DOI:
10.1039/D3SC01876H
(Edge Article)
Chem. Sci., 2023,
14, 6860-6866
A conductive catecholate-based framework coordinated with unsaturated bismuth boosts CO2 electroreduction to formate†
Received
11th April 2023
, Accepted 22nd May 2023
First published on 31st May 2023
Abstract
Bismuth-based metal–organic frameworks (Bi-MOFs) have received attention in electrochemical CO2-to-formate conversion. However, the low conductivity and saturated coordination of Bi-MOFs usually lead to poor performance, which severely limits their widespread application. Herein, a conductive catecholate-based framework with Bi-enriched sites (HHTP, 2,3,6,7,10,11-hexahydroxytriphenylene) is constructed and the zigzagging corrugated topology of Bi–HHTP is first unraveled via single-crystal X-ray diffraction. Bi–HHTP possesses excellent electrical conductivity (1.65 S m−1) and unsaturated coordination Bi sites are confirmed by electron paramagnetic resonance spectroscopy. Bi–HHTP exhibited an outstanding performance for selective formate production of 95% with a maximum turnover frequency of 576 h−1 in a flow cell, which surpassed most of the previously reported Bi-MOFs. Significantly, the structure of Bi–HHTP could be well maintained after catalysis. In situ attenuated total reflectance Fourier transform infrared spectroscopy (ATR-FTIR) confirms that the key intermediate is *COOH species. Density functional theory (DFT) calculations reveal that the rate-determining step is *COOH species generation, which is consistent with the in situ ATR-FTIR results. DFT calculations confirmed that the unsaturated coordination Bi sites acted as active sites for electrochemical CO2-to-formate conversion. This work provides new insights into the rational design of conductive, stable, and active Bi-MOFs to improve their performance towards electrochemical CO2 reduction.
Introduction
With ever-increasing global energy demand and the influence of climate change, the electrochemical CO2 reduction reaction (CO2RR) has attracted enormous attention for converting CO2 into value-added fuels and feedstocks, which is not only a promising way to reduce the negative impact of climate change but also enriches energy supply and achieves net-zero CO2 emission.1,2 Formate, as one of the main CO2RR products, has promising potential because of its compatibility with existing infrastructure and the promise for hydrogen storage and textile or leather production, proposing a carbon-neutral route to fuel generation.3–6
Electrocatalysts play a vital role in efficient CO2-to-formate conversion.4,7,8 Bismuth (Bi) with its low-cost and environment-friendly nature exhibits adequate adsorption intensity toward intermediate species for formate, attracting more and more attention.9–11 Considering its low natural abundance and potential future large-scale applications, it is crucial to enhance the atomic utilization of Bi. Bi-based metal–organic frameworks (Bi-MOFs) with well-defined coordination and single-site dispersion are regarded as promising approaches to address this issue.12,13 But their low electrical conductivity and saturated coordination mode remain significant obstacles to their performance and severely limit their broad application. Spectacularly, conductive MOFs (cMOFs) are deemed proactive materials for achieving excellent performance without the pyrolysis process owing to their distinctive electrical conductivity.14–21 Despite the advances in the CO2RR with cMOFs such as Cu-based cMOFs,22–24 there is still a scarcity of literature on conductive Bi-MOFs for electrochemical CO2-to-formate conversion with enhanced activity and satisfactory selectivity.
In this work, a three-dimensional (3D) Bi-based catecholate cMOF (Bi–HHTP) (HHTP, 2,3,6,7,10,11-hexahydroxytriphenylene) with enriched defects was fabricated for the CO2RR and single-crystal X-ray diffraction verified its structure. Bi–HHTP with its robust nature could achieve optimal faradaic efficiency (FE) towards formate (95%) with a maximum turnover frequency (TOF) of ∼576 h−1. In situ attenuated total reflectance Fourier transform infrared spectroscopy (ATR-FTIR) and density functional theory (DFT) calculations confirmed that the key intermediate is *COOH species. DFT calculations indicated that the unsaturated coordination Bi3+ sites could effectively facilitate the dissociation of *COOH to produce formate.
