provide the desired bicyclo[2.1.1]hexane 1a in 71% yield (ca. 90% purity) after distillation. Saponification of the ester group in 1a followed by crystallization from hexane-tBuOMe gave pure carboxylic acid 1b as a white crystalline solid in 70% yield. The structure of compound 1b was confirmed by X-ray crystallographic analysis (Scheme 2).23
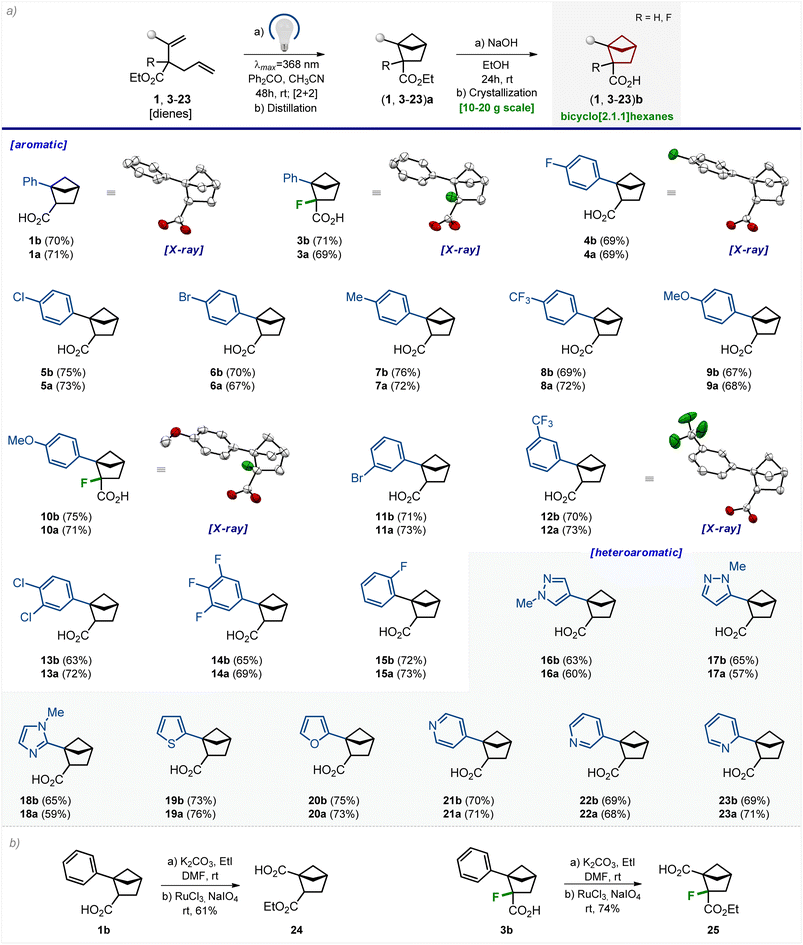 |
| Scheme 2 (a) Scope of the reaction. X-ray crystal structure of compounds 1b, 3b, 4b, 10b, and 12b (carbon – white, oxygen – red, and fluorine – green). Hydrogen and chlorine atoms are omitted for clarity. Ellipsoids are shown at a 50% probability level. (b) Synthesis of linkers 24 and 25. | |
It is important to note that following this optimized sequence, we easily synthesized 23 g of product 1b in one run. No column chromatography was involved at any step.
Scope
We studied the scope of the developed method. The photochemical reaction tolerated various substituents at the aromatic core (Scheme 2). Among them were an alkyl group (7); fluorine (4, 14, and 15), chlorine (5 and 13) and bromine atoms (6 and 11); methoxy (9 and 10) and trifluoromethyl groups (8 and 12). All three substitution patterns of the benzene ring, – para (4–10), meta (11–14), and ortho (15), – gave the corresponding bicyclo[2.1.1]hexanes 4a–15a in 67–73% yield. The photocycloaddition was also compatible with the fluorine atom directly attached to the diene structure (3 and 10). Various medicinal chemistry-relevant heterocycles, such as pyrazole (16 and 17), imidazole (18), thiophene (19), furane (20), and pyridine (21–23), provided the desired bicyclo[2.1.1]hexanes 16a–23a in 57–76% yield. All products 3a–23a were purified by distillation. Saponification of the ester group in 3a–23a followed by crystallization gave solid carboxylic acids 3b–23b in 63–76% yield.
