DOI:
10.1039/D3FO02330C
(Review Article)
Food Funct., 2023,
14, 7799-7824
Revisiting carotenoids as dietary antioxidants for human health and disease prevention
Received
10th June 2023
, Accepted 8th August 2023
First published on 16th August 2023
Abstract
Humans are unique indiscriminate carotenoid accumulators, so the human body accumulates a wide range of dietary carotenoids of different types and to varying concentrations. Carotenoids were once recognized as physiological antioxidants because of their ability to quench singlet molecular oxygen (1O2). In the 1990s, large-scale intervention studies failed to demonstrate that supplementary β-carotene intake reduces the incidence of lung cancer, although its antioxidant activity was supposed to contribute to the prevention of oxidative stress-induced carcinogenesis. Nevertheless, the antioxidant activity of carotenoids has attracted renewed attention as the pathophysiological role of 1O2 has emerged, and as the ability of dietary carotenoids to induce antioxidant enzymes has been revealed. This review focuses on six major carotenoids from fruit and vegetables and revisits their physiological functions as biological antioxidants from the standpoint of health promotion and disease prevention. β-Carotene 9′,10′-oxygenase-derived oxidative metabolites trigger increases in the activities of antioxidant enzymes. Lutein and zeaxanthin selectively accumulate in human macular cells to protect against light-induced macular impairment by acting as antioxidants. Lycopene accumulates exclusively and to high concentrations in the testis, where its antioxidant activity may help to eliminate oxidative damage. Dietary carotenoids appear to exert their antioxidant activity in photo-irradiated skin after their persistent deposition in the skin. An acceptable level of dietary carotenoids for disease prevention should be established because they can have deleterious effects as prooxidants if they accumulate to excess levels. Finally, it is expected that the reason why humans are indiscriminate carotenoid accumulators will be understood soon.
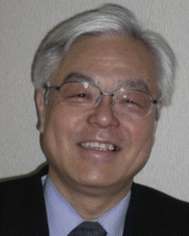 Junji Terao | Junji Terao, PhD is an Emeritus Professor of Tokushima University. He received his PhD at Kyoto University and worked at the Research Institute for Food Science Kyoto University, Oklahoma Medical Research Foundation, and National Food Research Institute MAFF Japan. In 1997, he joined the Department of Nutrition, Tokushima University School of Medicine. After the retirement in 2016, he worked at Konan Women's University for 6 years. His research interests are bioavailability and physiological function of dietary antioxidants and pathophysiological function of lipid peroxidation products. He has published more than 240 peer-reviewed scientific papers. |
1. Introduction
“Carotenoids” is a general term for the yellow, red, and orange pigments containing a long-chain hydrocarbon with conjugated double bonds. More than 1100 carotenoids are found in nature and are typically present as C-40-based tetraterpenoid secondary metabolites in the plant kingdom, although C-45- and C-50-based carotenoids are also distributed in bacteria.1 Carotenoids can be categorized as hydrocarbon carotenoids (carotenes and lycopene) and oxygenated carotenoids (xanthophylls) on the basis of the presence or absence of oxygen atoms in their structures.2 They are exogeneous nutrients for animals because animals cannot synthesize them de novo. Birds and fishes are known to accumulate various carotenoids in their body by oxidative modification after ingestion. In contrast, dietary carotenoids have a range of different fates in the bodies of mammals, including humans. In 1984, Goodwin3 proposed that mammals can be separated into three groups: indiscriminate accumulators, carotene accumulators, and carotene non-accumulators, on the basis of their patterns of absorption and accumulation of ingested carotenoids. Humans are unique indiscriminate accumulators and are believed to absorb more than 40 carotenoids as dietary components and accumulate them in the body indiscriminately. It was also confirmed that primates are uniquely distinguished by the wide range of carotenoids of different types and concentrations in their sera.4 It is noteworthy that six major carotenoids accumulate in human plasma and tissues: α-carotene, β-carotene, lycopene, β-cryptoxanthin, lutein, and zeaxanthin (Fig. 1).5,6
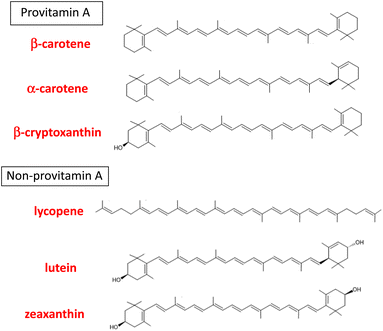 |
| Fig. 1 Structures of major six carotenoids detected in human plasma and tissues. | |
The major dietary sources of each carotenoid are as follows. α-carotene: carrots, winter squash and pumpkin; β-carotene: dark orange and green fruits and vegetables; lycopene: tomatoes and tomato products; β-cryptoxanthin: tropical fruits and sweet red peppers; lutein: leafy greens, corn and green vegetables; zeaxanthin: egg yolk, corn, corn meal, and leafy greens.7 The distribution of carotenoids in human plasma seems to reflect the composition of carotenoids ingested via the diet, especially fruit and vegetables.
In humans, the main biological function of dietary carotenoids is provitamin A activity. Among the six carotenoids mentioned above, α-carotene, β-carotene, and β-cryptoxanthin can act as provitamin A because they can release retinal by the central cleavage reaction with β-carotene 15,15′-oxygenase (BCO1) within epithelial cells during absorption in the small intestine. Nevertheless, a proportion of these three provitamin A carotenoids and the other three non-provitamin A carotenoids are absorbed from the small intestine without cleavage, and are transported into the blood circulation system in their original forms. Therefore, the biological functions of dietary carotenoids other than provitamin A activity have long attracted attention from the aspect of their health benefits. Such benefits include immunostimulatory function, promotion of intercellular gap junction formation, and adipocyte function, although the level of carotenoids in the blood may simply be an indicator of fruit and vegetable intake.
In the 1980s, Sies8 proposed the concept “oxidative stress”; that is, an imbalance of prooxidants that accelerate the production of reactive oxygen species (ROS) and antioxidants that attenuate ROS production. Oxidative stress was identified as a factor contributing to the etiology and progression of chronic diseases. In this context, the antioxidant activity of dietary carotenoids aroused much interest together with vitamin C and vitamin E because carotenoids were known to react readily with ROS. In the 2000s, the “oxidative stress” concept was revised to, “an imbalance between oxidants and antioxidants in favor of the oxidants, leading to a disruption of the redox signaling and control, and/or molecular damage”.9 In addition, novel metabolites other than retinal, which may affect cellular redox signaling, were discovered as metabolic products of carotenoids produced during intestinal absorption and blood circulation. Thus, the antioxidant activity of carotenoids has attracted renewed attention in relation to the role of food-derived factors in human health.10–12 This review article focuses on the six major dietary carotenoids and revisits their physiological functions as biological antioxidants from the viewpoint of health promotion and disease prevention.
2. Research background on carotenoids as dietary antioxidants
2.1 Progress of antioxidant research on carotenoids
Carotenoids are essential compounds for the survival of photosynthetic plants. These pigments coexist with chlorophylls in the thylakoid membranes of chloroplasts and protect plants from photodamage induced by the photodynamic action of chlorophylls.13 This is the reason why chlorophyll-rich leafy vegetables contain remarkable amounts of carotenoids. In fruits, carotenoid pigments act as attractants for pollinators and seed-dispersing animals after fruit ripening, in addition to functioning as protectants from photodamage in irradiated regions. Carotenoids also serve as precursors of phytohormones such as abscisic acid, and aroma components such as β-ionone.2,14
In 1968, Foote et al.15 discovered that β-carotene is capable of quenching highly reactive singlet molecular oxygen (1O2), a type of ROS that contributes to photooxidative damage in photosynthetic plants. Twenty years later, Di Mascio et al.16 found that lycopene exhibited the highest quenching rate constant with 1O2 among eight carotenoids including β-carotene, α-carotene, zeaxanthin, lutein, and β-cryptoxanthin. Thereafter, dietary carotenoids were frequently referred as powerful 1O2 quenchers that participate in protection against oxidative stress in the human body.17,18 Krinsky19 raised the possibility that carotenoids inhibit the free radical reaction of lipid peroxidation by scavenging lipid radicals.
In the 1980s, the potential roles of ROS and ROS-scavenging natural antioxidants attracted much attention in relation to the etiology of carcinogenesis. Several studies evaluated the roles of carotenoids in the molecular mechanism of carcinogenesis, including oxidative modification of genomic DNA.20,21 The results of Peto et al.22 implied that dietary β-carotene might exert its protective effect against human cancer by a mechanism other than its well-known provitamin A activity. In 1984, Burton and Ingold23 proposed that β-carotene is a unique radical-trapping type lipid antioxidant because it exhibits good radical-trapping capacity at lower oxygen partial pressures under physiological conditions. They also found that, at higher oxygen pressures and relatively high concentrations, β-carotene can act as a prooxidant by promoting the radical chain reaction of lipid peroxidation. Much attention was then paid to the antioxidant effect of dietary carotenoids in free radical-mediated lipid peroxidation.24,25 Terao et al.26,27 demonstrated that xanthophylls, like β-carotene, can act as chain-breaking antioxidants against lipid peroxidation in solution and in liposomal membranes. Concerning the possibility that ingested carotenoids exert antioxidant activity in the human body, low-density lipoprotein (LDL) derived from the plasma of human subjects consuming a diet supplemented with lycopene-rich tomato juice showed increased resistance to 1O2-induced LDL-cholesterol peroxidation, whereas this supplementation did not affect the free-radical chain reaction.28 Increased resistance of plasma LDL to metal ion-induced lipid oxidation and the suppression of a lipid peroxidation biomarker in the plasma were detected after dietary intake of tomato products for 3 weeks.29 The results of those studies implied that dietary carotenoids can improve the antioxidant defense of plasma LDL against lipid peroxidation. Because oxidized LDL is thought to participate in the incidence and progress of atherosclerosis leading to cardiovascular disease,30 it was proposed that dietary carotenoids can help to prevent atherosclerosis by acting as antioxidants in LDL particles.
2.2 Expectations and confusion about the chemopreventive effect of carotenoid supplementation
In the 1980s, epidemiological evidence strongly suggested that carotenoid intake may reduce the risk of lung cancer and certain other cancers.31,32 Several large-scale intervention studies were then carried out to determine whether supplementary intake of β-carotene exerted a preventive effect against the incidence of lung cancer (Table 1).
Table 1 Large-scale intervention trials examining the relationship between β-carotene supplementation and incidence of lung cancer
Trial |
Subject |
Dose |
Period |
Result |
Ref. |
y.: years old and RR: relative risk. |
Linxian study |
29 584 people (40–69 y.) living in Linxian, China |
Daily β-carotene (15 mg), vitamin E (30 mg) and selenium (50 mg) |
1986–1991 |
Significant difference in lowered total mortality (RR = 0.91), lower cancer rates (RR = 0.87) and lower stomach cancer rate (RR = 0.79) |
33
|
Physicians’ study |
22 071 physicians (40–84 y.) living in US |
β-Carotene (50 mg) on alternative days |
1982–1995 |
No significant difference in malignant neoplasms, cardiovascular diseases, or death from all diseases |
34
|
ATBC study |
29 133 male smokers (50–69 y.) living in southwestern Finland |
Daily β-carotene (20 mg) |
1985–1993 |
Significantly higher incidence of lung cancer (+18%) |
35
|
CARET trial |
18 314 current smokers and asbestos-exposed workers |
Daily β-carotene (30 mg) and retinyl palmitate (25 000 IU) |
1988–1998 |
Significantly excess lung cancer incidence (RR = 1.36) and lung cancer mortality (RR = 1.59) |
36
|
A major intervention trial involving approximately 30
000 people in Linxian County, China, was conducted from 1986 to 1991. The population in this region has one of the world's highest rates of esophageal/gastric cardia cancer and a persistently low intake of several micronutrients. Combined dietary supplementation with β-carotene, vitamin E, and selenium apparently reduced the risk of cancer in this population.33 In contrast, the Physicians’ study (1982–1995) targeting approximately 22
000 physicians in the U.S.A. showed that supplementation with β-carotene produced neither benefit nor harm in terms of the incidence of lung cancer and death from all causes.34 The results of both the alpha-tocopherol, beta-carotene cancer prevention (ATBC) study involving nearly 30
000 male smokers in southwestern Finland35 and the beta-Carotene and Retinol Efficacy Trial (CARET) involving approximately 18
000 men and women who were heavy cigarette smokers and men with occupational exposure to asbestos36 strongly indicated that β-carotene supplementation increased the rate of death by lung cancer. The CARET intervention was stopped 21 months early because of the possible harmful effect of daily consumption of β-carotene combined with retinyl palmitate.
Mayne et al.37 reported that up to 5 years’ daily supplementation with β-carotene (50 mg per day) produced an approximately 10-fold increase in the median plasma β-carotene concentration. In the ATBC study, the plasma β-carotene concentration was increased 15-fold (from 0.37 μM to 5.81 μM) by supplementation with β-carotene (20 mg per day) for 6.7 years.38 These phenomena indicated that excess β-carotene accumulated in the plasma of smokers and men with occupational exposure to asbestos after β-carotene supplementation, and therefore acted as a potential prooxidant, resulting in harmful effects and the development of lung cancer.39,40 In 2022, a systematic review on the role of β-carotene in primary chemoprevention of lung cancer reconfirmed that β-carotene supplementation may increase the risk of lung cancer.41 Thus, it was concluded that nutritional prevention of cancer through β-carotene supplementation should not be recommended.42
The “oxidative stress” concept attracted much attention in the field of clinical medicine in the 1990s. Together with vitamin E and vitamin C, carotenoids were expected to participate in the antioxidant network in the human body.43 However, the 5-year Medical Research Council/British Heart Foundation Heart Protection Study in which daily antioxidant vitamin supplementation (600 mg vitamin E, 250 mg vitamin C, and 20 mg β-carotene) was provided to approximately 20
000 adults in the United Kingdom did not provide any evidence for significant effects of antioxidant supplementation on vascular events and cancer incidence.44 Bjelakovic et al.45,46 conducted a systematic review and meta-analysis of randomized trials using antioxidant supplements for disease prevention, and proposed that supplementary intake of β-carotene, vitamin A, and vitamin E may increase all-cause mortality. They concluded that antioxidant supplementation has no preventive effect and may be harmful to human health at doses higher than the recommended daily allowances. Thus, the optimal source of antioxidants is from the diet, not from antioxidant supplements in pill or tablet form.47 In 2013, the Department of Health and Human Services (HHS) and the National Institutes of Health (NIH) in the U.S.A. warned of the deleterious effects of high-dose antioxidant supplements in some cases.48
Increasing the consumption of fresh fruits and vegetables is a favorable strategy to prevent non-communicable diseases including cancers, cardiovascular disease, diabetes, and chronic respiratory diseases.49,50 The inverse association between fruit and vegetable intake and all-cause mortality was recently reconfirmed in a study involving nearly 100
000 Japanese people.51 The HHS/NIH proposed three reasons for the discrepancy between beneficial and deleterious effects as follows: the beneficial health effect of fruits and vegetables may be caused by other substances or other dietary factors, the effects of the large doses used in supplements may differ from those of smaller amounts consumed in foods, and differences in the chemical composition of antioxidants in foods versus those in supplements may influence their effects.48 In the case of carotenoids, excess β-carotene intake by ingesting supplements may induce its prooxidant effect, leading to enhanced oxidative stress in the human body. In all intervention studies, only β-carotene was provided as the carotenoid supplement, whereas foods contain a variety of carotenoids. In any case, the mechanism of action of the health benefits of dietary carotenoids remains unclear, except for their provitamin A activity.