Results and discussion
Preparation and characterization
In this work, 3D Bi–HHTP was constructed via the solvothermal method (Fig. 1). Single-crystal X-ray diffraction (XRD) disclosed that Bi–HHTP exhibited a monoclinic type Bravais lattice (α, γ = 90° and β = 143.57(2)°) with space group P21/n. Intriguingly, the zigzagging corrugated chains of nonplanar ligands were coupled by the Bi–O bond (Fig. 1a–d), where each HHTP attached to seven Bi3+ ions at different angles (Fig. 1e) and the Bi3+ ion coordinated with the nonplanar catechol groups to form the unsaturated coordination mode (distorted tetragonal pyramid) (Fig. 1f). XRD patterns in Fig. 2a further confirmed the difference in Bi–HHTP, compared to traditional Ni–HHTP. No preferential orientation was found in Bi–HHTP. Thereafter, the conductivity of Bi–HHTP was measured by the two-contact probe technique. According to the current–voltage characteristic shown in Fig. 2b, Bi–HHTP exhibited an electrical conductivity of 1.65 S m−1, which is higher than those of the reported MOFs as listed in Fig. 2c and Table S2.† Electron paramagnetic resonance spectroscopy (EPR) could effectively disclose the existence of unpaired electrons.25 The EPR spectrum of Bi–HHTP (Fig. 2d) revealed a high-intensity peak at g = 2.005, verifying the presence of unpaired electrons located on defect sites. Transmission electron microscopy (TEM) and scanning electron microscopy (SEM) images showed that the morphology of Bi–HHTP was nanobelt-like (Fig. 2e, S1a and b†). The lattice fringes of Bi–HHTP in specific domains with lattice parameters of 0.18 nm matched well with [−4 −3 8] of Bi–HHTP (inset of Fig. 2e). The composition of Bi–HHTP was analyzed using the high-angle annular dark-field scanning transmission electron microscopy (HAADF-STEM) image and the corresponding energy-dispersive X-ray spectroscopy (EDS) elemental mappings (Fig. 2f), indicating the homogeneous distribution of Bi, C, and O elements.
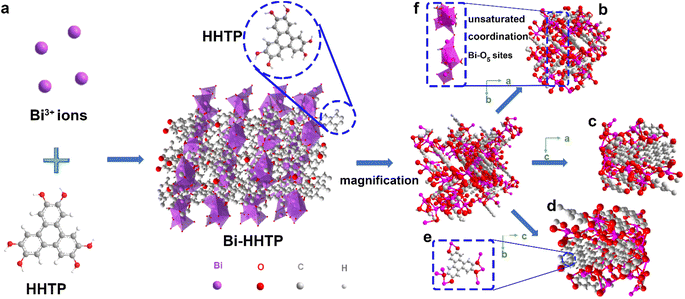 |
| Fig. 1 (a) The general process for the synthesis of Bi–HHTP. (b–d) The views in a different dimension. (e) Chelation of HHTP toward Bi3+ ions. (f) The coordination environment of Bi3+ ions (distorted tetragonal pyramid). | |
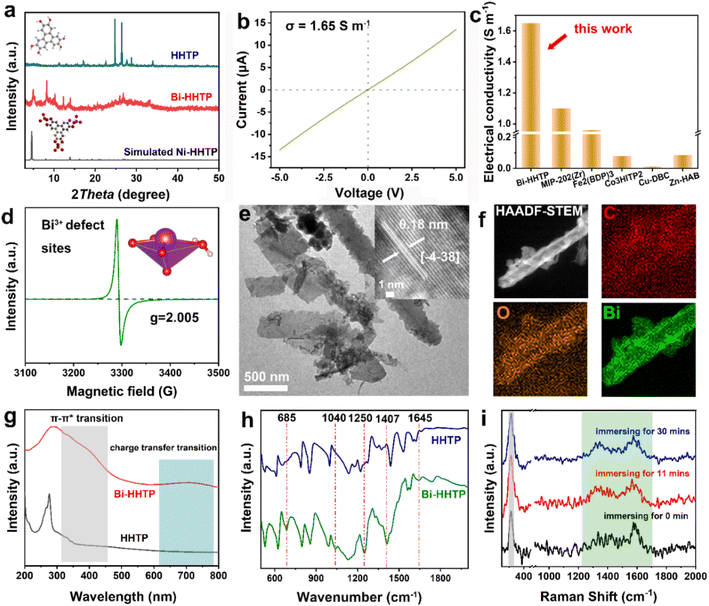 |
| Fig. 2 (a) XRD patterns of Bi–HHTP and HHTP. (b) Current–voltage characteristic of Bi–HHTP using the two-contact probe method. (c) The compassion of conductivity of different MOFs. (d) EPR spectrum of Bi–HHTP. (e) TEM image, (f) HAADF-STEM image and the corresponding EDS elemental mappings of Bi–HHTP. (g) UV-vis spectra of Bi–HHTP and HHTP. (h) FTIR and (i) in situ Raman spectra of Bi–HHTP. | |