All final products were synthesized in gram quantities. The structures of bicyclo[2.1.1]hexanes 1b, 3b, 4b, 10b, and 12b were confirmed by X-ray crystallographic analysis (Scheme 2).23
Modifications
Compounds 1b and 3b–23b possess one functional group (–CO2H). We aimed to perform some representative modifications of these bicyclo[2.1.1]hexanes to obtain linkers for medicinal chemistry – compounds with two functional groups.
Esterification of carboxylic acid 1b, followed by oxidation of the phenyl group with NaIO4/RuCl3 gave linker 24 (Scheme 2). The fluorine-substituted linker 25 was synthesized analogously from compound 3b. Both linkers 24 and 25 provide a way to synthesize various 1,2-disubstituted bicyclo[2.1.1]hexanes by standard stepwise modifications of carboxylic groups (amide synthesis, heterocyclizations, radical couplings, etc.).24
Crystallographic analysis
Next, we compared the geometric parameters of 1,2-disubstituted bicyclo[2.1.1]hexanes with those of the ortho-substituted benzene ring. For this, we employed the exit vector plot tool. In this method, two substituents on the scaffold were simulated by two exit vectors n1 and n2 (Fig. 3). The relative spatial arrangement of the vectors is described by four geometric parameters: the distance between C-atoms r, the plane angles φ1 (between vectors n1 and C–C) and φ2 (between vectors n2 and C–C), and the dihedral angle θ defined by vectors n1, C–C and n2. An additional representative parameter – the distance d between two carbon substituents (Fig. 3) – was also measured.
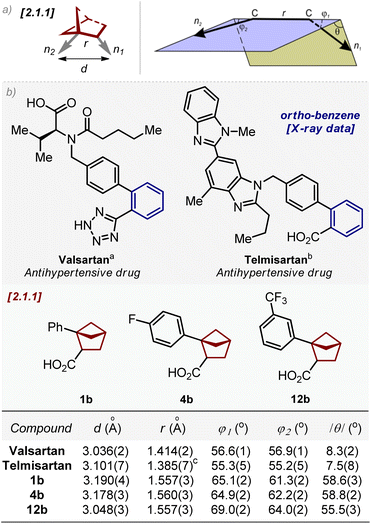 |
| Fig. 3 (a) Definition of vectors n1 and n2, and geometric parameters d, r, φ1, φ2 and θ. 1,2-Disubstituted bicyclo[2.1.1]hexane is shown as an example. (b) Geometric parameters d, r, φ1, φ2, and |θ| for ortho-substituted benzenes (valsartan and telmisartan); and bicyclo[2.1.1]hexanes 1b, 4b, and 12b. aData are taken from ref. 25a. bData are taken from ref. 25b. cTwo molecules of telmisartan are present in the crystalline lattice with r = 1.385(7)Å and 1.395(7)Å (ref. 25b). | |
We calculated the values of d, r, φ1, φ2, and /θ/of 1,2-disubstituted bicyclo[2.1.1]hexanes from the X-ray data of compounds 1b, 4b, and 12b.23
The corresponding parameters for ortho-substituted benzene were obtained from the reported crystal data of two antihypertensive drugs – valsartan and telmisartan (Fig. 3).25 Analysis of these data revealed that the geometric properties of 1,2-disubstituted bicyclo[2.1.1]hexanes in general were similar to those of ortho-substituted benzene. In particular, the distance d in bicyclo[2.1.1]hexanes was only 0.1 Å longer than that in the ortho-benzene ring: 3.05–3.19 Å (1b, 4b, and 12b) vs. 3.04–3.10 Å (ortho-benzene).