Specific carotenoids derived from fruit and vegetables might be effective in some diseases. In this sense, observational studies suggested that lycopene may act as a preventive factor against prostate cancer.52 The role of lutein and zeaxanthin in macular pigments attracted attention in connection with the prevention of age-related macular regeneration (AMD).53 Dietary carotenoids have also been implicated in the protection of skin against photoaging because human skin is known to accumulate these pigments from foods.54
2.3 Discovery of novel carotenoid-metabolic pathways and interest in the function of novel metabolites
In 1965, Olson and Hayaishi55 and Goodman and Huang56 independently discovered the enzyme β-carotene 15,15′-oxygenase (BCO1) in the rat liver and intestine. This enzyme catalyzes the cleavage of the central region of β-carotene symmetrically to produce retinal. Although it was proposed that eccentric cleavage to produce apocarotenoids with different chain lengths may occur in the metabolic pathway of β-carotene, a series of reports refuted the participation of eccentric cleavage in the mechanism of vitamin A formation and emphasized that retinal is the sole product of β-carotene cleavage.57 In 2001, Kiefer et al.58 discovered a novel enzyme from mouse cDNA that catalyzes the asymmetric oxidative cleavage at the 9′,10′ double bond of β-carotene to yield β-apo-10′-carotenal and β-ionone. This enzyme, designated as β-carotene 9′,10′-oxygenase 2 (BCO2), was found to cleave lycopene to yield apo-10′-lycopenoids.58,59 BCO2 prepared from ferret, an animal model of human carotenoid metabolism, was also found to cleave xanthophylls such as lutein, zeaxanthin, and β-cryptoxanthin, resulting in several isomers of 3-hydroxy-apo-10′-carotenal and 3-hydroxyionones.60
Khachik et al.61 earlier showed that oxidation products, such as 3-hydroxy-β,ε-carotene-3′-one, accumulate in human plasma as oxidative metabolites of lutein and zeaxanthin. These ketocarotenoids, as well as apocarotenoids and apolycopenoids, may have specific biological activities in relation to human health, as they can act as powerful electrophilic compounds.62,63 Apolycopenoids can activate the electrophile/antioxidant response element (EpRE/ARE) system by releasing NEF-E2-related factor 2 (Nrf2).64 This event may promote biological antioxidant defenses by increasing the expression of antioxidant enzymes, as described later in this review. It is currently suggested that the mechanism of action of carotenoids involves an indirect effect of their oxidative products to promote antioxidant enzyme activity, as well as their inherent 1O2-quenching and radical-trapping activities.
3. Absorption and metabolism of dietary carotenoids
3.1 Absorption of carotenoids in the intestine
Yonekura and Nagao65 and von Lintig et al.66 described a chain of processes from the ingestion of carotenoid-containing foods to the absorption of each carotenoid by intestinal epithelial cells. After ingestion of carotenoid-containing foods, carotenoids are released from the food matrix in the digestive tract. The lipophilic carotenoids incorporate into the lipid phase, which is emulsified in the stomach, and are then transferred to mixed micelles formed by the action of bile salts, biliary phospholipids, dietary lipids, and their lipase-hydrolysis products. The mixed micelles migrate to the brush border membranes of the lumen and then each carotenoid is absorbed by intestinal absorptive cells. In fruits and vegetables, xanthophylls are frequently present as their fatty acid esters, in which the hydroxy group at the β-ionone ring is esterified by various free fatty acids.67 These esters are mostly hydrolyzed by pancreatic carboxyl lipase during the digestion process.68,69 Thus, xanthophylls, that is, β-cryptoxanthin, lutein, and zeaxanthin, are present as free forms in human plasma. Fats and oils are good carriers for lipophilic carotenoids in the digestive tract, and they accelerate micelle formation by inducing the secretion of pancreatic lipase and bile acids. In fact, ingestion of fresh vegetables with larger proportions of oil in the dressing was shown to improve carotenoid absorption.70
Class B scavenger receptors, SR-B1 and CD36, contribute to the absorption of dietary carotenoids in the epithelium.71–73 These proteins are expressed in the intestine and facilitate the uptake of carotenoids by enterocytes without energy expenditure. After intestinal absorption, carotenoids are incorporated into triacylglycerol-rich lipoproteins (chylomicrons) and secreted into the lymphatic system. Then, they are absorbed into the liver as chylomicron remnants and finally distributed around the whole body via the carotenoid-containing lipoproteins secreted from the liver.
3.2 Metabolism of carotenoids
Provitamin A carotenoids such as β-carotene are partly converted to vitamin A in the intestine.66 That is, they are cleaved at the central position (between the 15- and 15′-position) to yield two molecules of retinal by the action of BCO1 located in the intestinal epithelial cells, followed by retinal dehydrogenase-dependent reduction to retinol (Fig. 2).
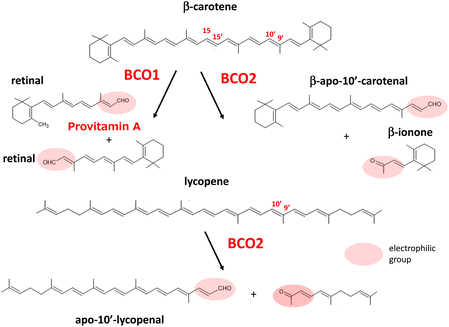 |
| Fig. 2 Oxidative cleavage of double bonds in carotenoids by BCO1 and BCO2. | |
Retinol is finally circulated in the blood stream after binding with retinol binding protein-4 (PBP4) in the liver. Human BCO1 mRNA has also been detected in the liver, kidney, and several peripheral tissues, in which BCO1 may play a role in local vitamin A synthesis.74 Dietary supply of provitamin A carotenoids at excess levels does not lead to symptoms of hypervitaminosis because vitamin A production is elegantly regulated by a negative feedback mechanism corresponding to dietary intake.75
BCO2, which catalyzes the cleavage between the 9′ and 10′ position of β-carotene resulting in β-apo-10′-carotenal, displays broad substrate specificity for carotenoids. Thus, this enzyme catalyzes the conversion of non-provitamin A carotenoids including acyclic lycopene as well as lutein and zeaxanthin as shown in Fig. 2. BCO2 is expressed in the intestine and several additional tissues, but its role in the metabolism of dietary carotenoids remains undefined. Interestingly, BCO2 is a mitochondrial enzyme associating with the inner membrane, in contrast to BCO1, which is a cytosolic enzyme.76 Experiments on BCO2-deficient mice implied that BCO2 protects against carotenoid-induced oxidative damage in hepatic mitochondria.77 In experiments using a human cell line, BCO2 was suggested to prevent carotenoid-caused oxidative stress and triggering of the apoptotic pathway in mitochondria by acting as a carotenoid scavenger and gatekeeper for the apoptotic pathway.78 BCO2 systemic knock out-mice showed oxidative stress-induced perturbation of mitochondrial function in the liver79 and the hypothalamus.80 Therefore, BCO2 seems to be essential to regulate macronutrient mitochondrial metabolism in rodents.81 Human BCO2 may also affect normal mitochondrial function, although its activity has not yet been fully clarified.
Babino et al.82 demonstrated that oxidative stress induces the expression of the gene encoding human BCO2, and proposed that the preventive function of BCO2 against oxidative stress is conserved in primates. The induction of BCO2 in human tissues may prevent excessive accumulation of carotenoids and explain the observation that carotenoid levels decrease in oxidative stress-related chronic disease states.66 However, Li et al.83 found that human BCO2 in the retina is inactive as a xanthophyll-cleavage enzyme, in contrast to the high activity of mouse BCO2. In contrast, the products of the reaction between lycopene and BCO2 showed antioxidant properties, in that they activated Nrf2 to induce antioxidant enzymes including heme oxygenase-1 (HO-1), NAD(P)H:quinone oxidoreductase-1 (NQO1), glutathione S-transferase (GST), and glutamate–cysteine ligases in human bronchial cells.84 At present, the levels of BCO2 activity and its physiological functions in humans with respect to the metabolism of dietary carotenoids are still unclear.
In 1992, Khachik et al.61 detected 3′-hydroxy-ε,ε-caroten-3-one, 3-hydroxy-β,ε-caroten-3′-one, and ε,ε-carotene-3,3′-dione, which were formed by the oxidation of lutein and zeaxanthin in human plasma. This implied that oxidative metabolism of xanthophylls occurs at their hydroxy group of the β-ionone ring in human tissues. Nagao et al.85 confirmed this oxidative modification of xanthophylls in the mouse liver. Their results suggested that this oxidative activity to convert the 3-hydroxy β-end group to a keto group also exists in humans because the same ketocarotenoids produced by oxidation of lutein, zeaxanthin, and β-cryptoxanthin in the mouse liver were detected in human plasma after ingestion of mandarin orange juice (Fig. 3).
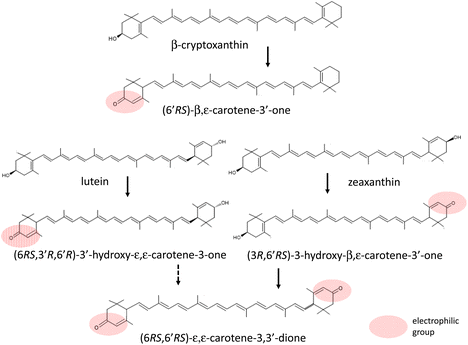 |
| Fig. 3 Proposed mechanism for oxidative modification to yield ketocarotenoids from xanthophylls (modified from ref. 85). | |
Microsomal NAD+-dependent dehydrogenase is likely to be responsible for this oxidative modification, although the enzyme itself has not yet been characterized.
3.3 Accumulation of dietary carotenoids in the human body
Böhm et al.86 estimated that a total plasma carotenoid concentration lower than 1000 nmol L−1 is associated with a higher risk of chronic diseases. They also clarified from the data on the average daily intake of carotenoids that plasma concentrations reflecting normal carotenoid intake in a healthy varied diet are ∼1725 nmol L−1 (total carotenoids), comprising 100 nmol L−1 α-carotene, 500 nmol L−1 β-carotene, 600 nmol L−1 lycopene, 230 nmol L−1 β-cryptoxanthin, and 330 nmol L−1 lutein and zeaxanthin. A variety of factors including diet, sex, age, and body mass index affect the plasma concentration of each carotenoid.87 Habitual alcohol drinking and cigarette smoking adversely affect plasma carotenoid concentrations.90 The carotenoid concentrations were found to be significantly higher in the liver (∼16
500 nmol kg−1), adrenal gland (9400 ± 7800 nmol kg−1), and testis (∼87
550 nmol kg−1) than in the kidney (3050 ± 4210 nmol kg−1) and lung (1905 ± 2820 nmol kg−1).86 Lycopene accounts for the majority of carotenoids in the testis, although β-carotene is the major carotenoid in other tissues.88 Lutein and zeaxanthin uniquely accumulate at the macula, where they are collectively called macular pigment.89 Once they accumulate in each tissue, carotenoids are speculated to be excreted from the body with and without metabolic conversion. The details of the excretion process are not yet known. The half-lives of carotenoids in human plasma were reported to be 76 days for lutein, 45 days for α-carotene, 39 days for β-cryptoxanthin, 38 days for zeaxanthin, 37 days for β-carotene, and 26 days for lycopene in healthy adult women,90 and 33–61 days for zeaxanthin and lutein, less than 12 days for β-carotene, α-carotene, and β-cryptoxanthin, 12–33 days for lycopene in healthy men.91
4. Mechanism of antioxidant activity of carotenoids
4.1 General comments
The antioxidant activity of carotenoids is explained by two mechanisms, one of which involves direct scavenging or quenching of ROS and lipid radicals. The other is indirect promotion of the antioxidant defense system via their involvement in cellular redox signaling pathways. 1O2 quenching is well characterized as a representative mechanism of carotenoids that function as biological antioxidants to regulate oxidative stress. It should be noted that carotenoids may also act as prooxidants by accelerating the radical chain reaction of lipid peroxidation when they react with oxygen radicals or lipid radicals under specified conditions. In the indirect action mechanism, electrophilic apocarotenoids, generated by the reaction of BCO2 with parent carotenoids, participate in a signal transduction pathway inducing Nrf2 activation. Ketocarotenoids derived from the oxidative metabolism of xanthophylls may also contribute to this pathway because ketocarotenoids possess electrophilic properties owing to their α,β-unsaturated carbonyl structure. The relationship between the site of oxidative damage and the location of carotenoids within the cells and extracellular fluids seems to affect the efficacy of carotenoids as biological antioxidants.
4.2 Generation of singlet oxygen in biological systems
The presence of 1O2 means that an oxygen molecule has been excited to the singlet state (spin quantum number S = 0) from the ground state triplet oxygen molecule (3O2: O2(3Σ−g)) by energy transfer. There are two species of 1O2: O2(1Σ+g) and O2(1Δg). Long-lived O2(1Δg) is probably responsible for the oxygenation reaction in biological systems because O2(1Σ+g) is short-lived owing to its rapid transition to O2(1Δg).921O2 attacks the electron-rich π-bond of organic compounds because this molecule possesses an empty anti-binding molecular orbital
. The reaction with olefines containing allylic hydrogen yields allylic hydroperoxide by an ene reaction, whereas endoperoxide is produced by [4 + 2] cycloaddition of cis 1,3-diene (Fig. 4).93,94
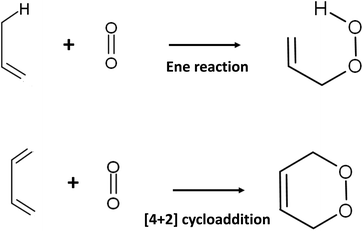 |
| Fig. 4 Oxygenation reaction of 1O2 with olefines and cis 1,3-diene. | |
1O2 can be produced by either light-dependent or light-independent (dark reaction) processes in biological systems.95 Type II photosensitized oxidation is responsible for the production of 1O2 in the light-dependent process. That is, 1O2 is generated from ground-state 3O2 by ultraviolet-A (UV-A) or visible light energy transfer through the photodynamic action of the sensitizer, as shown in Fig. 5.96
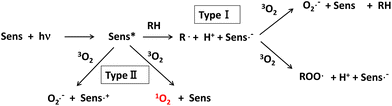 |
| Fig. 5 Mechanism of reactive oxygen species (ROS) generation in photosensitized oxidation reaction. Sens: photosensitizer, Sens*: excited photosensitizer, hν: light energy, 3O2: triplet state oxygen molecule, 1O2: singlet state excited oxygen molecule, RH: organic compound, ROO˙: peroxyl radical, O2˙−: superoxide anion radical. | |
An excited sensitizer reacts with organic compounds in type I photosensitized oxidation, while it reacts with 3O2 to yield 1O2 by energy transfer or superoxide anion (O2˙−) by electron transfer in the type II reaction.97,98 Riboflavin,99N-formyl-kynurenine,100,101 which is a metabolic intermediate of tryptophan, and chlorophylls and their related porphyrin compounds such as pheophytin and pheophorbide102–104 are frequently referred to as natural photosensitizers inducing photooxidation in biological systems. Psoralene and its related furocoumarins derived from citrus fruits such as oranges and grapefruits are potential photosensitizers leading to photo-carcinogenesis105 and are believed to act as type II sensitizers because they can produce 1O2 under UV-A irradiation.106,107 Further research is required to understand the effect of citrus consumption on the risk of photo-carcinogenesis,108 although the daily intake of psoralene seems to be below the safety threshold.109
Squalene hydroperoxides, which are the products of 1O2 oxygenation of squalene, were detected in the human skin surface after exposure to sunlight110 or UV-A radiation.111 It was suggested that squalene functions to prevent light-induced lipid peroxidation in human skin surface by acting as the first target of 1O2.112 Nevertheless, squalene hydroperoxides may cause oxidative damage in the skin.113 Extracellular matrix proteins114 and 3-hydroxypyridine derivatives115 are proposed to act as endogenous photosensitizers in photo-oxidative damage in human skin cells. Coproporphyrin produced from cutaneous Propionibacterium acnes was found to generate 1O2 by acting as a type II photosensitizer on the skin surface under exposure to UV radiation.116
Two major processes are proposed for light-independent 1O2 generation in biological systems.117,118 Firstly, the so-called Russell mechanism is the autocatalytic decomposition of peroxyl radicals (RCOO˙) involving cyclization via a tetraoxide (RCOO–OOCR) intermediate (eqn (1)).119
| 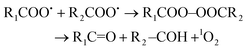 | (1) |
Direct evidence for the generation of 1O2 from lipid peroxyl radicals was provided using 18O-labeled linoleic acid hydroperoxide120 or cholesterol hydroperoxide121 and near-infrared emission analysis. Generation of 1O2 from lipid peroxyl radicals by the Russell mechanism implies that radical chain lipid peroxidation progresses together with the generation of both 1O2 and chain-propagating lipid peroxyl radicals. Miyamoto et al.122 indicated that the reaction of lipid hydroperoxides with peroxynitrite leads to 1O2 generation through the formation of lipid peroxyl radicals and the tetraoxide intermediate according to the Russell mechanism. They also suggested that cytochrome c-promoted phospholipid oxidation yields 1O2 from an excited triplet carbonyl intermediate, as well as from lipid peroxyl radicals via the Russell mechanism.123 Interestingly, Mano et al.124 recently demonstrated that chemically and enzymatically nascent excited carbonyls generate 1O2via energy transfer to 3O2. The alternative mechanism is a reaction of hypochlorous acid (HOCl) with hydrogen peroxide (H2O2) (eqn (2)).125
| 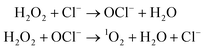 | (2) |
Thus, excited 1O2 is partly generated by the myeloperoxidase reaction in phagocytosing leukocytes. It was also reported that linoleic acid hydroperoxide can generate 1O2 by a reaction with HOCl.126 Notably, haloamines of amino acids and polyamines, especially bromoamines, were found to generate 1O2 in a reaction with H2O2.1271O2 may be readily generated during the inflammation process because inflammation is tightly linked with phagocytosis by leucocytes. The 1O2 generated during inflammation can lead to serious biological defects.