Besides, UV-vis and FTIR were also employed to detect the feature of Bi–HHTP. The peaks of UV-vis spectra shown in Fig. 2g at ∼380 nm and ∼700 nm correspond to π–π* and the ligand-to-metal charge transfer between the Bi3+ ion and HHTP.26,27 Moreover, the peak at 685 cm−1 for FTIR shown in Fig. 2h was attributed to the vibration of the Bi–O entities, providing convincing evidence for forming Bi–HHTP.16,28,29 Furthermore, we carried out in situ Raman tests to prove the robust stability of Bi–HHTP in 0.5 M KHCO3 aqueous solution (Fig. 2i). The stretching mode of Bi–O entities was found at 313 cm−1.30 The peaks at around 1335 and 1582 cm−1 could be attributed to the characteristic defect (D) and graphitic (G) bands.20,26,31 Remarkably, the unchanged Bi–O entities in KHCO3 aqueous solution illustrated the robust structure of Bi–HHTP, compared to the reported Bi-based MOFs.12,19
Electrochemical performance
The CO2RR performance was investigated in a flow cell (Fig. 3a) and the strong alkaline solution could enhance the conductivity of the electrolyte (0.194 S cm−1, 1 M KOH) and lower the overpotential.32,33 The liquid-phase products were detected by nuclear magnetic resonance (Fig. S4†). The polarization curves over Bi–HHTP in CO2-purged cells shown in Fig. 3b illustrated a more positive onset potential and dramatically larger current densities than those in N2-purged cells, signifying that Bi–HHTP could lower the reaction barriers for the CO2RR. Inspiringly, the FEformate of Bi–HHTP shown in Fig. 3c could exceed 95% with a cathodic energy efficiency (CEE) of 68.8% at −0.7 V vs. RHE. The current density of Bi–HHTP was 93 mA cm−2 at −1.1 V vs. RHE.
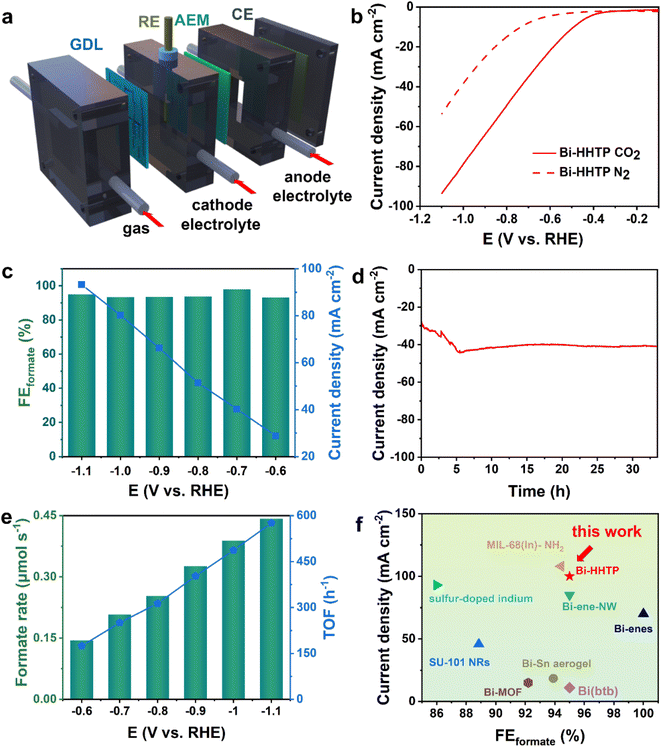 |
| Fig. 3 (a) The illustration scheme of a liquid-phase flow cell device. (GDL, CE, RE, and AEM represent the working electrode, counter electrode, reference electrode, and anion exchange membrane, respectively) (b) LSV curves of Bi–HHTP in CO2 and N2 atmospheres without correction. (c) Potential-dependent formate FEs and current density of Bi–HHTP. (d) Stability test of Bi–HHTP at −0.7 V vs. RHE. (e) Formate rate and TOF of Bi–HHTP. (f) Comparison of our work with previously reported literature. | |