The distance r in bicyclo[2.1.1]hexane was ca. 0.1–0.2 Å longer than that in the ortho-benzene ring: 1.56 Å (1b, 4b, and 12b) vs. 1.39–1.41 Å (ortho-benzene).
Angles φ1 and φ2 were similar in both scaffolds: 61–65° (1b, 4b, and 12b) vs. 55–57° (ortho-benzene). The difference in planarity, however, between the saturated scaffold and the benzene ring was significant: while ortho-benzene was almost flattened, bicyclo[2.1.1]hexanes had a significant three-dimensional character:/θ/= 56–59° (1b, 4b, and 12b) vs. 7–8° (ortho-benzene).
In general, both distances r and d and angles φ1 and φ2 of 1,2-disubstituted bicyclo[2.1.1]hexanes were similar to those of the ortho-substituted benzene ring.
Incorporation into bioactive compounds
The incorporation of the bicyclo[2.1.1]hexane scaffold into bioactive compounds was then attempted. We chose five drugs and agrochemicals with an ortho-substituted benzene ring: agent for hyponatremia treatment conivaptan, lipid-lowering agent lomitapide (Scheme 3); fungicides boscalid, bixafen, and fluxapyroxad (Scheme 4).
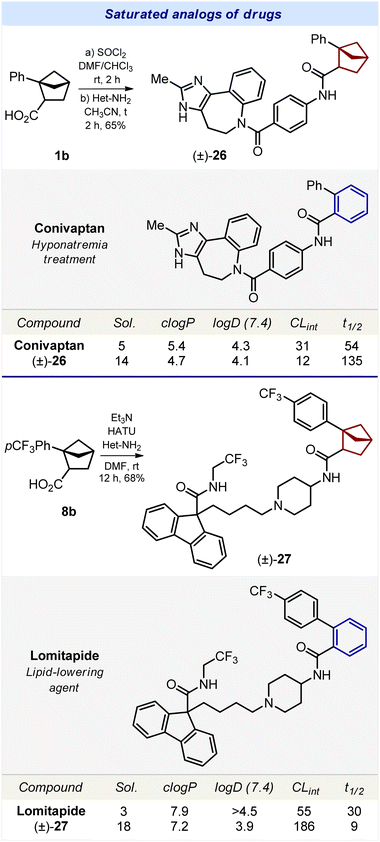 |
| Scheme 3 Synthesis and properties of compounds 26 and 27 – saturated analogs of drugs conivaptan and lomitapide, correspondingly. HATU: hexafluorophosphate azabenzotriazole tetramethyl uronium. Sol.: the experimental kinetic solubility in phosphate-buffered saline, pH 7.4 (μM). c log P: the calculated lipophilicity. log D (7.4): the experimental distribution coefficient in n-octanol/phosphate-buffered saline, pH 7.4. Reliable log D values could be obtained within a range of 1.0–4.5. CLint: the experimental metabolic stability in human liver microsomes (μL min−1 mg−1). t1/2 (min): the experimental half-time of metabolic decomposition. | |
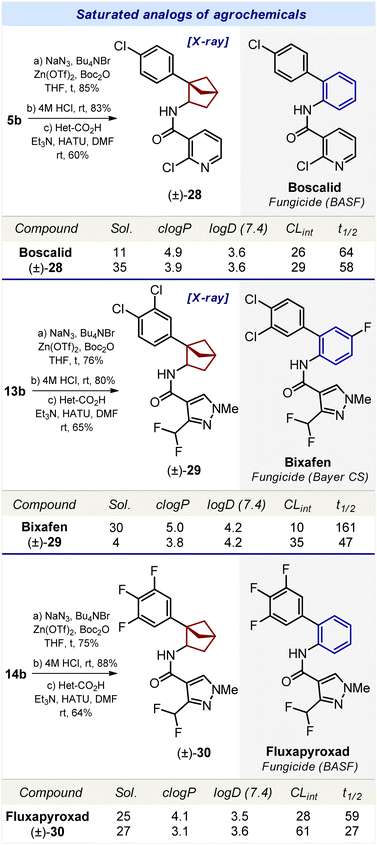 |
| Scheme 4 Synthesis and properties of compounds 28–30 – saturated analogs of agrochemicals boscalid, bixafen, and fluxapyroxad, correspondingly. Sol.: the experimental kinetic solubility in phosphate-buffered saline, pH 7.4 (μM). c log P: the calculated lipophilicity. log D (7.4): the experimental distribution coefficient in n-octanol/phosphate-buffered saline, pH 7.4. Reliable log D values could be obtained within a range of 1.0–4.5. CLint, clearance intrinsic (μL min−1 mg−1): experimental metabolic stability in human liver microsomes. t1/2 (min): the experimental half-time of metabolic decomposition. | |