4.3 Reactions of 1O2 with biomolecules and their consequences
The reaction of 1O2 with unsaturated fatty acids produces isomeric hydroperoxides via an ene reaction mechanism.128 The radical chain reaction of lipid peroxidation also produces isomeric hydroperoxides from unsaturated fatty acids. However, the isomeric composition of hydroperoxides derived from 1O2 oxygenation is different from that of those produced by the radical chain reaction because 1O2 oxygenation is a non-radical reaction.129 In the case of 1O2 oxygenation with linoleic acid (octadeca-9Z,12Z-dienoic acid), 1O2 attacks the double bond-constituting carbon at either the 9- and 10-position or the 12- and 13-position to yield four hydroperoxyoctadecadienoic acid (HpODE) isomers, 9-HpODE, 10-HpODE, 12-HpODE, and 13-HpODE, by the transfer of allylic hydrogen and the shift of the double bond to the adjacent position (Fig. 6).
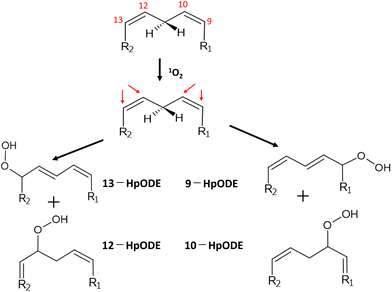 |
| Fig. 6 Production of isomeric hydroperoxides by 1O2 oxygenation of linoleic acid. R1 = (CH2)7–COOH, R2 = (CH3)4–CH3. HpODE: hydroperoxyoctadecadienoic acid. | |
The rate constant of 1O2 oxygenation with unsaturated fatty acids (kr ≃ 105 M−1 s−1) is much larger than that of the radical chain reaction (kr ≃ 102 M−1 s−1), whereas 1O2 oxygenation terminates without continuation in a chain.130 Umeno et al.131,132 reported that 10-hydroxyoctadedienoic acid (10-HODE) and 12-HODE, which are specifically derived from 1O2 oxygenation of linoleic acid, were detected in human plasma after reduction and saponification treatments. They suggested that these HODEs are potential biomarkers for the early detection of type 2 diabetes because monocytes seem to be recruited to adipose cells where myeloperoxidase is activated to yield 1O2 during hyperglycemia.132
Cholesterol is an essential constituent of cellular and subcellular membrane lipids. The radical chain reaction scarcely occurs with cholesterol because cholesterol lacks double allyl hydrogens to be eliminated. Nevertheless, this molecule can be a target of 1O2 oxygenation owing to the presence of a double bond between the 5-position and 6-position carbons.130 The rate constant for the reaction of 1O2 with the double bond of cholesterol does not substantially differ from that for the reaction of 1O2 with the cis-double bond of unsaturated fatty acids.133 This means that 1O2 can attack cholesterol and unsaturated fatty acids equally, resulting in isomeric hydroperoxycholesterols (Chol-OOHs). Fig. 7 shows the pathway of 1O2 oxygenation of cholesterol.
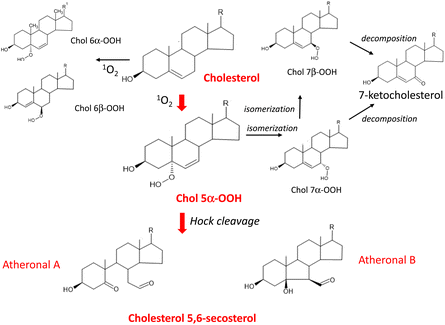 |
| Fig. 7 Pathway of 1O2 oxygenation of cholesterol leading to the production of atheronal A and B. R = C8H17. | |
Cholesterol 5α-hydroperoxide (Chol 5α-OOH), which is produced by an ene reaction at the 5,6-double bond position134 was found to serve as a precursor of cholesterol 5,6-secosterol A and B.135 These aldehydic cholesterols are called “atheronals” because they are frequently detected in atherosclerotic lesions, and are suggested to participate in the etiology of cardiovascular diseases.136 Because of their high electrophilicity, aldehydic cholesterols and ketocholesterols can exert potential biological activity by irreversible covalent modification of proteins.137 Therefore, cholesterol may function as a target molecule in 1O2-dependent lipid peroxidation occurring in biological systems.
Ferroptosis is a nonapoptotic form of cell death and characterized as the occurrence of iron ion- and ROS-dependent lipid peroxidation.138 Glutathione peroxidase-4 (GPX-4) occupies an essential position in the mitigative cascade of ferroptotic cell death by reducing phospholipid hydroperoxides.139 Among all the GPX isoforms, only GPX-4 reduces Chol-OOH to hydroxycholesterol (Chol-OH).140 Chol-OOH is inferior to unsaturated fatty acid hydroperoxides and their esterified phospholipid hydroperoxides as the substrate for GPX-4.141 The cellular level of lipophilic antioxidants including vitamin E may regulate this type of programmed cell death.142 Homma et al.143 showed that 1O2 can induce ferroptosis in mouse hepatoma cells exposed to a chemical 1O2 generator. Therefore, the role of dietary carotenoids and vitamin E in 1O2 quenching during ferroptotic cell death will be of much interest.
1O2 and the hydroxyl radical (˙OH) are major oxidants of cellular DNA that lead to deleterious effects.144,1451O2 is a key player contributing to oxidative DNA damage in light-irradiated human skin, as described by Di Mascio et al.146 Among the four nucleobases, guanine shows remarkedly high activity toward 1O2, followed by cytosine, adenine, and then thymine.147 The rate constants for guanosine and deoxyguanosine are ∼106 M−1 s−1, whereas those of other nucleosides are 103–104 M−1 s−1, indicating that guanine is the sole target in 1O2 oxygenation of DNA and other nucleic acids.147 Guanine contains electron-rich conjugated double bonds between the 4- and 5-carbons and the 7- and 8-carbons, so it readily reacts with 1O2via a [4 + 2] Diels–Alder-type cycloaddition reaction. 4,8-Endoperoxide is formed as an intermediate of 1O2 oxygenation of the imidazole ring, resulting in 8-hydroperoxydeoxyguanosine (8-OOHGuo). Finally, 8-oxodeoxyguanosine (8-oxoGuo) appears after the reduction of hydroperoxy group of 8-OOHGuo (Fig. 8).148,149
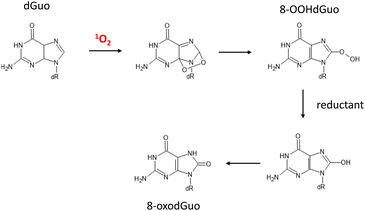 |
| Fig. 8 Degradation pathway of deoxyguanosine induced by 1O2 oxygenation. dR = 2-deoxyribose. dGuo: deoxyguanosine, 8-OOHdGuo: 8-hydroperoxy-deoxyguanosine, 8-oxodGuo: 8-oxo-7,8-dihydroguanosine. | |
Among the amino acids constituting functional proteins, cysteine, histidine, methionine, tryptophan, and tyrosine are known to react with 1O2 specifically (Fig. 9).150
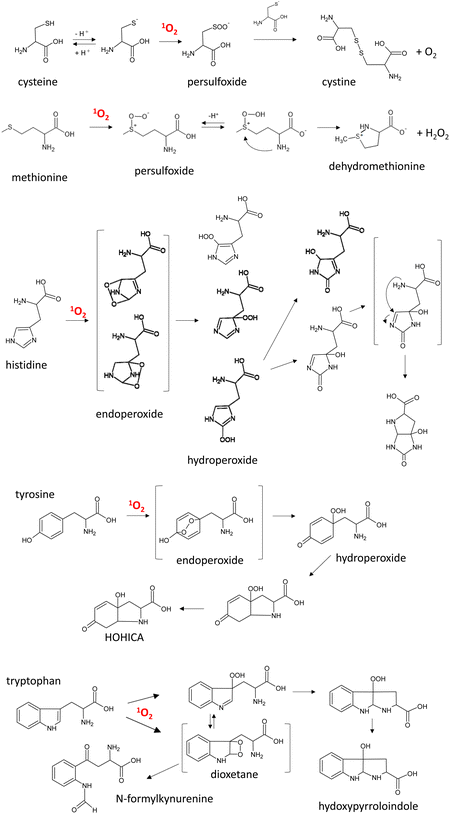 |
| Fig. 9 Main pathways for 1O2 oxygenation of amino acids. HOHICA: 3-a-hydroxy-6-oxo-2,3,3a,6,7,7a-hexahydro-1H-indol-2-carboxylic acid. | |
The rate constants of 1O2 oxygenation of these amino acids are reported to be approximately 106–107 M−1 s−1.146,151 In solution, 1O2 oxygenation of cysteine is proposed to produce a persulfoxide intermediate and then a disulfide via a reaction with another unprotonated thiol.151,152 In the case of methionine, persulfoxide is the first intermediate, and a free amino group attacks this intermediate to generate dehydromethionine and H2O2 at neutral pH.153 Histidine reacts with 1O2, yielding endoperoxides by cycloaddition of 1O2 across the 2,4- and 2,5-carbons of the imidazole ring.154 Rearrangements of these endoperoxides give rise to three isomeric hydroperoxides. Their subsequent decomposition followed by the nucleophilic attack of the α-amino group produce bicyclic products.155,1561O2 reacts with tyrosine via Diels–Alder [4 + 2] cycloaddition to the phenolic ring, producing 1,4-endoperoxide, and subsequent opening of the endoperoxide structure yields labile hydroperoxide.157,158 Then, bicyclic hydroperoxide is formed by Michael-type addition of the free amino group to the phenolic ring. This cyclization reaction does not occur in the case of tyrosine-containing peptides. The 1O2 oxygenation products of tryptophan are N-formylkynurenine and 3-hydroxypyrroloindole.159N-Formylkynurenine is a key metabolite of tryptophan metabolism in biological systems, and it is generated by the enzyme indoleamine 2,3-dioxygenase 1 (Ido1). This enzyme is expressed in arterial endothelial cells, where it contributes to the regulation of blood pressure during inflammation. It was recently demonstrated that Ido1 generates 1O2 in the presence of H2O2 to yield 3-hydroperoxypyrroloindole, which decreases blood pressure via oxidation of a specific cysteine residue of endothelial PKG1a (protein kinase G1a).160 This means that 1O2 derived from the enzymatic reaction serves as a redox signal molecule in the artery under inflammation conditions.161 It is expected that the roles of 1O2 in pathophysiological conditions will be resolved in the near future.
In terms of the reaction of proteins with 1O2, N-formylkinurenine was detected among the reaction products in UV-A-irradiated human lens epithelial cells in the presence of porphyrin.162 It was confirmed that methionine, histidine, and tryptophan residues are selectively oxidized by the reaction of 1O2 with lysozymes.163 However, methionine, histidine, and tryptophan, but not tyrosine, are oxidized in 1O2 oxygenation of cytochrome c.164 Crosslinking between certain residues including histidine and lysine occurs during the reaction of 1O2 with proteins.164,165 The 1O2 oxygenation of proteins is more complex than that of other biomolecules, and thus, many aspects of these reactions remain obscure.
4.4 Singlet oxygen quenching mechanism
Carotenoids seem to exert a potential role in human health and disease by acting as powerful antioxidants against 1O2.166 The antioxidant effect of carotenoids against 1O2 is based on the physical quenching of 1O2 (eqn (3)) and a chemical reaction with 1O2 (eqn (4)).
Physical quenching:
| 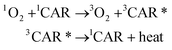 | (3) |
Chemical reaction:
| 1O2 + 1CAR → reaction products. | (4) |
In physical quenching, 1O2 is deactivated to the ground state oxygen molecule (3O2) by energy transfer from 1O2 to the ground state carotenoid molecule (1CAR). The resulting excited triplet state carotenoid molecule (3CAR*) returns to the original 1CAR by releasing its excited energy to the exogeneous environment as heat energy.167 In the chemical reaction, 1O2 reacts with carotenoids to yield oxygenated products. For example, the addition of 1O2 to the 5- and 8-positions of β-carotene generates β-carotene 5,8-endoperoxide.168,169 The rate constant of the physical quenching reaction (kq) is about 1000 times larger than that of the chemical reaction (kr), indicating that one carotenoid molecule can quench about 1000 molecules of 1O2 efficiently prior to its consumption by the chemical reaction with 1O2. In solution, the rate constant of the physical quenching reaction is close to that of the diffusion-controlled reaction (∼1010 M−1 s−1) and 30–100 times larger than that of a representative lipophilic antioxidant, α-tocopherol.170 Using a competitive reaction method, Aizawa et al.171 confirmed the superiority of dietary carotenoids to α-tocopherol in terms of their ability to quench 1O2.
Table 2 shows the rate constants of 1O2 quenching by major carotenoids in solution and liposomal membranes.16,167,172 Because nine or more conjugated double bonds are required for 1O2 quenching, neither retinol nor retinoic acid serve as 1O2 quenchers.173 Di Mascio et al.16 clarified that lycopene has the highest 1O2-quenching ability in solution. However, the 1O2-quenching activity of each carotenoid is significantly decreased in the liposomal model membrane system because the mobility of carotenoids is restricted when located in the lipid bilayer of the liposome structure.174 This means that the 1O2-quenching effect of carotenoids is unexpectedly low in biological systems as compared with that in solution because lipophilic carotenoids are presumed to be concentrated in the phospholipid bilayer of cellular and subcellular biomembranes. Carotenoids contain a long-chain hydrocarbon that stretches in lipid bilayer membranes,175 and polar carotenoids such as xanthophylls have their polar group anchored in the opposite polar zone of the membrane.176 The difference in orientation in a model membrane between β-carotene and zeaxanthin was pointed out by Cerezo et al.177 Bosio et al.178 emphasized that intracellular 1O2 is not efficiently quenched by β-carotene in β-carotene-incorporated mammalian cells. Thus, the efficiency of carotenoids as 1O2 quenchers in vivo remains a subject of argument. Nevertheless, carotenoids present in biomembranes together with α-tocopherol are likely to inhibit 1O2-dependent oxidative damage in vivo.179
Table 2 Rate constants for 1O2 quenching reaction by carotenoids
Carotenoids |
k
q (×108 M−1 s−1) |
Homogenous solvent |
Micelle solution |
DPPCb liposomes |
EtOH/CHCl3/H2Oa benzene |
RBc |
PBAd |
“—”: not determined. EtOH/CHCl3/H2O (50/50/1, v/v/v). DPPC: dipalmitoylphosphatidylcholine. Water-soluble rose bengal (RB) was used as type II photosensitizer. Lipid soluble 1-pyrenebutyric acid (RBA) was used as type II photosensitizer. |
Lycopene |
310 |
170 |
20 |
24 |
23 |
α-Carotene |
190 |
120 |
— |
— |
— |
β-Carotene |
140 |
130 |
24 |
23 |
25 |
Zeaxanthin |
100 |
120 |
25 |
2.3 |
1.7 |
Lutein |
80 |
66 |
33 |
1.1 |
0.82 |
β-Cryptoxanthin |
60 |
— |
— |
— |
— |
4.5 Reaction with free radicals and antioxidant/prooxidant effects
It has been suggested that carotenoids can act as antioxidants by scavenging free radicals, which induce the free radical-mediated radical chain reaction of lipid peroxidation.180 Carotenoids are proposed to inhibit this chain reaction efficiently at low-oxygen pressure in biological environments.23,181 In the lipid phase, the radical chain reaction occurs via chain-initiating radicals (X˙) and propagates until chain-propagating lipid peroxyl radicals (LOO˙) disappear from this phase. Chain-breaking antioxidants can stop the chain reaction by scavenging LOO˙. Carotenoids react with LOO˙ at the position of conjugated double bonds to yield the radical adduct of the carotenoid and LOO˙. This carbon radical (LOO-CAR˙) is subject to resonance stabilization at low oxygen pressure, so that the chain-termination reaction prevails over the chain-propagation reaction (Fig. 10).
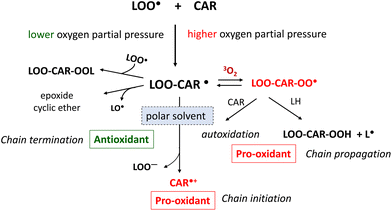 |
| Fig. 10 Antioxidant and prooxidant mechanisms of carotenoids in their radical trapping reaction. CAR: carotenoid, LH: unsaturated lipids, LO˙: lipid alkoxyl radical, LOO˙: lipid peroxyl radical, LOO-CAR˙: lipid peroxyl-carotene radical, LOO-CAR-OO˙: lipid peroxyl-carotene peroxyl radical, LOO−: lipid peroxyl anion, CAR˙+: carotenoid cation radical. | |
Carotenoids were earlier reported to inhibit liposomal lipid peroxidation significantly by scavenging chain-propagating LOO˙.27 However, the antioxidant activity of carotenoids as free radical scavengers was found to be much lower than that of α-tocopherol.182,183 Furthermore, assuming that the concentration of α-tocopherol is higher than that of carotenoids in cellular and subcellular membranes, it is reasonable to assume that carotenoids are significantly inferior to α-tocopherol as free radical scavengers against the radical chain reaction of lipid peroxidation occurring in biomembranes.