Afterward, there was no discernible change in current density for over 30 h (Fig. 3d). The formate produced by Bi–HHTP at various potentials (Fig. 3e) illustrated that the generation rate could exceed 0.4 μmol s−1 and the TOF of Bi–HHTP could reach a maximum of 576 h−1. Meanwhile, Bi–HHTP demonstrated a high FE of formate with a high current density, which outperforms most of the reported Bi-based or In-based materials (Fig. 3f and Table S3†). To boost the electrocatalytic activity of Bi–HHTP, this work also replaced the oxygen evolution reaction (OER) with the methanol oxidation reaction (MOR) (Fig. S9–S18†) to promote reaction kinetics. Inspiringly, the pair of CO2RR//MOR systems only needed −1.9 V to achieve a current density of 10 mA cm−2, much lower (about 400 mV) than that for the CO2RR//OER (Fig. S19†). Moreover, the produced formate was quantitatively detected after electrolysis. Notably, the FEformate for the CO2RR was over 90% from −2.1 to −2.5 V.
In situ ATR-FTIR spectroscopy and DFT calculations
To ascertain the intermediates and gain an understanding of the structure–performance relationship, in situ ATR-FTIR spectra were recorded and DFT calculations were applied. As shown in Fig. 4a, two FTIR signals at ∼1379 and 1410 cm−1 are assigned to the formation of *COOH species,33 and the peak at 1643 cm−1 arises from the vibration frequency of the carboxylate (*CO2−).34–36
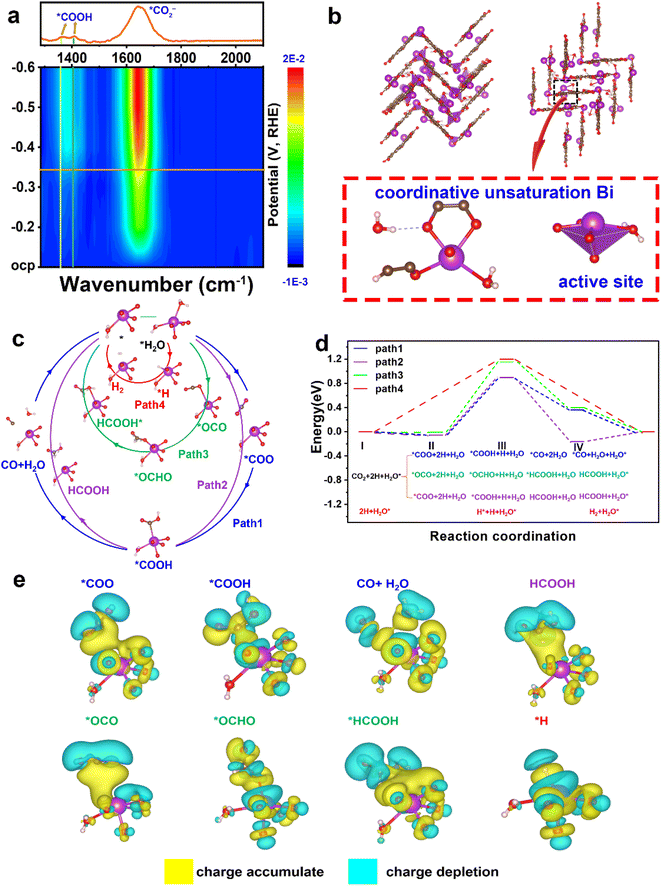 |
| Fig. 4 (a) In situ ATR-FTIR spectra in CO2-saturated 0.5 M KHCO3 at applied potentials. (b) The active site Bi coordinative unsaturation with 4 oxygen atoms and one H2O molecule bonded. (c) Four proposed reaction pathways on the active site to different products corresponding to the CO2RR and HER. (d) The energy profiles in eV for the four pathways proposed in (c) with active compositions. (e) The charge difference of every active species (*COO, *COOH, CO + H2O, HCOOH, *OCO, *OCHO, *HCOOH, and *H) on the catalyst surface. The yellow part indicates the charge accumulation and the cyan part illustrates the charge depletion. | |
The structural pattern of Bi–HHTP may be regarded as being composed of BiO4–H2O joined by organic linkers (HHTP). The Bi3+ ions have an unusual unsaturated coordination in their structure. As opposed to the saturated coordination of Bi3+ ions with six chemical bonds, the Bi site relates to four oxygen atoms and one water molecule (Fig. 4b). Fig. S20† illustrates the density of states of active Bi coordinative unsaturation. Four potential routes of unsaturated coordination Bi3+ sites for the CO2RR are provided (paths 1–4 in Fig. 4c). Path 1 produces CO, paths 2 and 3 result in generating HCOOH, and path 4 is the hydrogen evolution reaction (HER) pathway. The active site is denoted as H2O* (* denotes the adsorption state) because the active Bi3+ sites were distorted