Synthesis of the saturated analog of conivaptan was performed from carboxylic acid 1b (Scheme 3). Amide coupling with the corresponding para-substituted aniline gave the desired compound 26. Compound 27, – a saturated analog of lomitapide, – was obtained analogously from carboxylic acid 8b (Scheme 3).
Synthesis of the saturated analog of boscalid was performed from carboxylic acid 5b (Scheme 4). The Curtius reaction followed by acylation of the intermediate amine with 2-chloropyridine-3-carboxylic acid gave compound 28. Using an analogous strategy, compound 29, – a saturated analog of bixafen, – was obtained from carboxylic acid 13b (Scheme 4). The saturated analog of fluxapyroxad, compound 30, was synthesized from carboxylic acid 14b.
The structures of bicyclo[2.1.1]hexanes 28 and 29 were confirmed by X-ray crystallographic analysis.23
Physicochemical parameters
We studied the effect of the replacement of the ortho-substituted benzene ring by bicyclo[2.1.1]hexanes on the physicochemical properties of bioactive compounds (Schemes 3 and 4).
Water solubility.
Replacement of the ortho-benzene ring in conivaptan by bicyclo[2.1.1]hexane (26) increased the solubility by threefold: 5 μM (conivaptan) vs. 14 μM (26) (Scheme 3). An analogous trend was observed with lomitapide. Replacement of the benzene ring in lomitapide with bicyclo[2.1.1]hexane (27) led to a dramatic sixfold increase in solubility: 3 μM (lomitapide) vs. 18 μM (27).
Replacement of the ortho-benzene ring by bicyclo[2.1.1]hexane in agrochemicals boscalid, bixafen, and fluxapyroxad produced conflicting results. In boscalid, such a replacement led to a significant threefold increase in solubility: 11 μM (boscalid) vs. 35 μM (28). In bixafen, the opposite effect was observed, and the solubility was reduced: 30 μM (bixafen) vs. 4 μM (29).26 In fluxapyroxad, such a replacement resulted in a slight increase in solubility: 25 μM (fluxapyroxad) vs. 27 μM (30).
In conclusion, in four out of the five bioactive compounds, the replacement of the ortho-benzene ring by bicyclo[2.1.1]hexane led to an enhanced water solubility.
Lipophilicity.
To estimate the influence of the replacement of the ortho-benzene ring with bicyclo[2.1.1]hexane on lipophilicity, we used two parameters: calculated lipophilicity (c
log
P)27 and experimental lipophilicity (log
D).
Replacement of the ortho-benzene ring in conivaptan, lomitapide, boscalid, bixafen, and fluxapyroxad with bicyclo[2.1.1]hexane (26–30) led to a decrease in c
log
P by 0.7–1.2 units.