Carotenoids may change from antioxidants to prooxidants under increased oxygen partial pressure. Under higher oxygen partial pressure, LOO-CAR˙, which is generated by the addition reaction of a carotenoid with LOO˙, reacts with the ground state oxygen molecule (3O2) to yield a peroxyl radical (LOO-CAR-OO˙). These radicals abstract a hydrogen molecule from unsaturated lipids to yield a lipid radical (L˙), thereby accelerating the chain-propagation reaction of lipid peroxidation. El-Agamey and McGarvey184 showed that the addition reaction of a carotenoid with LOO˙ progresses differently depending on the properties of the reaction solvent. In polar solvents, a carotenoid radical cation (CAR˙+) and a peroxyl anion (LOO−) are produced from LOO-CAR˙, while in non-polar solvents the SHi reaction proceeds to yield epoxides or cyclic ethers from LOO-CAR˙.
Switching from an antioxidant to a prooxidant is a critical factor when estimating the role of carotenoids in human health and disease prevention.185 Boehm et al.186 demonstrated that lycopene switched from an antioxidant to a prooxidant in human lymphoid cell membranes when the oxygen concentration increased. This switch can also depend on the carotenoid concentration, whereby carotenoids at higher concentrations can act as prooxidants.187 Furthermore, the reaction products of carotenoids with free radicals can also determine whether carotenoids act as antioxidants or prooxidants.188 The carotenoid radical cation (CAR˙+) serves as a strong oxidant of biological components, leading to oxidative damage.188 For example, these radicals participate in the oxidative modification of amino acids such as tyrosine, tryptophan, and cysteine.189 However, as shown in eqn (5) and (6), vitamin E (T-OH) and vitamin C (AsA) may have protective roles against the prooxidant effect of carotenoids by converting CAR˙+ to the original CAR form.190,191
| CAR˙+ + T–OH → CAR + T–O˙ | (5) |
| CAR˙+ + AsA → CAR + AsA˙ | (6) |
Palozza et al.39 noted that carotenoids may act as prooxidants at relatively high concentrations and under chronic oxidative stress. In large-scale β-carotene intervention studies, namely the CARET study and the ATBC trial, it was speculated that the reason why β-carotene supplements were deleterious to smokers was the combined effect of excess β-carotene accumulation and vitamin C deficiency.189
Peroxynitrite (ONOO−), generated by the reaction of nitric oxide (NO) with the superoxide anion radical (O2˙−), is a powerful oxidant in biological systems.192 Carotenoids are suggested to scavenge ONOO− by producing nitro compounds.193–195 Therefore, carotenoids may suppress oxidative damage derived from the combination of NO and O2˙− in biological systems.
4.6 Activation of the Nrf2 signaling pathway and indirect antioxidant activity
Dietary antioxidants may exert their physiological functions during oxidative stress by modulating intracellular signaling pathways that affect the translocation of transcription factors.196 It is known that carotenoids control the expression of genes encoding antioxidant enzymes by enhancing the transcription of the Nrf2 gene.197,198 In this sense, carotenoids act as indirect antioxidants, by enhancing other antioxidant defenses that protect against the oxidative stress that causes chronic diseases.
An elaborate mechanism underlies the activation of the Nrf2 transcription factor, as shown in Fig. 11.199,200
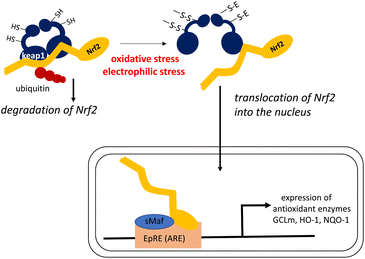 |
| Fig. 11 Oxidative/electrophilic stress-induced activation of the Nrf-2/Keap1 pathway, which induces the expression of genes encoding antioxidant enzymes. EpRE (ARE): electrophile response element (antioxidant response element). | |
In a steady state, Nrf2 is bound to Kelch-like ECH-associated protein-1 (Keap1) resulting in ubiquitinated Nrf2, which is successively decomposed by the ubiquitin–proteasome system. When intracellular oxidative stress is elevated owing to the imbalance between oxidants and antioxidants, a cysteine residue in Keap1 is oxidized to create an intramolecular disulfide bond. This leads to a structural transition, and ultimately the release of Nrf2 from the Nrf2–Keap1 complex without ubiquitination. Free Nrf2 is easily translocated into the nucleus where this transcription factor induces the expression of genes encoding phase II enzymes, antioxidant enzymes, and other enzymes that protect against cellular stress. Nrf2 induces gene expression by forming a heterodimer with the small Maf transcription factor, and then binding to the electrophile response element (EpRE) in the promoter region of its target genes. Electrophilic compounds can also react with the SH group of Keap1 to activate Nrf2 by the same mechanism. Thus, Keap1 regulates the activity of the Nrf2 signaling pathway and acts as a sensor for oxidative and electrophilic stresses. Antioxidant enzymes induced by the Nrf2–Keap1 system include the glutamate–cysteine ligase regulatory subunit (GCLm) for the synthesis of glutathione, as well as heme oxygenase-1 (HO-1) and NAD(P):quinone oxidoreductase-1 (NQO-1).
Experiments using cultured cells have demonstrated that carotenoids induce the expression of genes encoding antioxidant enzymes by activating Nrf2 signaling pathways. For example, lycopene protected mouse photoreceptor cells against light-induced oxidative damage through Nrf2 activation.201 Lutein significantly enhanced Nrf2 translocation to the nucleus and increased the abundance of NQO1, GCLm, and HO-1 in human retinal pigment epithelial cells,202 and protected cerebrovascular endothelial cells against β-amyloid peptide-induced oxidative stress by upregulating Nrf2 expression.203 Zeaxanthin promoted the nuclear translocation of Nrf2 in human retinal pigment epithelium cells exposed to tert-butyl hydroperoxide-induced oxidative stress.204 Similarly, β-cryptoxanthin attenuated H2O2-induced oxidative stress in human renal tubular epithelial cells by promoting Nrf2 nuclear translocation.205
In vivo studies on the indirect antioxidant effects of carotenoids have generally used rodent models. In a cortical impact model mimicking traumatic brain injury, administration of β-carotene to mice reduced ROS levels in the brain, accompanied by nuclear accumulation of Nrf2 and decreased Keap1 expression, indicating that β-carotene alleviated oxidative stress by modulating the Nrf2/Keap1-mediated pathway.206 Rats fed with a high-fat diet supplemented with lycopene and tomato extract showed increased abundance of nuclear Nrf2 and HO-1 proteins and inhibition of nonalcoholic steatohepatitis-promoted hepatocarcinogenesis.207 Supplementation with lycopene was found to alleviate oxidative stress-induced neuroinflammation and cognitive impairment of D-galactose-loaded mice by modulating the Nrf2/nuclear factor kappa B (NF-κB) transcriptional pathway.208 Lycopene also ameliorated renal injury by activating the Nrf2 antioxidant signaling pathway in the aflatoxin B1-induced nephrotoxicity mouse model209 and attenuated oxidative stress and inflammation via the interaction of NF-κB, mitogen-activated protein kinases (MAPKs), and Nrf2 signaling pathways in the chronic prostatitis/chronic pelvic pain syndrome rat model.210 Lycopene alleviated hepatic injury in ischemia reperfusion-stressed mice via Nrf2 activation211 and suppressed phthalate-induced oxidative stress in phthalate-treated mice via mediating the Nrf2 signaling pathway in Leydig cells.212 Lutein showed a protective effect against hepatotoxicity by enhancing Nrf2 signaling in the arsenic-induced oxidative stress mouse model,213 and suppressed oxidative stress and inflammation in ovariectomized rats by Nrf2 activation.214 Zeaxanthin supplementation to rats increased the level of GSH, which contributed to the reduced lipid and protein oxidation in the retina.204 β-Cryptoxanthin ameliorated metabolic risk factors by regulating Nrf2 pathways and NF-κB pathways in rats with insulin resistance induced by a high-fat diet.215
Wang216 explored whether the physiological effect of lycopene results from its metabolites generated by the activity of BCO2, that is, apo-10′-lycopenoids. In fact, it was confirmed that the BCO2-generated lycopene metabolites apo-10′-lycopenoic acid and apo-10′-lycopenal induced the nuclear accumulation of Nrf2, leading to the induction of antioxidant enzymes such as HO-1 in human bronchial cells.217 Bohn et al.218,219 suggested that certain carotenoid metabolites act as suitable electrophiles to react with Nrf2. Linnewiel et al.220 demonstrated that carotenoid oxidation products including apocarotenals, but not hydrophobic carotenoids lacking an electrophilic group, actively mediate Nrf2 during stimulation of the Nrf2 signaling pathway by carotenoids. The proposed reaction of apocarotenals with the SH group of Keap1 is shown in eqn (7).
|  | (7) |
Ketocarotenoids with the 3-oxo β-end group derived from oxidative modification of xanthophylls may also participate in antioxidant defenses. It has been suggested that their electrophilic α,β-unsaturated carbonyl group may contribute to the activation of Nrf2 signaling, leading to the regulation of oxidative stress and inflammation.88 However, the exact mechanism by which dietary carotenoids activate Nrf2 and enhance cellular antioxidant defenses has not been fully demonstrated yet. Many questions remain about the specificity of each enzymatic and/or non-enzymatic oxidation product as the electrophiles inducing nuclear Nrf2 translocation and expression of antioxidant enzymes. Other mechanisms may also contribute to the release of Keap1 from the Nrf2–Keap1 complex by carotenoids. Research to date suggests that the direct promotion of Nrf2 gene expression may be involved in the indirect antioxidant activity of carotenoids. In addition, it should be noted that the metabolism of dietary carotenoids differs between rodents and humans. The rodents used for in vivo experiments are carotene non-accumulators, whereas humans are indiscriminate accumulators.3 Wu et al.221 pointed out the differences in the properties and distribution of BCO2 between mice and humans. These differences may make it difficult to interpret the results of rodent studies in terms of their relevance to humans. Nevertheless, it is expected that these difficulties will be overcome and that the efficacy of dietary carotenoids as indirect antioxidants for human health will be evaluated accurately.
5. Contribution of antioxidative carotenoids to the prevention of human diseases
5.1 Age-related macular degeneration
AMD is one of the major age-related eye diseases in elderly people suffering from severe visual impairment.222 The macula is an oval-shaped pigmented area in the center of the human retina with a diameter of around 5.5 mm. It is responsible for central, high-resolution, and color vision. Oxidative stress and inflammation induced by chronic light exposure are believed to be closely related to the onset of AMD, depending on the wavelength, the irradiation time, and the intensity of the light source.223,224 Blue light with a wavelength of around 450 nm in sunlight causes severe macular damage because the energy of blue light is around 100 times stronger than that of red light. Xanthophylls including lutein, zeaxanthin, and meso-zeaxanthin ((3R,3′S;meso)-zeaxanthin), in which the double bond of the lutein ionone ring is shifted to the neighboring site, are specifically distributed in the human retina.225 Compared with other human cell types, macular cells accumulate these xanthophylls exclusively and to high levels.226,227 Lutein supplementation was known to increase macular pigment optical density in a dose-dependent manner.228 Landrum et al.229 compared macular lutein/zeaxanthin concentrations between control and AMD-affected eyes, and proposed that lower levels of macular pigmentation are a causative factor in AMD development. Overall, dietary lutein and zeaxanthin are thought to help to prevent AMD by acting as macular pigments.227,230 There are two mechanisms by which macular pigments prevent AMD.231,232 One is a shielding effect against phototoxic blue light, as the maximum absorption wavelength of lutein/zeaxanthin is nearly 440 nm in accordance with that of blue light. The other mechanism is an antioxidant effect to scavenge or quench ROS generated under exposure to short-wavelength visible light or UV radiation. Several oxidation products of lutein and zeaxanthin have been detected in the human retina, indicating that lutein and zeaxanthin may act as direct antioxidants to protect the macula against short-wave visible light or UV radiation.233
Several pilot-scale intervention trials have explored the effects of lutein supplementation on vision impairment. Visual function was found to be improved when lutein was administered to AMD patients.234–236 It was suggested that lutein supplementation can improve the visual field and visual acuity in patients with retinitis pigmentosa or Usher syndrome.237,238 Zeaxanthin supplementation provided distinct visual benefits to elderly patients with early atrophic macular degeneration by improving foveal cone-based visual parameters.239 McGill et al.240 demonstrated that rhesus macaques fed with a diet lacking lutein and zeaxanthin for their whole lifetime displayed significantly higher fundus autofluorescence, indicating that a long-term deficiency of dietary lutein and zeaxanthin induces the progression of macular disease in primates. A large-scale intervention study, the Age-Related Eye Disease Study (AREDS), was conducted with 3640 elderly AMD patients for 6.3 years, and demonstrated that daily supplementation with antioxidants (vitamin C 500 mg, vitamin E 400 IU, and β-carotene 15 mg) and zinc (80 mg as zinc oxide) significantly reduced the development of advanced AMD.241 A second study, the Age-Related Eye Disease Study 2 (AREDS2), was conducted in 2006–2012. AREDS2 enrolled 4203 elderly people with a high risk of advanced AMD and explored the effect of adding lutein (10 mg per day) + zeaxanthin (2 mg per day) to the formulation used in the initial AREDS.242 The results implied that lutein + zeaxanthin play a role in reducing the risk of progression to advanced AMD when given without β-carotene, although the addition of lutein + zeaxanthin to the AREDS formulation did not further reduce the risk. An epidemiological 5-year follow-up study of AREDS2 suggested that lutein + zeaxanthin was an appropriate replacement for β-carotene in AREDS2 supplements, as lutein + zeaxanthin supplementation did not increase the risk of lung cancer, unlike β-carotene supplementation.243 Thus, it is conclusive that lutein and zeaxanthin are essential dietary factors for preventing and treating AMD through selective accumulation in the macula and protection against light-induced macular impairment by acting as antioxidants.
5.2 Skin photodamage and photoaging
Human skin is inevitably exposed to solar light during a person's lifetime. During exposure to sunlight, wavelengths in the UV region with higher energy potential often induce skin damage. Although UV-C radiation (200–290 nm) does not reach the earth's surface because it is absorbed by the ozone layer, UV-B (280–320 nm) and UV-A (320–400 nm) radiation in sunlight are capable of irradiating human skin. UV-B attacks the epidermis to induce severe skin damage, e.g., direct DNA strand breakage and generation of ROS.244 UV-A can penetrate the dermis to interact with skin chromophores, which then act as photosensitizers to trigger photosensitized oxidation and generate ROS in skin tissue.245,246 Visible light (400–700 nm) was also reported to contribute to skin damage by producing ROS.247
Sunburn is acute skin damage after overexposure to UV radiation and characteristically yields erythema in the skin tissue.248 Photoaging is chronic skin damage after long-term exposure to sunlight, and is observed as winkles and sagging. Carotenoids are expected to prevent sunlight-induced skin damage because they are powerful antioxidants in skin tissue.249 Stahl et al.250 demonstrated that the carotenoid level in skin was increased after 12 week's daily ingestion of 24 mg β-carotene in 12 women. The highest accumulation of β-carotene was in forehead skin (1.36 ± 0.23 nmol g−1), followed by skin on the palm of the hand (1.03 ± 0.12 nmol g−1), and then skin on the back of the hand (0.54 ± 0.52 nmol g−1). Several intervention studies with supplements or a carotenoid-rich diet documented the efficiency of carotenoids in photoprotection in human skin, as measured by decreased sensitivity to UV-induced erythema.251 Stahl et al.252 demonstrated that the combined intake of mixed carotenoids (25 mg per day) and α-tocopherol (500 IU per day) for 8 weeks suppressed erythema formation after 24 h of blue light irradiation. They also clarified that supplementation with β-carotene (24 mg per day) and supplementation with mixed carotenoids (β-carotene, lutein, lycopene, each 8 mg per day) for 12 weeks reduced the intensity of erythema after 24 h of UV irradiation.253 Daily intake of tomato paste was shown to improve the photoprotection of skin from UV irradiation. In intervention studies where tomato paste (16 mg lycopene per day) was supplied to healthy adults for 10 weeks254 and to healthy women for 12 weeks,255 dorsal erythema formation at 24 h after UV irradiation was lower in those that consumed supplements than in those in the control group (with no tomato paste supplementation). In 2013, Meinke et al.256 conducted a study on 24 healthy volunteers ingesting mixed carotenoid-rich capsules for 8 weeks, and found that increased cutaneous carotenoid levels increased the radical scavenging activity of the skin and provided significant protection against visible and near-infrared light.