tetragonal pyramids and one of the ligands is H2O. In Fig. 4d, the energy profiles are displayed, showing that step II indicates the rate-determining step (RDS) and paths 1 and 2 are the best routes for producing HCOOH due to the lower RDS energy barrier (0.897 eV), compared to path 3 (1.15 eV) and path 4 (1.2 eV). Additionally, the blue value (path 1) is higher than the purple data (path 2). The HCOOH formation from step IV is preferable to CO generation, which is consistent with the in situ ATR-FTIR signals at ∼1379 and 1410 cm−1. The stronger selectivity for HCOOH shown between paths 1 and 2 is because *COOH on the Bi3+ site is strong enough to weaken the carbon–oxygen double bond. Path 4 for the HER has the highest energy barrier of 1.2 eV, which indicates that the generation of H2 is unfavorable.37 Therefore, the CO2RR is preferable to the HER because CO2 is easier to adsorb than H. The charge difference of every active species (*COO, *COOH, CO + H2O, HCOOH, *OCO, *OCHO, *HCOOH, and *H) on the catalyst surface is displayed in Fig. 4e. The yellow part indicates the charge accumulation and cyan part illustrates the charge depletion. Much charge transfer could be found between the unsaturated coordination Bi3+ sites and active species. The experimental in situ ATR-FTIR results and the DFT data both show that Bi–HHTP exhibits a strong selectivity for HCOOH production.
Conclusions
In summary, this work fabricates conductive Bi–HHTP via the solvothermal method, and its crystal structure is first unravelled via single-crystal X-ray diffraction. Bi–HHTP with excellent electrical conductivity (1.65 S m−1) exhibits numerous defects, which is verified by EPR. Bi–HHTP displays high selectivity to formate (95%) in a flow cell with a CEE of 68.8% and a maximum TOF of 576 h−1. The in situ Raman spectroscopy, XRD, and XPS results further confirm its robust structure. Both in situ ATR-FTIR and DFT calculations confirm that the key intermediate is *COOH species. DFT calculations also confirm that the unsaturated coordination Bi sites within Bi–HHTP serve as active sites, which could promote charge transfer and lower the energy barrier (0.897 eV) for producing HCOOH. Besides, the electrocatalytic activity of Bi–HHTP is boosted by replacing the OER with the MOR, illustrating that the CO2RR//MOR system requires less overpotential (about 400 mV) than the CO2RR//OER to achieve a current density of 10 mA cm−2. This work paves a new way into constructing highly active and stable cMOFs for high-performance CO2RR, in terms of experimental and theoretical aspects.