Replacement of the ortho-benzene ring with bicyclo[2.1.1]hexane had only a small effect on the logD index. In four bioactive compounds (conivaptan, boscalid, bixafen, and fluxapyroxad) such a replacement almost did not affect log
D. Only in lomitapide, the saturated analog 27 had a significantly lower log
D index: >4.5 (lomitapide) vs. 3.9 μM (27).
In summary, in all five bioactive compounds, replacement of the ortho-benzene ring by bicyclo[2.1.1]hexane led to a decrease in calculated lipophilicity (c
log
P) by 0.7–1.2 units; and in four of them it had little effect on the experimental lipophilicity (log
D).
Metabolic stability.
The effect of bicyclo[2.1.1]hexane on the metabolic stability of bioactive compounds was more complex. In conivaptan, the incorporation of bicyclo[2.1.1]hexane (26) increased the metabolic stability: CLint (μL min−1 mg−1) = 31 (conivaptan) vs. 12 (26). In lomitapide, bixafen, and fluxapyroxad the incorporation of bicyclo[2.1.1]hexane (27, 29, and 30) dramatically decreased the metabolic stability by two (30) to three (27, 29) times, as measured by using t1/2 (min). In boscalid, such a replacement led to a slight decrease in metabolic stability: CLint (μL min−1 mg−1) = 26 (boscalid) vs. 29 (28) (Schemes 3 and 4).
In brief summary, in four out of the five bioactive compounds, replacement of the ortho-benzene ring with bicyclo[2.1.1]hexane decreased the metabolic stability.
Bioactivity
Finally, we wanted to answer the key question, – can 1,2-disubstituted bicyclo[2.1.1]hexanes indeed act as bioisosteres of the ortho-benzene ring in bioactive compounds?9 Therefore, we measured the antifungal activity of the marketed fungicides boscalid (BASF), bixafen (Bayer CS), fluxapyroxad (BASF), and their saturated analogs 28–30. In strict contrast to medicinal chemistry, the use of racemic mixtures in agrochemistry is common;5 therefore for the validation of the proof-of-concept, we directly studied the biological activity of the available racemic compounds 28–30 (Fig. 4).
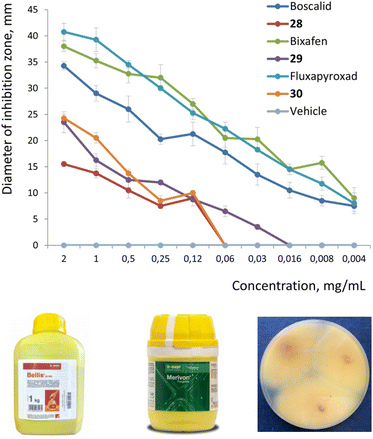 |
| Fig. 4 Inhibition of growth of Aspergillus niger (strain VURV-F 822; measured as a diameter of the inhibition zone, mm) with boscalid, bixafen, fluxapyroxad, and their analogs 28–30 at different concentrations after 48 h of incubation. Fungicides boscalid (@Bellis) and bixafen (@Merivon) are shown. Inhibition of fungal growth by compound 29. | |
We measured the antifungal activity of all six compounds against the fungal strain Aspergillus niger, – using the disk diffusion method (ESI, p. S327–S333†). Even though the original agrochemicals were more potent, all three saturated analogs 28–30 were active and showed a high inhibition of the growth of Aspergillus niger compared to the vehicle (Fig. 4; and ESI, p. S327–S333†).
Conclusions
In this work, we have synthesized, characterized, and biologically validated 1,2-disubstituted bicyclo[2.1.1]hexanes as saturated bioisosteres of the ortho-substituted benzenes. These structures were obtained from readily available and inexpensive starting materials (acetophenone) on a multigram scale (Schemes 1 and 2). Physicochemical and geometric properties of bicyclo[2.1.1]hexanes were measured and compared to those of the ortho-substituted benzenes (Fig. 3). The incorporation of the bicyclo[2.1.1]hexane core into the structure of agrochemicals boscalid (BASF), bixafen (Bayer CS), and fluxapyroxad (BASF) gave the saturated patent-free analogs 28–30 with high antifungal activity.