Photoaging occurs in the dermis where UV-A radiation may induce ROS-dependent breakdown of extracellular matrix proteins through upregulation of matrix metalloproteinases (MMPs).257 Interestingly, 1O2 was already thought to mediate UV-A radiation-induced synthesis of an interstitial collagenase, MMP-1, in dermis cells.258 Then, Polte and Tyrrel259 reported that peroxidation of membrane lipids is involved in MMP-1 expression in skin fibroblast cells in response to UV-A radiation. The author's group developed a photoaging skin model using hairless mouse with chronic exposure to UV-A radiation. The results of studies using this model suggested that cholesterol hydroperoxides (Ch-OOH) derived from 1O2 oxygenation of membrane lipids mediate the activation of MMP-9, leading to the formation of wrinkles and sagging.260,261 It was also found that dietary β-carotene can prevent both the expression of MMP-9 and the formation of Chol-OOH in the skin. The results of these animal studies support the idea that dietary carotenoids accumulated in the dermis help to prevent photoaging by acting as direct antioxidants against ROS.262
5.3 Antioxidant activity of lycopene in prostate and testis
Epidemiological studies have reported that the plasma lycopene concentration is inversely correlated with the risk of prostate cancer, suggesting that high consumption of lycopene-rich tomatoes is helpful to prevent the incidence of this disease.263,264 However, a prospective study of lycopene and tomato intake in approximately 29
000 men with a follow-up period of 4.2 years did not support the hypothesis that greater lycopene/tomato product consumption protects against prostate cancer.265 A systematic review of intervention trials on lycopene supplementation concluded that there is insufficient evidence to support or refute the use of lycopene to prevent prostate cancer.266 Further studies are required to assess the impact of dietary lycopene consumption upon the incidence of prostate cancer, as a population that may benefit from lycopene supplementation is likely to be predicted.267,268
Lycopene accumulates to higher levels in the testis and seminal plasma than in other organs and fluids.269 The administration of 2 mg lycopene twice a day for 3 months to 30 patients with idiopathic infertility improved their sperm concentration and mobility.270 Daily ingestion of tomato juice containing 30 mg lycopene for 12 weeks by 44 male infertility patients increased their sperm motility.271 Ribeiro et al.272 reported that an imbalance between ROS production and antioxidants leads to impairment of sperm function, and semen ROS levels are strongly related to infertility in men. It is therefore rational to conclude that dietary lycopene acts as an essential antioxidant in the testis to prevent ROS-induced sperm dysfunction.
5.4 Antioxidant effect of carotenoids in cardiovascular disease and other chronic diseases
A systematic review of cohort and case-control studies by Bahonar et al.273 in 2000–2017 showed that higher intake of the six main carotenoids (lycopene, α/β carotene, lutein, zeaxanthin, and astaxanthin) was associated with reduced risks of stroke and other cardiovascular events, with multiple mechanisms other than direct antioxidant activity involved in their beneficial effects. Upregulation of paraoxonase 1 (PON1), an antioxidant enzyme attached to high-density lipoprotein with anti-atherogenic potential, was recently proposed to be the antioxidant mechanism by which β-carotene and lycopene protect LDL from lipid peroxidation.274 A systematic review on diabetic retinopathy therapies concluded that carotenoids can potentially delay the initiation and progression of diabetic retinopathy.275 In liver diseases including non-alcoholic fatty liver disease (NAFLD) and alcoholic liver disease (ALD), carotenoids exert a hepatoprotective effect by acting as antioxidants, provitamin A, and regulators of lipid metabolism in hepatocytes.276,277 Interestingly, a meta-analysis of 12 observational studies showed that total carotenoid intake is inversely associated with depression symptoms, suggesting that dietary intake of carotenoids may help in reducing the risk of depression.278 At present, the antioxidant activity of dietary carotenoids cannot be definitively identified as the key factor in the prevention of these chronic diseases. However, it is reasonable to conclude that dietary carotenoids elevate the body's own antioxidant defense system through Nrf2 signaling pathways, as well as acting as direct scavengers or quenchers of ROS.279
6. Conclusion
Sies280 recently proposed that oxidative stress can be defined as reductive stress (a deviation to the opposite side of the redox balance), oxidative eustress (physiological deviation within the redox control), and oxidative distress (supraphysiological deviation exceeding redox control), depending on the type of deviation from the steady-state cellular redox balance. In humans, the pleiotropic regulation of oxidative stress can help to preserve lifelong health and prevent a variety of chronic diseases. Carotenoids are among the exogeneous antioxidants that contribute to this regulation. Fig. 12 summarizes the function of dietary carotenoids as exogeneous antioxidants working in the human body.
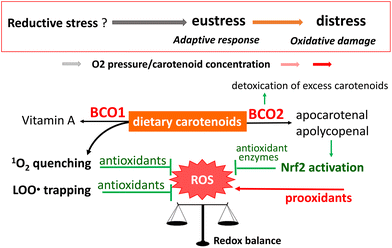 |
| Fig. 12 Proposed scheme for the functions of dietary carotenoids as exogeneous antioxidants in the human body. | |
Although dietary carotenoids of fruit and vegetable origin are partly converted to vitamin A by the enzyme BCO1, a large proportion is translocated to several tissues and biological fluids. In those tissues and fluids, carotenoids exert a direct antioxidant function based on their inherent structures, and/or an indirect antioxidant function after oxidative modification through the actions of BCO2 and other enzymes or non-enzymatic processes. Physical quenching of 1O2 is an essential part of the direct antioxidant function of carotenoids. Research in recent decades has revealed how 1O2 is generated in vivo and how it contributes to the development of biological disorders.146,281,282 Therefore, 1O2 quenching is recognized as a key role of carotenoids in the prevention of oxidative stress-related diseases. However, the relationship between the site and target of 1O2 generation and the location of hydrophobic carotenoids in microenvironments such as phospholipid bilayers of biomembranes is yet to be elucidated, even though the reaction mechanism of carotenoids with 1O2 is well established. In-depth research is still required to assess the efficacy of carotenoids as 1O2 quenchers in vivo.
The antioxidant activity of carotenoids derived from their radical-trapping activity is greatly affected by their concentration and the partial pressure of oxygen at the site of action. Notably, carotenoids are liable to switch from antioxidants to prooxidants in the lipid peroxidation process. Among mammals, humans eccentrically and indiscriminately accumulate carotenoids. As such, humans are predicted to accumulate supplementarily ingested carotenoids in their body to high concentrations, which may induce a switch to prooxidant activity. In addition, each carotenoid is distributed differently in the human body. The mechanisms of, and the reasons for, the high accumulation and different distributions of various carotenoids in the body are still unknown. However, it is known that the dietary lutein and zeaxanthin selectively distributed in the macula contribute to the preservation of vision by protecting the macula from light-induced oxidative stress. The characteristic accumulation of lycopene in the testis may also reflect a pertinent function to protect against oxidative stress, although experimental evidence for this is still insufficient. Skin is an important target for dietary carotenoids to exert their antioxidant activity because human skin is inevitably exposed to light-induced oxidative stress and dietary carotenoids readily accumulate in the skin.
Recently, the induction of antioxidant enzymes via activation of the Nrf2 signaling pathway has attracted much attention as an alternative antioxidant function of dietary carotenoids. This induction relates to cellular redox regulation, which leads to oxidative eustress. The electrophilic property is key for the activation of Nrf2, but carotenoids do not have this property per se. Instead, their oxidative metabolites that are produced by the activity of BCO2 can induce Nrf2 activation, leading to the promotion of antioxidant defenses. At present, it is difficult to present an overview of the roles of BCO2 in carotenoid metabolism because the distribution and activity of BCO2 in human tissues are yet to be clarified. Further research is urgently required to clarify the role of BCO2 in the antioxidant effect of carotenoids.
Intervention studies verified that β-carotene supplementation had harmful effects on smokers and people exposed to asbestos. An acceptable level of carotenoids other than β-carotene should be assessed for clinical applications. At present, it is recommended to obtain a variety of carotenoids by eating a wide range of fruits and vegetables. It can be hypothesized that, during the process of evolution, the human body has used the antioxidant activity of dietary carotenoids instead of eliminating their potential toxicity. It is expected that carotenoid research will progress and will soon be able to explain why humans are indiscriminate carotenoid accumulators.
Conflicts of interest
The author declares no conflict of interest.
Acknowledgements
The author thanks Dr Noriko Bando at Tokushima University, Dr Sayuri Miyamoto at University of Sao Paulo, Dr Akihiko Nagao at Jumonji University, and Dr Ryo Yamauchi at Gifu University for their support in the collection of data on carotenoid function. The author also thanks Jennifer Smith, PhD, from Edanz (https://jp.edanz.com/ac) for editing a draft of this manuscript.
Notes and references
- J. Yabuzaki, Carotenoids data base: structures, chemical fingerprints and distribution among organisms, Database, 2017, bax004 Search PubMed.
- T. Maoka, Carotenoids as natural functional pigments, J. Nat. Med., 2020, 74, 1–16 CrossRef CAS PubMed.
-
T. W. Goodwin, The Biochemistry of the Carotenoids Volume 2. Animals, Chapman and Hall, NY, 2nd edn, 1984 Search PubMed.
- K. A. Slifka, P. E. Bowen, M. Stacewicz-Sapuntzakis and S. D. Crissey, A survey of serum and dietary carotenoids in captive wild animals, J. Nutr., 1999, 129, 380–390 CrossRef CAS PubMed.
- F. Khachik, G. R. Beecher, M. B. Goli, W. R. Lusby and C. E. Daitch, Separation and quantification of carotenoids in human plasma, Methods Enzymol., 1992, 213, 205–219 CAS.
- L. A. Kaplan, J. M. Lau and E. A. Stein, Carotenoid composition, concentrations, and relationships in various human organs, Clin. Physiol. Biochem., 1990, 8, 1–10 CAS.
- J. M. Holden, A. L. Eldridge, G. R. Beecher, I. M. Buzzard, S. Bhagwat, C. S. Davis, L. W. Douglass, S. Gebhardt, D. Haytowitz and S. Schakel, Carotenoid content of U.S. foods: an update of the database, J. Food Compost. Anal., 1999, 12, 169–196 CrossRef CAS.
-
H. Sies, Oxidative stress: Introductory remarks, in Oxidative stress, ed. H. Sies, Academic Press, London, 1985, pp. 1–8 Search PubMed.
-
H. Sies and D. P. Jones, Oxidative stress, in Encyclopedia of Stress, ed. G. Fink, Elsevier, Amsterdam, 2nd edn, 2007, vol. 3, pp. 45–48 Search PubMed.
- G. Britton, Carotenoid research: History and new perspectives for chemistry in biological systems, Biochim. Biophys. Acta, Mol. Cell Biol. Lipids, 2020, 1865, 158699 CrossRef CAS PubMed.
- T. Bohn, M. L. Boner, P. Borel, J. Keijer, J.-F. Landrier, I. Milisav, J. Ribot, P. Riso, B. Winklhofer-Roob, Y. Sharoni, J. Corte-Real, Y. van Helden, M. R. Loizzo, B. Poljsak, M. Porrini, J. Roob, P. Trebse, R. Tundis, A. Wawrzyniak, R. Ruhl and J. Dulinska-Litewka, Mechanistic aspects of carotenoid health benefits-where are we now?, Nutr. Res. Rev., 2021, 34, 276–302 CrossRef CAS PubMed.
- R. K. Saini, P. Prasad, V. Lokesh, X. Shang, J. Shin, Y.-S. Keum and J.-H. Lee, Carotenoids: Dietary sources, Extraction, Encapsulation, Bioavailability and health benefits – A review of recent advancements, Antioxidants, 2022, 11, 795 CrossRef CAS.
- J. Peñuelas and S. Munne-Bosch, Isoprenoids: an evolutionary pool for photoprotection, Trends Plant Sci., 2005, 10, 166–169 CrossRef PubMed.
- C. I. Cazzonelli, Carotenoids in nature: insights from plants and beyond, Funct. Plant Biol., 2011, 38, 833–847 CrossRef CAS PubMed.
- C. S. Foote and R. W. Denny, Chemistry of singlet oxygen. VII. Quenching by beta-carotene, J. Am. Chem. Soc., 1968, 90, 6233–6235 CrossRef CAS.
- P. Di Mascio, S. Kaiser and H. Sies, Lycopene as the most efficient biological carotenoid singlet oxygen quencher, Arch. Biochem. Biophys., 1989, 274, 532–538 CrossRef CAS PubMed.
- A. Bendich and J. A. Olson, Biological actions of carotenoids, FASEB J., 1989, 3, 1927–1932 CrossRef CAS PubMed.
- P. Di Mascio, T. P. Devasagayam, S. Kaiser and H. Sies, Carotenoids, tocopherols and thiols as biological singlet molecular oxygen quenchers, Biochem. Soc. Trans., 1990, 18, 1054–1056 CrossRef CAS PubMed.
- N. I. Krinsky, Carotenoid protection against oxidation, Pure Appl. Chem., 1979, 51, 649–660 CAS.
- B. N. Ames, Dietary carcinogens and anticarcinogens. Oxygen radicals and degenerative diseases, Science, 1983, 221, 1256–1264 CrossRef CAS PubMed.
- C. E. Cross, B. Halliwell, E. T. Borish, W. A. Pryor, B. N. Ames, R. L. Saul, J. M. McCord and D. Harman, Oxygen radicals and human disease, Ann. Intern. Med., 1987, 107, 526–545 CrossRef CAS PubMed.
- R. Peto, R. Doll, J. D. Buckley and M. B. Sporn, Can dietary beta-carotene materially reduce human cancer rates?, Nature, 1981, 290, 201–208 CrossRef CAS PubMed.
- G. W. Burton and K. U. Ingold, beta-Carotene: an unusual type of lipid antioxidant, Science, 1984, 224, 569–573 CrossRef CAS PubMed.
- N. I. Krinsky, The antioxidant and biological properties of the carotenoids, Ann. N. Y. Acad. Sci., 1998, 854, 443–447 CrossRef CAS PubMed.
- A. Bast, G. R. Haenen, R. van den Berg and H. van den Berg, Antioxidant effect of carotenoids, Int. J. Vitam. Nutr. Res., 1998, 68, 399–403 CAS.
- J. Terao, Antioxidant activity of beta-carotene-related carotenoids in solution, Lipids, 1989, 24, 659–661 CrossRef CAS PubMed.
- B. P. Lim, A. Nagao, J. Terao, K. Tanaka, T. Suzuki and K. Takama, Antioxidant activity of xanthophylls on peroxyl radical-mediated phospholipid peroxidation, Biochim. Biophys. Acta, 1992, 1126, 178–184 CAS.
- S. Ohshima, F. Ojima, H. Sakamoto, Y. Ishiguro and J. Terao, Supplementation with carotenoids inhibits singlet oxygen-mediated oxidation of human plasma low-density lipoprotein, J. Agric. Food Chem., 1996, 44, 2306–2309 CrossRef.
- F. Visioli, P. Riso, S. Grande, C. Galli and M. Porrini, Protective activity of tomato products on in vitro markers of lipid oxidation, Eur. J. Nutr., 2003, 42, 201–206 CrossRef CAS PubMed.
- J. L. Witztum and D. Steinberg, Role of oxidized low density lipoprotein in atherogenesis, J. Clin. Invest., 1991, 88, 1785–1792 CrossRef CAS PubMed.
- S. Palmer, Diet, nutrition, and cancer, Prog. Food Nutr. Sci., 1985, 9, 283–341 CAS.
- R. G. Ziegler, A review of epidemiologic evidence that carotenoids reduce the risk of cancer, J. Nutr., 1989, 119, 116–122 CrossRef CAS PubMed.
- W. J. Blot, J. Y. Li, P. R. Taylor, W. Guo, S. Dawsey, G. Q. Wang, C. S. Yang, S. F. Zheng, M. Gail, G. Y. Li, Y. Yu, B. Liu, J. Tangrea, Y. Sun, F. Liu, J. F. Fraumeni, Y. Zhang and B. Li, Nutrition intervention trials in Linxian, China: Supplementation with specific vitamin/mineral combinations, cancer incidence, and disease-specific mortality in the general population, J. Natl. Cancer Inst., 1993, 85, 1483–1491 CrossRef CAS PubMed.