Data availability
All relevant data are presented in the main text and ESI.†
Author contributions
Zengqiang Gao wrote the original draft and carried out the characterization and catalysis. Man Hou, Yongxia Shi, and Li Li participated in the characterization and helped draft the manuscript. Qisheng Sun and Zhiqiang Jiang joined the discussion of the data and gave useful suggestions. Shuyuan Yang participated in the characterization. Zhicheng Zhang, Wenjuan Yang and Wenping Hu supervised and guided the project.
Conflicts of interest
There are no conflicts to declare.
Acknowledgements
This work was supported by the National Natural Science Foundation of China (No. 22071172, 52121002, 51733004, and 21866032) and the National Key R&D Program of China (No. 2018YFA0703200). Financial support was provided by the Haihe Laboratory of Sustainable Chemical Transformations. The authors thank Prof. Jun Xu (School of Pharmaceutical Science and Technology, Tianjin University) for the analysis of single-crystal X-ray >diffraction.
Notes and references
- S. Chu, Y. Cui and N. Liu, The path towards sustainable energy, Nat. Mater., 2017, 16, 16–22 CrossRef.
- H. Xu, D. Rebollar, H. He, L. Chong, Y. Liu, C. Liu, C.-J. Sun, T. Li, J. V. Muntean, R. E. Winans, D.-J. Liu and T. Xu, Highly selective electrocatalytic CO2 reduction to ethanol by metallic clusters dynamically formed from atomically dispersed copper, Nat. Energy, 2020, 5, 623–632 CrossRef CAS.
- J. Zhang, W. Cai, F. X. Hu, H. Yang and B. Liu, Recent advances in single atom catalysts for the electrochemical carbon dioxide reduction reaction, Chem. Sci., 2021, 12, 6800–6819 RSC.
- Y. Y. Birdja, E. Pérez-Gallent, M. C. Figueiredo, A. J. Göttle, F. Calle-Vallejo and M. T. M. Koper, Advances and challenges in understanding the electrocatalytic conversion of carbon dioxide to fuels, Nat. Energy, 2019, 4, 732–745 CrossRef CAS.
- J. Li, Y. Sun and Z. Zhang, Perspective on machine learning in energy material discovery, SmartMat, 2023 DOI:10.1002/smm2.1171.
- B. Wang, S. Chen, Z. Zhang and D. Wang, Low-dimensional material supported single-atom catalysts for electrochemical CO2 reduction, SmartMat, 2022, 3, 84–110 CrossRef CAS.
- Y. Wang, P. Han, X. Lv, L. Zhang and G. Zheng, Defect and Interface Engineering for Aqueous Electrocatalytic CO2 Reduction, Joule, 2018, 2, 2551–2582 CrossRef CAS.
- Y. Yang, M. Z. Ertem and L. Duan, An amide-based second coordination sphere promotes the dimer pathway of Mn-catalyzed CO2-to-CO reduction at low overpotential, Chem. Sci., 2021, 12, 4779–4788 RSC.
- S. Chu, C. Kang, W. Park, Y. Han, S. Hong, L. Hao, H. Zhang, T. W. B. Lo, A. W. Robertson, Y. Jung, B. Han and Z. Sun, Single atom and defect engineering of CuO for efficient electrochemical reduction of CO2 to C2H4, SmartMat, 2022, 3, 194–205 CrossRef CAS.
- J. Li, C. Wang, D. Wang, C. Yang, X. Cui, X. J. Gao and Z. Zhang, A hexacoordinated Bi3+-based ellagate MOF with acid/base resistance boosting carbon dioxide electroreduction to formate, J. Mater. Chem. A, 2022, 10, 20018–20023 RSC.
- Y. Wang, L. You and K. Zhou, Origin of the N-coordinated single-atom Ni sites in heterogeneous electrocatalysts for CO2 reduction reaction, Chem. Sci., 2021, 12, 14065–14073 RSC.
- W. Zhang, M. Jiang, S. Yang, Y. Hu, B. Mu, Z. Tie and Z. Jin,
In situ grown CuOx nanowire forest on copper foam: a 3D hierarchical and freestanding electrocatalyst with enhanced carbonaceous product selectivity in CO2 reduction, Nano Res. Energy, 2022, 1, e9120033 CrossRef.