We believe that given the commonplace of the phenyl group in chemistry, its saturated bioisosteres developed here will become common in medicinal chemistry in the coming years.
Data availability
The ESI† contains method description, product characterization data, and NMR spectra.
Author contributions
A. D. and P. K. M. designed the project. A. D., P. G., Y. M., O. S., G. A.-M., and R. K. carried out the experiments. I. V. S. and P. K. M. wrote the manuscript and all authors provided comments.
Conflicts of interest
A. D., P. G., Y. M., O. S., G. A.-M., R. K., I. V. S., and P. K. M. are employees of a chemical supplier Enamine.
Acknowledgements
The authors are grateful to Prof. A. A. Tolmachev for the support of this work. This project has received funding from the European Research Council (ERC) under the European Union's Horizon 2020 research and innovation program (grant agreement no. 101000893 – BENOVELTY). PM is very grateful to Dr S. Shishkina (IOC, Kyiv) for the X-ray studies; to Dr P. Borysko (Bienta), Dr Y. Holota (Bienta), and Mrs L. Bortnichuk (Bienta) for ADME studies; to Dr Vladimir Kubyshkin (Enamine) and Dr Bill Heilman for proofreading the text and helpful suggestions.
Notes and references
- Y. Chen, C. Rosenkranz, S. Hirte and J. Kirchmair, Nat. Prod. Rep., 2022, 39, 1544–1556 RSC
.
- R. D. Taylor, M. MacCoss and A. D. G. Lawson, J. Med. Chem., 2014, 57, 5845–5859 CrossRef CAS PubMed
.
- J. Shearer, J. L. Castro, A. D. G. Lawson, M. MacCoss and R. D. Taylor, J. Med. Chem., 2022, 65, 8699–8712 CrossRef CAS PubMed
.
-
http://www.drugbank.ca
.
-
The Pesticide Manual, ed. C. MacBean, British Crop Production Council, 2012 Search PubMed
.
-
(a) A. Denisenko, P. Garbuz, N. M. Voloshchuk, Y. Holota, G. Al-Maali, P. Borysko and P. K. Mykhailiuk, Nat. Chem., 2023, 15, 1155–1163 CrossRef CAS PubMed
;
(b) A. Denisenko, P. Garbuz, S. V. Shishkina, N. M. Voloshchuk and P. K. Mykhailiuk, Angew. Chem., Int. Ed., 2020, 59, 20515–20521 CrossRef CAS PubMed
.
-
(a) R. Kleinmans, T. Pinkert, S. Dutta, T. O. Paulisch, H. Keum, C. G. Daniliuc and F. Glorius, Nature, 2022, 605, 477–482 CrossRef CAS PubMed
;
(b) R. Kleinmans, S. Dutta, K. Ozols, H. Shao, F. Schafer, R. E. Thielemann, H. T. Chan, C. G. Daniliuc, K. N. Houk and F. Glorius, J. Am. Chem. Soc., 2023, 145, 12324–12332 CrossRef CAS PubMed
.
- R. Guo, Y.-C. Chang, L. Herter, C. Salome, S. E. Braley, T. Fessard and M. K. Brown, J. Am. Chem. Soc., 2022, 144, 7988–7994 CrossRef CAS PubMed
.
- S. Agasti, F. Beltran, E. Pye, N. Kaltsoyannis, G. E. M. Crisenza and D. J. Procter, Nat. Chem., 2023, 15, 535–541 CrossRef CAS PubMed
.
- M. Xu, Z. Wang, Z. Sun, Y. Ouyang, Z. Ding, T. Yu, L. Xu and P. Li, Angew. Chem., Int. Ed., 2022, 61, e202214507 CrossRef CAS PubMed
.