- C. H. Hennekens, J. E. Buring, J. E. Manson, M. Stampfer, B. Rosner, N. R. Cook, C. Belanger, F. LaMotte, J. M. Gaziano, P. M. Ridker, W. Willett and R. Peto, Lack of effect of long-term supplementation with beta-carotene on the incidence of malignant neoplasms and cardiovascular disease, N. Engl. J. Med., 1996, 334, 1145–1149 CrossRef CAS PubMed.
- The Alpha-Tocopherol, Beta-Carotene Cancer Prevention Study Group, The effect of vitamin E and beta carotene on the incidence of lung cancer and other cancers in male smokers, N. Engl. J. Med., 1994, 33, 1029–1035 Search PubMed.
- G. S. Omenn, G. E. Goodman, M. D. Thornquist, J. Balmes, M. R. Cullen, A. Glass, J. P. Keogh, F. L. Meyskens Jr., B. Valanis, J. H. Williams Jr., S. Barnhart, M. G. Cherniack, C. A. Brodkin and S. Hammar, Risk factors for lung cancer and for intervention effects in CARET, the beta-carotene and retinol efficacy trial, J. Natl. Cancer Inst., 1996, 88, 1550–1591 CrossRef CAS PubMed.
- S. T. Mayne, B. Cartmel, F. Silva, C. S. Kim, B. G. Fallon, K. Briskin, T. Zheng, M. Baum, G. Shor-Posner and W. J. Goodwin Jr., Effect of supplemental beta-carotene on plasma concentrations of carotenoids, retinols, and alpha-tocopherol in humans, Am. J. Clin. Nutr., 1998, 68, 642–647 CrossRef CAS PubMed.
- D. Albanes, J. Virtamo, P. R. Taylor, M. Rautalahti, P. Pietinen and O. P. Heinonen, Effects of supplemental beta-carotene, cigarette smoking, and alcohol consumption on serum carotenoids in Alpha-Tocopherol, Beta-Carotene Cancer Prevention Study, Am. J. Clin. Nutr., 1997, 66, 366–372 CrossRef CAS PubMed.
- P. Palozza, Prooxidant actions of carotenoids in biological systems, Nutr. Rev., 1998, 56, 257–265 CrossRef CAS PubMed.
- L. Patrick, Beta-carotene: the controversy continues, Altern. Med. Rev., 2000, 5, 530–545 CAS.
- J. Kordiak, F. Bielec, S. Jablonski and D. Pastuszak-Lewandoska, Role of beta-carotene in lung cancer primary chemoprevention; a systematic review with meta-analysis and meta-regression, Nutrients, 2022, 14, 1361 CrossRef CAS PubMed.
- N. Druesne-Pecollo, P. Latino-Martel, T. Norat, E. Barrandon, S. Bertrais, P. Galan and S. Hercberg, Beta-carotene supplementation and cancer risk: a systematic review and metaanalysis of randomized controlled trials, Int. J. Cancer, 2010, 127, 172–184 CrossRef CAS PubMed.
-
L. Packer and C. Colman, The antioxidant miracle: Your complete plan for total health and healing, John Wiley and Sons, Inc., New York, 1999 Search PubMed.
- Heart Protection Study Collaborative Group, MRC/BHF heart protection study of antioxidant vitamin supplementation in 20,536 high-risk individuals: a randomized placebo-controlled trial, Lancet, 2002, 360, 23–33 CrossRef PubMed.
- G. Bjelakovic, D. Nikolova, L. L. Gluud, R. G. Simonetti and C. Gluud, Mortality in randomized trials of antioxidant supplements for primary and secondary prevention: systematic review and meta-analysis, J. Am. Med. Assoc., 2007, 297, 842–857 CrossRef CAS PubMed.
- G. Bjelakovic, D. Nikolova, L. L. Gluud, R. G. Simonetti and C. Gluud, Antioxidant supplements for prevention of mortality in healthy participants with various disease (Review), Cochrane Database Syst. Rev., 2012, 3, CD007176 Search PubMed.
- G. Bjelakovic, D. Nikolova and C. Gluud, Antioxidant supplements and mortality, Curr. Opin. Clin. Nutr. Metab. Care, 2014, 17, 40–44 CAS.
-
U.S. Department of Health and Human Services and National Institute of Health, Antioxidants: In depth https://nccih.nih.gov/health/antioxidants/indroduction.htm, Jan. 21, 2023.
- M. Ezzati and E. Riboli, Behavioral and dietary risk factors for noncommunicable diseases, N. Engl. J. Med., 2013, 369, 954–964 CrossRef CAS PubMed.
- M. Ezzati and E. Riboli, Can noncommunicable diseases be prevented? Lessons from studies of populations and individuals, Science, 2012, 337, 1482–1487 CrossRef PubMed.
- Y. Sahashi, A. Goto, R. Takachi, J. Ishihara, K. Kito, R. Kanehara, T. Yamaji, M. Iwasaki, M. Inoue, S. Tsugane and N. Sawada, Inverse association between fruit and vegetable intake and all-cause mortality: Japan public health center-based prospective study, J. Nutr., 2022, 152, 2245–2254 CrossRef PubMed.
- N. J. Barber and J. Barber, Lycopene and prostate cancer, Prostate Cancer Prostatic Dis., 2002, 5, 6–12 CrossRef CAS PubMed.
- D. M. Snodderly, Evidence for protection against age-related macular degeneration by carotenoids and antioxidant vitamins, Am. J. Clin. Nutr., 1995, 62, 1448S–1461S CrossRef CAS PubMed.
- H. K. Biesalski and U. C. Obermueller-Jevic, UV-light, beta-carotene and human skin-beneficial and potentially harmful effects, Arch. Biochem. Biophys., 2001, 389, 1–6 CrossRef CAS PubMed.
- J. A. Olson and O. Hayaishi, The enzymatic cleavage of β-carotene into vitamin A by soluble enzymes of rat liver and intestine, Proc. Natl. Acad. Sci. U. S. A., 1965, 54, 1364–1369 CrossRef CAS PubMed.
- D. S. Goodman and H. S. Huang, Biosynthesis of vitamin A with rat intestinal
enzymes, Science, 1965, 149, 879–880 CrossRef CAS PubMed.
- M. R. Lakshman, Alpha and omega of carotenoid cleavage, J. Nutr., 2004, 134, 241S–245S CrossRef CAS PubMed.
- C. Kiefer, S. Hessel, J. M. Lampert, K. Vogt, M. O. Lederer, D. E. Breithaupt and J. von Lintig, Identification and characterization of a mammalian enzyme catalyzing the asymmetric oxidative cleavage of provitamin A, J. Biol. Chem., 2001, 276, 14110–14116 CrossRef CAS PubMed.
- K.-Q. Hu, C. Liu, H. Ernst, N. I. Krinsky, R. M. Russell and X.-D. Wang, The biochemical characterization of ferret carotene-9′,10′-monooygenase catalyzing cleavage of carotenoids in vitro and in vivo, J. Biol. Chem., 2006, 281, 19327–19338 CrossRef CAS PubMed.
- J. R. Mein, G. G. Dolnikoski, H. Ernst, R. M. Russell and X.-D. Wang, Enzymatic formation of apo-carotenoids from the xanthophyll carotenoids lutein, zeaxanthin and β-cryptoxanthin by ferret carotene-9′,10′-monooxygenase, Arch. Biochem. Biophys., 2011, 506, 109–121 CrossRef CAS PubMed.
- F. Khachik, C. J. Spangler, J. C. Smith Jr., L. M. Canfield, A. Steck and H. Pfander, Identification, quantification, and relative concentrations of carotenoids and their metabolites in human milk and serum, Anal. Chem., 1997, 69, 1873–1881 CrossRef CAS PubMed.
- A. Ben-Dor, M. Steiner, L. Gheber, M. Danilenko, N. Dubi, K. Linnewiel, A. Zick, Y. Sharoni and J. Levy, Carotenoids activate the antioxidant response element transcription system, Mol. Cancer Ther., 2005, 4, 177–186 CrossRef CAS PubMed.
- B. L. Lindshild, K. Canene-Adams and J. W. Erdman Jr., Lycopenoids: Are lycopene metabolites bioactive?, Arch. Biochem. Biophys., 2007, 458, 136–140 CrossRef PubMed.
- K. Linnewiel, H. Ernst, C. Caris-Veyrat, A. Ben-Dor, A. Kampf, H. Salman, M. Danilenko, J. Levy and Y. Sharoni, Structure activity relationship of carotenoid derivatives in activation of the electrophile/antioxidant response element transcription system, Free Radicals Biol. Med., 2009, 47, 659–667 CrossRef CAS PubMed.
- L. Yonekura and A. Nagao, Intestinal absorption of dietary carotenoids, Mol. Nutr. Food Res., 2007, 51, 107–115 CrossRef CAS PubMed.
- J. von Lintig, J. Moon, J. Lee and S. Ramkumar, Carotenoid metabolism at the intestinal barrier, Biochim. Biophys. Acta, Mol. Cell Biol. Lipids, 2020, 1865, 158580 CrossRef CAS PubMed.
- L. R. B. Mariutti and A. Z. Mercadante, Carotenoid esters analysis and occurrence: What do we know so far?, Arch. Biochem. Biophys., 2018, 648, 36–43 CrossRef CAS PubMed.
- D. E. Breithaupt, A. Barmedi and U. Wirt, Carotenol fatty acid esters: easy substrates for digestive enzymes?, Comp. Biochem. Physiol., Part B: Biochem. Mol. Biol., 2002, 132, 721–728 CrossRef PubMed.
- C. Chitchumroonchokchai and M. L. Failla, Hydrolysis of zeaxanthin esters by carboxyl ester lipase during digestion facilitates micellization and uptake of the xanthophyll by Caco-2 human intestinal cells, J. Nutr., 2006, 136, 588–594 CrossRef CAS PubMed.
- M. J. Brown, M. G. Ferruzzi, M. L. Nguyen, D. A. Cooper, A. L. Eldridge, S. J. Schwartz and W. S. White, Carotenoid bioavailability is higher from salads ingested with full-fat than with fat-reduced salad dressings as measured with electrochemical detection, Am. J. Clin. Nutr., 2004, 80, 396–403 CrossRef CAS PubMed.
- A. Van Bennekum, M. Werder, S. T. Thuahnai, C.-H. Han, P. Duong, D. L. Williams, P. Wettstein, G. Schulthess, M. C. Phillips and H. Hauser, Class B scavenger receptor-mediated intestinal absorption of dietary beta-carotene and cholesterol, Biochemistry, 2005, 44, 4517–4525 CrossRef CAS PubMed.
- M. Moussa, J.-F. Landrier, E. Reboul, O. Ghiringhelli, C. Comera, X. Colllet, K. Frohlich, V. Bohm and P. Borel, Lycopene absorption in human intestinal cells and in mice involves scavenger receptor class B type I but not Niemann-Pick C1-like 1, J. Nutr., 2008, 138, 1432–1436 CrossRef CAS PubMed.
- P. Borel, G. Lietz, A. Goncalves, F. S. de Edelenyi, S. Lecompe, P. Curtis, L. Goumidi, M. J. Caslake, E. A. Miles, C. Packard, P. C. Calder, J. C. Mathers, A. M. Minihane, F. Tourniaire, E. Kesse-Guyot, P. Galan, S. Hercberg, C. Breidenassel, M. G. Gross, M. Moussa, A. Meirhaeghe and E. Reboul, CD36 and SR-B1 are involved in cellular uptake of provitamin A carotenoids by Caco-2 and HEK cells, and some of their genetic variants are associated with plasma concentrations of these micronutrients in humans, J. Nutr., 2013, 143, 448–456 CrossRef CAS PubMed.
- A. Lindqvist and S. Andersson, Biochemical properties of purified recombinant human β-carotene 15,15′-monooxygenase, J. Biol. Chem., 2002, 277, 23942–23948 CrossRef CAS PubMed.
- G. Lobo, S. Hessel, A. Eichinger, N. Noy, A. R. Moise, A. Wyss, K. Palczewski and J. von Lintig, ISX is a retinoic acid-sensitive gatekeeper that controls intestinal beta, beta-carotene absorption and vitamin A production, FASEB J., 2010, 24, 1656–1666 CrossRef CAS PubMed.
- G. Palczewski, J. Amengual, C. L. Hoppel and J. von Lintig, Evidence for compartmentalization of mammalian carotenoid metabolism, FASEB J., 2014, 28, 4457–4469 CrossRef CAS PubMed.
- J. Amengual, G. P. Lobo, M. Golczak, H. N. M. Li, T. Klimova, C. L. Hoppel, A. Wyss, K. Palczewski and J. von Lintig, A mitochondrial enzyme degrades carotenoids and protect against oxidative stress, FASEB J., 2011, 25, 948–959 CrossRef CAS PubMed.
- G. P. Lobo, A. Isken, S. Hoff, D. Babino and J. von Lintig, BCDO2 acts as a carotenoid scavenger and gatekeeper for the mitochondrial apoptotic pathway, Development, 2012, 139, 2966–2977 CrossRef CAS PubMed.
- L. Wu, X. Guo, S. D. Hartson, M. A. Davis, H. He, D. M. Medeiros, W. Wang, S. L. Clarke, E. A. Lucas, B. J. Smith, J. von Lintig and D. Lin, Lack of β,β-carotene-9′,10′-oxygenase 2 leads to hepatic mitochondrial dysfunction and cellular oxidative stress in mice, Mol. Nutr. Food Res., 2017, 61, 1600576 CrossRef PubMed.
- X. Guo, L. Wu, Y. Lyu, W. Chowanadisai, S. L. Clarke, E. A. Lucas, B. J. Smith, H. He, W. Wang, D. M. Medeiros and D. Lin, Ablation of β,β-carotene-9′,10′-oxygenase 2 remodels the hypothalamic metabolome leading to metabolic disorders in mice, J. Nutr. Biochem., 2017, 46, 74–82 CrossRef CAS PubMed.
- L. Wu, X. Guo, W. Wang, D. M. Medeiros, S. L. Clarke, E. A. Lucas, B. J. Smith and D. Lin, Molecular aspects of β,β-carotene-9′,10′-oxygenase 2 in carotenoid metabolism and diseases, Exp. Biol. Med., 2016, 241, 1879–1887 CrossRef CAS PubMed.
- D. Babino, G. Palczewski, M. A. K. Widjaja-Adhi, P. D. Kiser, M. Golczak and J. von Lintig, Characterization of the role of β-carotene 9,10-dioxygenase in macular pigment metabolism, J. Biol. Chem., 2015, 290, 24844–24857 CrossRef CAS PubMed.
- B. Li, P. P. Vachali, A. Gorusupudi, Z. Shen, H. Sharifzadeh, B. M. Besch, K. Nelson, M. M. Horvath, J. M. Frederick, W. Baehr and P. S. Bernstein, Inactivity of human β,β-carotene-9′,10′-dioxygenase (BCO2) underlies retinal accumulation of the human macular carotenoid pigment, Proc. Natl. Acad. Sci. U. S. A., 2014, 111, 10173–10178 CrossRef CAS PubMed.
- F. Lian and X.-D. Wang, Enzymatic metabolites of lycopene induce Nrf2-mediated expression of phase II detoxifying/antioxidant enzymes in human bronchial epithelial cells, Int. J. Cancer, 2008, 123, 1262–1268 CrossRef CAS PubMed.
- A. Nagao, T. Maoka, H. Ono, E. Kotake-Nara, M. Kobayashi and M. Tomita, A 3-hydroxy β-end group in xanthophylls is preferentially oxidized to a 3-oxo-ε-end group in mammals, J. Lipid Res., 2015, 56, 449–462 CrossRef CAS PubMed.
- V. Böhm, G. Lietz, B. Olmedilla-Alonso, D. Phelan, E. Reboul, D. Banati, P. Borel, J. Corte-Real, A. R. de Lera, C. Desmarchelier, J. Dulinska-Litewka, J.-F. Landrier, I. Milisav, J. Nolan, M. Porrini, P. Riso, J. M. Roob, E. Valanou, A. Wawrzyniak, B. M. Winklhofer-Roob, R. Ruhl and T. Bohn, From carotenoid intake to carotenoid blood and tissue concentrations-implications for dietary intake recommendations, Nutr. Rev., 2021, 79, 544–573 CrossRef PubMed.
- P. Galan, F. E. Viteri, S. Bertrais, S. Czernichow, H. Faure, J. Arnaud, D. Ruffieux, S. Chenal, N. Arnault, A. Favier, A.-M. Roussel and S. Hercberg, Serum concentrations of beta-carotene, vitamins C and E, zinc and selenium are influenced by sex, age, diet, smoking status, alcohol consumption and corpulence in a general French adult population, Eur. J. Clin. Nutr., 2005, 59, 1181–1190 CrossRef CAS PubMed.