- W. Yang, Y. Zhu, J. Li, Z. Chen, F. Nosheen, Q. Zhang and Z. Zhang, Understanding the dehydrogenation mechanism over iron nanoparticles catalysts based on density functional theory, Chin. Chem. Lett., 2021, 32, 286–290 CrossRef CAS.
- Y.-S. Wei, M. Zhang, R. Zou and Q. Xu, Metal-Organic Framework-Based Catalysts with Single Metal Sites, Chem. Rev., 2020, 120, 12089–12174 CrossRef CAS PubMed.
- B. An, Z. Li, Y. Song, J. Zhang, L. Zeng, C. Wang and W. Lin, Cooperative copper centres in a metal–organic framework for selective conversion of CO2 to ethanol, Nat. Catal., 2019, 2, 709–717 CrossRef CAS.
- M. Hmadeh, Z. Lu, Z. Liu, F. Gándara, H. Furukawa, S. Wan, V. Augustyn, R. Chang, L. Liao, F. Zhou, E. Perre, V. Ozolins, K. Suenaga, X. Duan, B. Dunn, Y. Yamamto, O. Terasaki and O. M. Yaghi, New Porous Crystals of Extended Metal-Catecholates, Chem. Mater., 2012, 24, 3511–3513 CrossRef CAS.
- L. S. Xie, G. Skorupskii and M. Dincă, Electrically Conductive Metal-Organic Frameworks, Chem. Rev., 2020, 120, 8536–8580 CrossRef CAS PubMed.
- W. Yang, J. Li, X. Cui, C. Yang, Y. Liu, X. Zeng, Z. Zhang and Q. Zhang, Fine-tuning inverse metal-support interaction boosts electrochemical transformation of methanol into formaldehyde based on density functional theory, Chin. Chem. Lett., 2021, 32, 2489–2494 CrossRef CAS.
- Z. Zhao, J. Zhang, M. Lei and Y. Lum, Reviewing the impact of halides on electrochemical CO2 reduction, Nano Research Energy, 2023, 2, e9120044 CrossRef.
- Y. Zhu, Z. Gao, Z. Zhang, T. Lin, Q. Zhang, H. Liu, L. Gu and W. Hu, Selectivity regulation of CO2 electroreduction on asymmetric AuAgCu tandem heterostructures, Nano Res., 2022, 15, 7861–7867 CrossRef CAS.
- D. Du, Q. Geng, L. Ma, S. Ren, J.-X. Li, W. Dong, Q. Hua, L. Fan, R. Shao, X. Wang, C. Li and Y. Yamauchi, Mesoporous PdBi nanocages for enhanced electrocatalytic performances by all-direction accessibility and steric site activation, Chem. Sci., 2022, 13, 3819–3825 RSC.
- Z. Gao, C. Wang, J. Li, Y. Zhu, Z. Zhang and W. Hu, Conductive metal-organic frameworks for electrocatalysis: achievements, challenges, and opportunities, Acta Phys.-Chim. Sin., 2021, 37, 2010025 Search PubMed.
- Z. Meng, J. Luo, W. Li and K. A. Mirica, Hierarchical Tuning of the Performance of Electrochemical Carbon Dioxide Reduction Using Conductive Two-Dimensional Metallophthalocyanine Based Metal-Organic Frameworks, J. Am. Chem. Soc., 2020, 142, 21656–21669 CrossRef CAS PubMed.
- X.-F. Qiu, H.-L. Zhu, J.-R. Huang, P.-Q. Liao and X.-M. Chen, Highly Selective CO2 Electroreduction to C2H4 Using a Metal–Organic Framework with Dual Active Sites, J. Am. Chem. Soc., 2021, 143, 7242–7246 CrossRef CAS PubMed.
- R. M. Stolz, A. Mahdavi-Shakib, B. G. Frederick and K. A. Mirica, Host–Guest Interactions and Redox Activity in Layered Conductive Metal–Organic Frameworks, Chem. Mater., 2020, 32, 7639–7652 CrossRef CAS.
- V. Rubio-Giménez, M. Galbiati, J. Castells-Gil, N. Almora-Barrios, J. Navarro-Sánchez, G. Escorcia-Ariza, M. Mattera, T. Arnold, J. Rawle, S. Tatay, E. Coronado and C. Martí-Gastaldo, Bottom-Up Fabrication of Semiconductive Metal–Organic Framework Ultrathin Films, Adv. Mater., 2018, 30, 1704291 CrossRef PubMed.