- Y. Liu, S. Lin, Y. Li, J.-H. Xue, Q. Li and H. Wang, ACS Catal., 2023, 13, 5096–5103 CrossRef CAS
.
- N. Radhoff, C. G. Daniliuc and A. Studer, Angew. Chem., Int. Ed., 2023, 62, e202304771 CrossRef CAS PubMed
.
- New photochemical approaches to bicyclo[2.1.1]hexanes appeared recently:
(a) L. Herter, I. Koutsopetras, L. Turelli, T. Fessard and C. Salomé, Org. Biomol. Chem., 2022, 20, 9108–9111 RSC
;
(b) T. Rigotti and T. Bach, Org. Lett., 2022, 24, 8821–8825 CrossRef CAS PubMed
;
(c) S. Paul, D. Adelfinsky, C. Salome, T. Fessard and M. K. Brown, Chem. Sci., 2023, 14, 8070–8075 RSC
;
(d) M. Reinhold, J. Steinebach, C. Golza and J. C. L. Walker, Chem. Sci., 2023, 14, 9885–9891 RSC
;
(e) R. Jeyaseelan, M. Utikal, C. G. Daniliuc and L. Næsborg, Chem. Sci., 2023, 14, 11040–11044 RSC
.
- For the synthesis of bicyclo[2.1.1]hexane-containing boronates, see: Y. Yang, J. Tsien, J. M. E. Hughes, B. K. Peters, R. R. Merchant and T. Qin, Nat. Chem., 2021, 13, 950–955 CrossRef CAS PubMed
.
- 2-Oxabicyclo[2.1.1]hexanes:
(a) Y. Liang, R. Kleinmans, C. G. Daniliuc and F. Glorius, J. Am. Chem. Soc., 2022, 144, 20207–20213 CrossRef CAS PubMed
;
(b) Y. Liang, F. Paulus, C. G. Daniliuc and F. Glorius, Angew. Chem., Int. Ed., 2023, 62, e202305043 CrossRef CAS PubMed
.
-
(a) X. Ma, Y. Han and D. J. Bennett, Org. Lett., 2020, 22, 9133–9138 CrossRef CAS PubMed
;
(b) J.-X. Zhao, Y.-X. Chang, C. He, B. J. Burke, M. R. Collins, M. D. Bel, J. Elleraas, G. M. Gallego, T. P. Montgomery, J. J. Mousseau, S. K. Nair, M. A. Perry, J. E. Spangler, J. C. Vantourout and P. S. Baran, Proc. Natl. Acad. Sci. U.S.A., 2020, 118, e2108881118 CrossRef PubMed
;
(c) O. L. Garry, M. Heilmann, J. Chen, Y. Liang, X. Zhang, X. Ma, C. S. Yeung, D. J. Bennett and D. W. C. MacMillan, J. Am. Chem. Soc., 2023, 145, 3092–3100 CrossRef CAS PubMed
;
(d) B. A. Wright, A. Matviitsuk, M. J. Black, P. García-Reynaga, L. E. Hanna, A. T. Herrmann, M. K. Ameriks, R. Sarpong and T. P. Lebold, J. Am. Chem. Soc., 2023, 145, 10960–10966 CrossRef CAS PubMed
.