- W. Stahl, W. Schwarz, A. R. Sundquist and H. Sies, cis-trans Isomers of lycopene and beta-carotene in human serum and tissues, Arch. Biochem. Biophys., 1992, 294, 173–177 CrossRef CAS PubMed.
- S. Beatty, J. Nolan, H. Kavanagh and O. O'Donovan, Macular pigment optical density and its relationship with serum and dietary levels of lutein and zeaxanthin, Arch. Biochem. Biophys., 2004, 430, 70–76 CrossRef CAS PubMed.
- B. J. Burri, T. R. Neidlinger and A. J. Clifford, Serum carotenoid depletion follows first-order kinetics in healthy adult women fed naturally low carotenoid diets, J. Nutr., 2001, 131, 2096–2100 CrossRef CAS PubMed.
- C. L. Rock, M. E. Swendseid, R. A. Jacob and R. W. McKee, Plasma carotenoid levels in human subjects fed a low carotenoid diet, J. Nutr., 1992, 122, 96–100 CrossRef CAS PubMed.
- M. C. DeRosa and R. J. Crutchley, Photosensitized singlet oxygen and its applications, Coord. Chem. Rev., 2002, 233–234, 351–371 CrossRef CAS.
- M. Orfanopoulos and C. S. Foote, The ene reaction of singlet oxygen with olefins, Free Radical Res. Commun., 1987, 2, 321–326 CrossRef CAS PubMed.
- C. S. Foote, Mechanism of addition of singlet oxygen to olefins and other substrates, Pure Appl. Chem., 1971, 27, 635–646 CrossRef CAS.
- K. Briviba, L. O. Klotz and H. Sies, Toxic and signaling effects of photochemically or chemically generated singlet oxygen in biological systems, Biol. Chem., 1997, 378, 1259–1265 CAS.
- C. S. Foote, Photosensitized oxygenation and the role of singlet oxygen, Acc. Chem. Res., 1968, 1, 104–110 CrossRef CAS.
- C. S. Foote, Mechanism of photosensitized oxidation. There are several different types of photosensitized oxidation which may be important in biological systems, Science, 1968, 162, 963–970 CrossRef CAS PubMed.
- C. S. Foote, Definition of type I and type II photosensitized oxidation, Photochem. Photobiol., 1991, 54, 659 CrossRef CAS PubMed.
- D. R. Cardoso, S. H. Libardi and L. H. Skibsted, Riboflavin as a photosensitizer. Effects on human health and food quality, Food Funct., 2012, 3, 487–502 RSC.
- L. I. Grossweiner, Photochemistry of proteins: a review, Curr. Eye Res., 1984, 3, 137–144 CrossRef CAS PubMed.
- P. Walrant and R. Santus, N-Formyl-kynurenine, a tryptophan photooxidation product, as a photodynamic sensitizer, Photochem. Photobiol., 1974, 19, 411–417 CrossRef CAS PubMed.
- G. R. Seely, Photosensitization reactions of chlorophyll, Methods Enzymol., 1972, 24, 238–246 CAS.
- D. Kessel and K. Smith, Photosensitization with derivatives of chlorophyll, Photochem. Photobiol., 1989, 49, 157–160 CrossRef CAS PubMed.
- L. E. Xodo, V. Rapozzi, M. Zacchigna, S. Drioli and S. Zorzet, The chlorophyll catabolite pheophorbide a as a photosensitizer for the photodynamic therapy, Curr. Med. Chem., 2012, 19, 799–807 CrossRef CAS PubMed.
- D. A. Grekin and J. H. Epstein, Psoralenes, UVA (PUVA) and photocarcinogenesis, Photochem. Photobiol., 1981, 33, 957–960 CrossRef CAS PubMed.
- M. A. Pathak, Molecular aspects of drug photosensitivity with special emphasis on psoralene photosensitization reaction, J. Natl. Cancer Inst., 1982, 69, 163–170 CrossRef CAS PubMed.
- M. A. Pathak and P. C. Joshi, Production of active oxygen species (1O2 and O2−˙) by psoralens and ultraviolet radiation (320–400 nm), Biochim. Biophys. Acta, 1984, 798, 115–126 CrossRef CAS PubMed.
- S. Wu, J. Han, D. Feskanich, E. Cho, M. J. Stampfer, W. C. Willett and A. A. Qureshi, Citrus consumption and risk of cutaneous malignant melanoma, J. Clin. Oncol., 2015, 33, 2500–2508 CrossRef PubMed.
- D. Kerekes, A. Csorba, B. Gosztola, E. Nemeth-Zambori, T. Kiss and D. Csupor, Furocoumarin content of fennel-below the safety threshold, Molecules, 2019, 24, 2844 CrossRef CAS PubMed.
- N. Hayashi, K. Togawa, M. Yanagisawa, J. Hosogi, D. Mimura and Y. Yamamoto, Effect of sunlight exposure and aging on skin surface lipids and urate, Exp. Dermatol., 2003, 12S, 13–17 CrossRef PubMed.
- S. E. Mudiyanselage, M. Hamburger, P. Elsner and J. J. Thiele, Ultraviolet a induces generation of squalene monohydroperoxide isomers in human sebum and skin surface lipids in vitro and in vivo, J. Invest. Dermatol., 2003, 120, 915–922 CrossRef CAS PubMed.
- Y. Kohno, Y. Egawa, S. Itoh, T. Nagaoka, M. Takahashi and K. Mukai, Kinetic study of quenching reaction of singlet oxygen and scavenging reaction of free radical by squalene in n-butanol, Biochim. Biophys. Acta, 1995, 1256, 52–56 CrossRef PubMed.
- A. Natsch, R. Emter, R. P. Badertscher, G. Brunner, T. Granier, S. Kern and G. Ellis, Oxidative tryptophan modification by terpene- and squalene-hydroperoxides and a possible link to cross-reactions in diagnostic tests, Chem. Res. Toxicol., 2015, 28, 1205–1208 Search PubMed.
- G. T. Wondrak, M. J. Roberts, D. Cervantes-Laurean, M. K. Jacobson and E. L. Jacobson, Proteins of the extracellular matrix are sensitizers of photo-oxidative stress in human skin cells, J. Invest. Dermatol., 2003, 121, 578–586 CrossRef CAS PubMed.
- G. T. Wondrak, M. J. Roberts, M. K. Jacobson and E. L. Jacobson, 3-Hydroxyprydine chromophores are endogenous sensitizers of photooxidative stress in human skin cells, J. Biol. Chem., 2004, 279, 3009–3020 CrossRef PubMed.
- K. Arakane, A. Ryu, C. Hayashi, T. Matsunaga, K. Shinmoto, S. Mashiko, T. Nagano and M. Hirobe, Singlet oxygen (1Δg) generation from coproporphyrin in propionibacterium acnes on irradiation, Biochem. Biophys. Res. Commun., 1996, 223, 578–582 CrossRef CAS PubMed.
- J. R. Kanofsky, Singlet oxygen production by biological systems, Chem.-Biol. Interact., 1989, 70, 1–28 CrossRef CAS PubMed.
- S. Miyamoto, G. R. Martinez, M. H. G. Medeiros and P. Di Mascio, Singlet molecular oxygen generated by biological hydroperoxides, J. Photochem. Photobiol., B, 2014, 139, 24–33 CrossRef CAS PubMed.
- G. A. Russell, Deuterium-isotope effects in the autoxidation of alkyl hydrocarbons. Mechanism of the interaction of peroxyl radicals, J. Am. Chem. Soc., 1957, 79, 3871–3877 CrossRef CAS.
- S. Miyamoto, G. R. Martinez, M. H. G. Medeiros and P. Di Mascio, Singlet molecular oxygen generated from lipid hydroperoxides by the Russell mechanism: Studies using 18O-labeled linoleic acid hydroperoxide and monomol light emission measurements, J. Am. Chem. Soc., 2003, 125, 6172–6179 CrossRef CAS PubMed.
- M. Uemi, G. E. Ronsein, F. M. Prado, F. D. Motta, S. Miyamoto, M. H. G. Medeiros and P. Di Mascio, Cholesterol hydroperoxides generate singlet molecular oxygen [O2(1Δg)]: near-IR emission 18O-labeled hydroperoxides, and mass spectrometry, Chem. Res. Toxicol., 2011, 24, 887–895 Search PubMed.
- S. Miyamoto, G. R. Martinez, A. P. B. Martin, M. H. G. Medeiros and P. Di Mascio, Direct evidence of singlet molecular oxygen O2 (1Δg) production in the reaction of linoleic acid hydroperoxide with peroxynitrite, J. Am. Chem. Soc., 2003, 125, 4510–4517 CrossRef CAS PubMed.
- S. Miyamoto, I. L. Nantes, P. A. Faria, D. Cunha, G. E. Ronsein, M. H. G. Medeiros and P. Di Mascio, Cytochrome c-promoted cardiolipin oxidation generates singlet molecular oxygen, Photochem. Photobiol. Sci., 2012, 11, 1536–1546 CrossRef CAS PubMed.
- C. M. Mano, F. M. Prado, J. Massari, G. E. Ronsein, G. R. Martinez, S. Miyamoto, J. Cadel, H. Sies, M. H. G. Medeiros, E. J. H. Bechara and P. Di Mascio, Excited singlet molecular O2 (1Δg) is generated enzymatically from excited carbonyls in the dark, Sci. Rep., 2014, 4, 5398 CrossRef PubMed.
- M. J. Steinbeck, A. U. Khan and M. J. Karnovsky, Intracellular singlet oxygen generation by phagocytosing neutrophils in response to particles coated with a chemical trap, J. Biol. Chem., 1992, 267, 13425–13433 CrossRef CAS PubMed.
- S. Miyamoto, G. R. Martinez, D. Rettori, O. Augusto, M. H. G. Medeiros and P. Di Mascio, Linoleic acid hydroperoxide reacts with hypochlorous acid, generating peroxyl radical intermediates and singlet molecular oxygen, Proc. Natl. Acad. Sci. U. S. A., 2006, 103, 293–298 CrossRef CAS PubMed.
- R. O. Nascimento, F. M. Prado, M. H. G. de Medeiros, G. E. Ronsein and P. Di Mascio, Singlet molecular oxygen generation in the reaction of biological haloamines of amino acids and polyamines with hydrogen peroxide, Photochem. Photobiol., 2023, 99, 661–671 CrossRef CAS PubMed.
- J. Terao and S. Matsushita, Products formed by photosensitized oxidation of unsaturated fatty acid esters, J. Am. Oil Chem. Soc., 1977, 54, 234–238 CrossRef CAS.
- N. Frankel, Chemistry of free radical and singlet oxidation of lipids, Prog. Lipid Res., 1984, 23, 97–221 CrossRef PubMed.
- E. Niki, Y. Yoshida, Y. Saito and N. Noguchi, Lipid peroxidation: mechanisms, inhibition, and biological effects, Biochem. Biophys. Res. Commun., 2005, 338, 668–676 CrossRef CAS PubMed.
- A. Umeno, M. Shichiri, N. Ishida, Y. Hashimoto, K. Abe, M. Kataoka, K. Yoshino, Y. Hagihara, N. Aki, M. Funaki, Y. Asada and Y. Yoshida, Singlet oxygen induced products of linoleates, 10- and 12-(Z,E)-hydroxyoctadecadienoic acids (HODE), can be potential biomarkers for early detection of type 2 diabetes, PLoS One, 2013, 8, e63542 CrossRef CAS PubMed.
- A. Umeno, K. Yoshino, Y. Hashimoto, M. Shichiri, M. Kataoka and Y. Yoshida, Multi-biomarkers for early detection of type 2 diabetes, including 10- and 12-(Z,E)-hydroxyoctadecadienoic acids, insulin, leptin, and adiponectin, PLoS One, 2015, 10, e0130971 CrossRef PubMed.
- C. Vever-Bizet, M. Dellinger, D. Brault, M. Rougee and R. V. Bensasson, Singlet molecular oxygen quenching by saturated and unsaturated fatty-acids and by cholesterol, Photochem. Photobiol., 1989, 50, 321–325 CrossRef CAS PubMed.
- A. A. Frimer, The reaction of singlet oxygen with olefins: the question of mechanism, Chem. Rev., 1979, 79, 359–387 CrossRef CAS.
- J. Brinkhorst, S. J. Nara and D. A. Pratt, Hock cleavage of cholesterol 5alpha-hydroperoxide:
An ozone-free pathway to the cholesterol ozonolysis products identified in arterial plaque and brain tissue, J. Am. Chem. Soc., 2008, 130, 12224–12225 CrossRef CAS PubMed.
- C. Takeuchi, R. Galve, J. Nieva, D. P. Witter, A. D. Wentworth, R. P. Troseth, R. A. Lerner and P. Wentworth Jr., Proatherogenic effects of the cholesterol ozonolysis products, atheronal-A and atheronal-B, Biochemistry, 2006, 45, 7162–7170 CrossRef CAS PubMed.
- S. Miyamoto, R. S. Lima, A. Inague and L. G. Viviani, Electrophilic oxysterols: generation, measurement and protein modification, Free Radic. Res., 2021, 55, 416–440 CrossRef CAS PubMed.
- S. J. Dixon, K. M. Lemberg, M. R. Lamprecht, R. Skouta, E. M. Zaitsev, C. E. Gleason, D. N. Patel, A. J. Bauer, A. M. Cantley, W. S. Yang, B. M. Morrison III and B. R. Stockwell, Ferroptosis: An iron-dependent form of nonapoptotic cell death, Cell, 2012, 149, 1060–1072 CrossRef CAS PubMed.
- F. Ursini, M. Maiorino and C. Gregolin, The selenoenzyme phospholipid hydroperoxide glutathione peroxidase, Biochim. Biophys. Acta, 1985, 839, 62–70 CrossRef CAS PubMed.
- T. P. James, M. Maiorino, F. Ursini and A. W. Girotti, Protective action of phospholipid hydroperoxide glutathione peroxidase against membrane-damaging lipid peroxidation. In situ reduction of phospholipid and cholesterol hydroperoxides, J. Biol. Chem., 1990, 265, 454–461 CrossRef.
- P. G. Geiger, W. Korytowski and A. W. Girotti, Photodynamically generated 3-beta-hydroxy-5 alpha-cholestet-6-ene-5-hydroperoxide: toxic reactivity in membranes and susceptibility to enzymatic detoxification, Photochem. Photobiol., 1995, 62, 580–587 CrossRef CAS PubMed.
- N. Kajarabille and G. O. Lantunde-Dada, Programmed cell-death by ferroptosis: Antioxidants as mitigators, Int. J. Mol. Sci., 2019, 20, 4968 CrossRef CAS PubMed.
- T. Homma, S. Kobayashi and J. Fujii, Induction of ferroptosis by singlet oxygen generated from naphthalene endoperoxide, Biochem. Biophys. Res. Commnun., 2019, 518, 519–525 CrossRef CAS PubMed.
- J. Cadet, T. Douki and J.-L. Ravanat, Oxidatively generated damage to the guanine moiety of DNA: mechanistic aspects and formation in cells, Acc. Chem. Res., 2008, 41, 1075–1083 CrossRef CAS PubMed.
- J. Cadet, T. Douki and J.-L. Ravanat, Oxidatively generated damage to cellular DNA by UVB and UVA radiation, Photochem. Photobiol., 2015, 91, 140–155 CrossRef CAS PubMed.
- P. Di Mascio, G. R. Martinez, S. Miyamoto, G. E. Ronsein, M. H. G. Medeiros and J. Cadet, Singlet molecular oxygen reactions with nucleic acids, lipids and proteins, Chem. Rev., 2019, 119, 2043–2086 CrossRef CAS PubMed.
- F. Prat, C.-C. Hou and C. S. Foote, Determination of the quenching rate constants of singlet oxygen by derivatized nucleosides in nonaqueous solution, J. Am. Chem. Soc., 1997, 119, 5051–5052 CrossRef CAS.
- J. E. Schenider, S. Price, L. Madit, J. M. C. Gutteridge and R. A. Floyd, Methylene blue plus light mediates 8-hydroxy 2′-deoxyguanosine formation in DNA preferentially over strand breakage, Nucleic Acid Res., 1990, 18, 631–635 CrossRef PubMed.
- J.-L. Ravanat, G. R. Martinez, M. H. G. Medeiros, P. Di Mascio and J. Cadet, Mechanistic aspects of the oxidation of DNA constituents mediated by singlet molecular oxygen, Arch. Biochem. Biophys., 2004, 423, 23–30 CrossRef CAS PubMed.
- B. Sjoberg, S. Foley, A. Staicu, A. Pascu, M. Pascu and M. Enescu, Protein reactivity with singlet oxygen: influence of the solvent exposure of the reactive amino acid residues, J. Photochem. Photobiol., B, 2016, 159, 106–110 CrossRef CAS PubMed.