- N. Wang, R. K. Miao, G. Lee, A. Vomiero, D. Sinton, A. H. Ip, H. Liang and E. H. Sargent, Suppressing the liquid product crossover in electrochemical CO2 reduction, SmartMat, 2021, 2, 12–16 CrossRef CAS.
- H. Wu, W. Zhang, S. Kandambeth, O. Shekhah, M. Eddaoudi and H. N. Alshareef, Conductive Metal-Organic Frameworks Selectively Grown on Laser-Scribed Graphene for Electrochemical Microsupercapacitors, Adv. Energy Mater., 2019, 9, 1900482 CrossRef.
- C. Chen, X. Yan, R. Wu, Y. Wu, Q. Zhu, M. Hou, Z. Zhang, H. Fan, J. Ma, Y. Huang, J. Ma, X. Sun, L. Lin, S. Liu and B. Han, Quasi-square-shaped cadmium hydroxide nanocatalysts for electrochemical CO2 reduction with high efficiency, Chem. Sci., 2021, 12, 11914–11920 RSC.
- A. Dutta, I. Zelocualtecatl Montiel, K. Kiran, A. Rieder, V. Grozovski, L. Gut and P. Broekmann, A Tandem (Bi2O3 → Bimet) Catalyst for Highly Efficient ec-CO2 Conversion into Formate: Operando Raman Spectroscopic Evidence for a Reaction Pathway Change, ACS Catal., 2021, 11, 4988–5003 CrossRef CAS.
- T. Chen, J.-H. Dou, L. Yang, C. Sun, N. J. Libretto, G. Skorupskii, J. T. Miller and M. Dincă, Continuous Electrical Conductivity Variation in M3(Hexaiminotriphenylene)2 (M = Co, Ni, Cu) MOF Alloys, J. Am. Chem. Soc., 2020, 142, 12367–12373 CrossRef CAS PubMed.
- F. P. García de Arquer, C.-T. Dinh, A. Ozden, J. Wicks, C. McCallum, A. R. Kirmani, D.-H. Nam, C. Gabardo, A. Seifitokaldani, X. Wang, Y. C. Li, F. Li, J. Edwards, L. J. Richter, S. J. Thorpe, D. Sinton and E. H. Sargent, CO2 electrolysis to multicarbon products at activities greater than 1 A cm−2, Science, 2020, 367, 661–666 CrossRef PubMed.
- W. Deng, L. Zhang, L. Li, S. Chen, C. Hu, Z.-J. Zhao, T. Wang and J. Gong, Crucial Role of Surface Hydroxyls on the Activity and Stability in Electrochemical CO2 Reduction, J. Am. Chem. Soc., 2019, 141, 2911–2915 CrossRef CAS PubMed.
- Y. Shi, M. Hou, J. Li, L. Li and Z. Zhang, Cu-Based Tandem Catalysts for Electrochemical CO2 Reduction, Acta Phys.-Chim. Sin., 2022, 38, 2206020 Search PubMed.
- X. Zhao, M. Huang, B. Deng, K. Li, F. Li and F. Dong, Interfacial engineering of In2O3/InN heterostructure with promoted charge transfer for highly efficient CO2 reduction to formate, Chem. Eng. J., 2022, 437, 135114 CrossRef CAS.
- J. Wu, X. Li, W. Shi, P. Ling, Y. Sun, X. Jiao, S. Gao, L. Liang, J. Xu, W. Yan, C. Wang and Y. Xie, Efficient Visible-Light-Driven CO2 Reduction Mediated by Defect-Engineered BiOBr Atomic Layers, Angew. Chem., Int. Ed., 2018, 57, 8719–8723 CrossRef CAS PubMed.
- N. Qiu, J. Li, H. Wang and Z. Zhang, Emerging dual-atomic-site catalysts for electrocatalytic CO2 reduction, Sci. China Mater., 2022, 65, 3302–3323 CrossRef CAS.
|
This journal is © The Royal Society of Chemistry 2023 |