-
(a) A. S. Harmata, T. E. Spiller, M. J. Sowden and C. R. J. Stephenson, J. Am. Chem. Soc., 2021, 143, 21223–21228 CrossRef CAS PubMed
;
(b) N. Frank, J. Nugent, B. R. Shire, H. D. Pickford, P. Rabe, A. J. Sterling, T. Zarganes-Tzitzikas, T. Grimes, A. L. Thompson, R. C. Smith, C. J. Schofield, P. E. Brennan, F. Duarte and E. A. Anderson, Nature, 2022, 611, 721–726 CrossRef CAS PubMed
;
(c) T. Iida, J. Kanazawa, T. Matsunaga, K. Miyamoto, K. Hirano and M. Uchiyama, J. Am. Chem. Soc., 2022, 144, 21848–21852 CrossRef CAS PubMed
;
(d) Y. Zheng, W. Huang, R. K. Dhungana, A. Granados, S. Keess, M. Makvandi and G. A. Molander, J. Am. Chem. Soc., 2022, 144, 23685–23690 CrossRef CAS PubMed
;
(e) T. Yu, J. Yang, Z. Wang, Z. Ding, M. Xu, J. Wen, L. Xu and P. Li, J. Am. Chem. Soc., 2023, 145, 4304–4310 CrossRef CAS PubMed
.
-
(a) B. A. Chalmers, H. Xing, S. Houston, C. Clark, S. Ghassabian, A. Kuo, B. Cao, A. Reitsma, C.-E. P. Murray, J. E. Stok, G. M. Boyle, C. J. Pierce, S. W. Littler, D. A. Winkler, P. V. Bernhardt, C. Pasay, J. J. De Voss, J. McCarthy, P. G. Parsons, M. T. Smith, H. M. Cooper, S. K. Nilsson, J. Tsanaktsidis, G. P. Savage and C. M. Williams, Angew. Chem., Int. Ed., 2016, 55, 3580–3585 CrossRef CAS PubMed
;
(b) M. P. Wiesenfeldt, J. A. Rossi-Ashton, I. B. Perry, J. Diesel, O. L. Garry, F. Bartels, S. C. Coote, X. Ma, C. S. Yeung, D. J. Bennett and D. W. C. MacMillan, Nature, 2023, 618, 513–518 CrossRef CAS PubMed
;
(c) T. A. Reekie, C. M. Williams, L. M. Rendina and M. Kassiou, J. Med. Chem., 2019, 62, 1078–1095 CrossRef CAS PubMed
.
- P. M. Mykhailiuk, Org. Biomol. Chem., 2019, 17, 2839–2849 RSC
.
- M. A. M. Subbaiah and N. A. Meanwell, J. Med. Chem., 2021, 64, 14046–14128 CrossRef CAS PubMed
.
- The most popular reaction in medicinal chemistry is the synthesis of amides from carboxylic acids and amines: D. G. Brown and J. Boström, J. Med. Chem., 2016, 59, 4443–4458 CrossRef CAS PubMed
.
- Amines could be easily obtained from carboxylic acids via the Curtius reaction (see the ESI, p. S45–S48).†.
- Cambridge Crystallographic Data Center (CCDC) deposition numbers: 1b (2286523), 3b (2286521), 4b (2286526), 10b (2286525), 12b (2286524), 31 (2286523) and 32 (2286527).
- This tactic is currently being used to obtain 1,3-disubstituted bicyclo[1.1.1]pentanes by the stepwise modifications of the carboxylic groups in bicyclo[1.1.1]pentane-1,3-dicarboxylic acid: V. Ripenko, D. Vysochyn, I. Klymov, S. Zhersh and P. K. Mykhailiuk, J. Org. Chem., 2021, 86, 14061–14068 CrossRef CAS PubMed
.
-
(a) J.-R. Wang, X. Wang, L. Lu and X. Mei, Cryst. Growth Des., 2013, 13, 3261–3269 CrossRef CAS
;
(b) R. Chadha, S. Bhandari, J. Haneef, S. Khullar and S. Mandal, CrystEngComm, 2014, 16, 8375–8389 RSC
.
- Technically, the comparison of bixafen and its saturated analog 29 is not accurate, because bixafen has an additional polar C(sp2)–F bond, and analog 29 does not have it.
-
c
log
P was calculated with ChemAxon Marvin Sketch (version 22.13).
|
This journal is © The Royal Society of Chemistry 2023 |