- M. Rougee, R. V. Bensasson, E. L. Land and R. Pariente, Deactivation of singlet molecular oxygen by thiols and related compounds, possible protectors against skin photosensitivity, Photochem. Photobiol., 1988, 47, 485–489 CrossRef CAS PubMed.
- G. R. Buettner and R. D. Hall, Superoxide, hydrogen peroxide
and singlet oxygen in hematoporphyrin derivative-cysteine, -NADH and -light systems, Biochim. Biophys. Acta, 1987, 923, 501–507 CrossRef CAS PubMed.
- P. K. Sysak, C. S. Foote and T.-Y. Ching, Chemistry of singlet oxygen-XXV. Photooxygenation of methionine, Photochem. Photobiol., 1977, 26, 19–27 CrossRef CAS.
- P. Kang and C. S. Foote, Synthesis of a 13C, 15N labeled imidazole and characterization of the 2,5-endoperoxide and its decomposition, Tetrahedron Lett., 2000, 41, 9623–9626 CrossRef CAS.
- V. V. Agon, W. A. Bubb, A. Wright, C. L. Hawkins and M. J. Davies, Sensitizer-mediated photooxidation of histidine residues: evidence for the formation of reactive side-chain peroxides, Free Radicals Biol. Med., 2006, 40, 698–710 CrossRef CAS PubMed.
- H. Wasserman, M. S. Wolf, K. Stiller, I. Saito and J. E. Pickett, The dye-sensitized photooxidation of imidazoles: trapping of intermediates by nucleophiles, Tetrahedron, 1981, 37, 191–200 CrossRef.
- F. Jin, J. Leitich and C. von Sonntag, The photolysis (λ = 254 nm) of tyrosine in aqueous solutions in the absence and presence of oxygen. The reaction of tyrosine with singlet oxygen, J. Photochem. Photobiol., A, 1995, 92, 147–153 CrossRef CAS.
- A. Wright, W. A. Bubb, C. L. Hawkins and M. J. Davies, Singlet oxygen-mediated protein oxidation: evidence for the formation of reactive side chain peroxides on tyrosine residues, Photochem. Photobiol., 2002, 76, 35–46 CrossRef CAS PubMed.
- M. Nakagawa, H. Watanabe, S. Kodato, H. Okajima, T. Hino, J. L. Flippen and B. Witkop, A valid model for the mechanism of oxidation of tryptophan to formylkynurenine-25 years later, Proc. Natl. Acad. Sci. U. S. A., 1977, 74, 4730–4733 CrossRef CAS PubMed.
- C. P. Stanley, G. J. Maghzal, A. Ayer, J. Talib, A. M. Giltrap, S. Shengule, K. Wolhuter, Y. Wang, P. Chadha, C. Suarna, O. Prysyazhna, J. Scotcher, L. L. Dunn, F. M. Prado, N. Nguyen, J. O. Odiba, J. B. Baell, J.-P. Stasch, Y. Yamamoto, P. Di Mascio, P. Eaton, R. J. Payne and R. Stocker, Singlet molecular oxygen regulates vascular tone and blood pressure in inflammation, Nature, 2019, 566, 548–552 CrossRef CAS PubMed.
- C. P. Stanley and R. Stocker, Regulation of vascular tone and blood pressure by singlet molecular oxygen in inflammation, Curr. Opin. Nephrol. Hypertens., 2021, 30, 145–150 CrossRef CAS PubMed.
- M. Ehrenshaft, B. Zhao, U. P. Andley, R. P. Mason and J. E. Roberts, Immunological detection of N-formylkynurenine in porphyrin-mediated photooxided lens α-crystallin, Photochem. Photobiol., 2011, 87, 1321–1329 CrossRef CAS PubMed.
- E. F. Marques, M. H. G. Medeiros and P. Di Mascio, Lysozyme oxidation by singlet molecular oxygen: peptide characterization using [18O]-labeling oxygen and nLC-MS/MS, J. Mass Spectrom., 2017, 52, 739–751 CrossRef CAS PubMed.
- J. Kim, M. E. Rodriguez, M. Guo, M. E. Kenney, N. L. Oleinick and V. E. Anderson, Oxidative modification of cytochrome c by singlet oxygen, Free Radicals Biol. Med., 2008, 44, 1700–1711 CrossRef CAS PubMed.
- F. Leinisch, M. Mariotti, M. Rykaer, C. Lopez-Alarcon, P. Hagglund and M. J. Davies, Peroxyl radical- and photo-oxidation of glucose 6-phosphate dehydrogenase generates cross-links and functional changes via oxidation of tyrosine and tryptophan residues, Free Radicals Biol. Med., 2017, 112, 240–252 CrossRef CAS PubMed.
- J. Fiedor and K. Burda, Potential role of carotenoids as antioxidants in human health and disease, Nutrients, 2014, 6, 464–488 CrossRef PubMed.
- R. Edge, D. J. McGarvey and T. G. Truscott, The carotenoids as anti-oxidants – a review, J. Photochem. Photobiol., B, 1997, 41, 189–200 CrossRef CAS PubMed.
- S. P. Stratton, W. H. Schaefer and D. C. Liebler, Isolation and identification of singlet oxygen oxidation products of beta-carotene, Chem. Res. Toxicol., 1993, 6, 542–547 Search PubMed.
- R. Yamauchi, K. Tsuchihashi and K. Kato, Oxidation products of β-carotene during the peroxidation of methyl linoleate in the bulk phase, Biosci., Biotechnol., Biochem., 1998, 62, 1301–1306 CrossRef CAS PubMed.
- P. Di Mascio, T. P. Devasagayam, S. Kaiser and H. Sies, Carotenoids, tocopherols and thiols as biological singlet molcular oxygen quenchers, Biochem. Soc. Trans., 1990, 18, 1054–1056 CrossRef CAS PubMed.
- K. Aizawa, Y. Iwasaki, A. Ouchi, T. Inakuma, S. Nagaoka, J. Terao and K. Mukai, Development of singlet absorption capacity (SOAC) assay methods. 2. Measurements of SOAC values for carotenoids and food extracts, J. Agric. Food Chem., 2011, 59, 3717–3729 CrossRef CAS PubMed.
- A. Cantrell, D. J. McGarvey, T. G. Truscott, F. Rancan and F. Bohm, Singlet oxygen quenching by dietary carotenoids in a model membrane environment, Arch. Biochem. Biophys., 2003, 412, 47–54 CrossRef CAS PubMed.
- C. S. Foote, Y. C. Chang and R. W. Denny, Chemistry of singlet oxygen. X. Carotenoid quenching parallels biological protection, J. Am. Chem. Soc., 1970, 92, 5216–5218 CrossRef CAS PubMed.
- K. Fukuzawa, Y. Inokami, A. Tokumura, J. Terao and A. Suzuki, Rate constants for quenching singlet oxygen and activities for inhibiting lipid peroxidation of carotenoids and alpha-tocopherol in liposomes, Lipids, 1998, 33, 751–756 CrossRef CAS PubMed.
- M. Jemiola-Rzeminska, M. Pasenkiewicz-Gierula and K. Strzalka, The behavior of β-carotene in the phosphatidylcholine bilayer as revealed by a molecular simulation study, Chem. Phys. Lipids, 2005, 135, 27–37 CrossRef CAS PubMed.
- W. I. Gruszecki and K. Strzalka, Carotenoids as modulators of lipid membrane physical properties, Biochim. Biophys. Acta, 2005, 1740, 108–115 CrossRef CAS PubMed.
- J. Cerezo, J. Zuniga, A. Bastida, A. Requena and J. P. Ceron-Carrasco, Conformational changes of β-carotene and zeaxanthin immersed in a model membrane through atomistic molecular dynamics simulations, Phys. Chem. Chem. Phys., 2013, 15, 6527–6538 RSC.
- G. N. Bosio, T. Breitenbach, J. Parisi, M. Reigosa, F. H. Blaikie, B. W. Pedersen, E. F. F. Silva, D. O. Martire and P. R. Ogilby, Antioxidant β-carotene does not quench singlet oxygen in mammalian cells, J. Am. Chem. Soc., 2013, 135, 272–279 CrossRef CAS PubMed.
- E. Niki, Antioxidants: Basic principles, emerging concepts, and problems, Biomed. J., 2014, 37, 106–111 Search PubMed.
- N. I. Krinsky, Antioxidant functions of carotenoids, Free Radicals Biol. Med., 1989, 7, 617–635 CrossRef CAS PubMed.
- N. I. Krinsky and K.-J. Yeum, Carotenoid-radical interactions, Biochem. Biophys. Res. Commun., 2003, 305, 754–760 CrossRef CAS PubMed.
- H. Tsuchihashi, M. Kigoshi, M. Iwatsuki and E. Niki, Action of beta-carotene as an antioxidant against lipid peroxidation, Arch. Biochem. Biophys., 1995, 323, 137–147 CrossRef CAS PubMed.
- M. Takashima, M. Shichiri, Y. Hagihara, Y. Yoshida and E. Niki, Capacity of peroxyl radical scavenging and inhibition of lipid peroxidation by β-carotene., lycopene and commercial tomato juice, Food Funct., 2012, 3, 1153–1160 RSC.
- A. El-Agamey and D. J. McGarvey, Evidence for a lack of reactivity of carotenoid addition radicals towards oxygen; a laser flash photolysis study of the reactions of carotenoids with acylperoxyl radicals in polar and non-polar solvents, J. Am. Chem. Soc., 2003, 125, 3330–3340 CrossRef CAS PubMed.
- D. Ribeiro, M. Freitas, A. M. S. Silva, F. Carvalho and E. Fernandes, Antioxidant and pro-oxidant activities of carotenoids and their oxidation products, Food Chem. Toxicol., 2018, 120, 681–699 CrossRef CAS PubMed.
- F. Boehm, R. Edge, T. G. Truscott and C. Witt, A dramatic effect of oxygen on protection of human cells against γ-radiation by lycopene, FEBS
Lett., 2016, 590, 1086–1093 CrossRef CAS PubMed.
- E.-S. Hwang and P. E. Bowen, Effects of lycopene and tomato paste extracts on DNA and lipid oxidation in LNCaP human prostate cancer cell, BioFactors, 2005, 23, 97–105 CrossRef CAS PubMed.
- A. Mortensen, L. H. Skibsted and T. G. Truscott, The interaction of dietary carotenoids with radical species, Arch. Biochem. Biophys., 2001, 385, 13–19 CrossRef CAS PubMed.
- H. S. Black, F. Boehm, R. Edge and T. G. Truscott, The benefits and risks of certain dietary carotenoids that exhibit both anti-and pro-oxidative mechanisms-a comprehensive review, Antioxidants, 2020, 9, 264 CrossRef CAS PubMed.
- A. Mortensen, L. H. Skibsted, A. Willnow and S. A. Everett, Re-appraisal of the tocopheroxyl radical reaction with beta-carotene: evidence for oxidation of vitamin E by the beta-carotene radical cation, Free Radic. Res., 1998, 28, 69–80 CrossRef CAS PubMed.
- M. Burke, R. Edge, E. J. Land and T. G. Truscott, Characterization of carotenoid radical cations in liposomal environments: interaction with vitamin C, J. Photochem. Photobiol., B, 2001, 60, 1–6 CrossRef CAS PubMed.
- R. Radi, Peroxynitrite, a stealthy biological oxidant, J. Biol. Chem., 2013, 288(37), 26464–26472 CrossRef CAS PubMed.
- K. Kikugawa, K. Hiramoto, S. Tomiyama and Y. Asano, β-Carotene effectively scavenge toxic nitrogen oxides: nitrogen dioxide and peroxynitrous acid, FEBS Lett., 1997, 404, 175–178 CrossRef CAS PubMed.
- R. Scheidegger, A. K. Pande, P. L. Bounds and W. H. Koppenol, The reaction of peroxynitrite with zeaxanthin, Nitric Oxide, 1998, 2, 8–16 CrossRef CAS PubMed.
- T. Yokota, T. Otake, H. Ishikawa, T. Inakuma, Y. Ishiguro, J. Terao, A. Nagao and H. Etoh, Quenching of peroxynitrite by lycopene in vitro, Chem. Lett., 2004, 33, 80–81 CrossRef CAS.
- A. M. Hegazy, E. M. El-Sayed, K. S. Ibrahim and A. S. Abdel-Azeem, Dietary antioxidant for disease prevention corroborated by the Nrf2 pathway, J. Complementary Integr. Med., 2019, 16, 20180161 Search PubMed.
- A. Kaulmann and T. Bohn, Carotenoids, inflammation, and oxidative stress-implications of cellular signaling pathways and relation to chronic disease prevention, Nutr. Res., 2014, 34, 907–929 CrossRef CAS PubMed.
- M. P. Barros, M. J. Rodrigo and L. Zacarias, Dietary carotenoid roles in redox homeostasis and human health, J. Agric. Food Chem., 2018, 66, 5733–5740 CrossRef CAS PubMed.
- T. Suzuki and M. Yamamoto, Stress-sensing mechanisms and the physiological roles of the Keap1-Nrf2 system during cellular stress, J. Biol. Chem., 2017, 292, 16817–16824 CrossRef CAS PubMed.
- L. Baird and M. Yamamoto, The molecular mechanism regulating the KEAP1-NRF2 pathway, Mol. Cell. Biol, 2020, 40, e00099-20 CrossRef PubMed.
- Y. Inoue, M. Shimazawa, R. Nagano, Y. Kuse, K. Takahashi, K. Tsuruma, M. Hayashi, T. Ishibashi, T. Maoka and H. Hara, Astaxanthin analogues, adonixanthin and lycopene, activate Nrf2 to prevent light-induced photoreceptor degeneration, J. Pharmacol. Sci., 2017, 134, 147–157 CrossRef CAS PubMed.
- K. Frede, F. Ebert, A. P. Kipp, T. Schwerdtle and S. Baldermann, Lutein activates the transcription factor Nrf2 in human retinal pigment epithelial cells, J. Agric. Food Chem., 2017, 65, 5944–5952 CrossRef CAS PubMed.
- T. Liu, W.-H. Liu, J.-S. Zhao, F.-Z. Meng and H. Wang, Lutein protects against β-amyloid peptide-induced oxidative stress in cerebrovascular endothelial cells through modulation of Nrf-2 and NF-κB, Cell Biol. Toxicol., 2017, 33, 57–67 CrossRef CAS PubMed.
- X. Zou, J. Gao, Y. Zheng, X. Wang, C. Chen, K. Cao, J. Xu, Y. Li, W. Lu, J. Liu and Z. Feng, Zeaxanthin induces Nrf2-mediated phase-II
enzymes in protection of cell death, Cell Death Dis., 2014, 5, e1218 CrossRef CAS PubMed.
- Y. Zhang, H. Mao, Y. Li, Y. Xiong, X. Liu, L. Wang and Z. Chen, β-Cryptoxanthin maintains mitochondrial function by promoting NRF2 nuclear translocation to inhibit oxidative stress-induced senescence in HK-2 cells, Int. J. Mol. Sci., 2023, 24, 3851 CrossRef CAS PubMed.
- P. Chen, L. Li, Y. Gao, Z. Xie, Y. Zhang, Z. Pan, Y. Tu, H. Wang, Q. Han, X. Hu and X. Xin, β-Carotene provides neuro protection after experimental traumatic brain injury via the Nrf2-ARE pathway, J. Integr. Neurosci., 2019, 18, 153–161 CrossRef PubMed.
- Y. Wang, L. M. Ausman, A. S. Greenberg, R. M. Russell and X.-D. Wang, Dietary lycopene and tomato extract supplementations inhibit nonalcoholic steatohepatitis-promoted hepatocarcinogenesis in rats, Int. J. Cancer, 2010, 126, 1788–1796 CrossRef CAS PubMed.
- B. Zhao, B. Ren, R. Guo, W. Zhang, S. Ma, Y. Yao, T. Yuan, Z. Liu and X. Liu, Supplementation of lycopene attenuates oxidative stress induced neuroinflammation and cognitive impairment via Nrf2/NF-κB transcriptional pathway, Food Chem. Toxicol., 2017, 109, 505–516 CrossRef CAS PubMed.
- K. Yu, J. Zhang, Z. Cao, Q. Ji, Y. Han, M. Song, B. Shao and Y. Li, Lycopene attenuates AFB1-induced renal injury with the activation of the Nrf2 antioxidant signaling pathway in mice, Food Funct., 2018, 9, 6427–6434 RSC.
- Q. Zhao, F. Yang, L. Meng, D. Chen, M. Wang, X. Lu, D. Chen, Y. Jiang and N. Xing, Lycopene attenuates chronic prostatitis/chronic pelvic pain syndrome by inhibiting oxidative stress and inflammation via the interaction of NF-κB, MAPKs, and Nrf2 signaling pathways in rats, Andrology, 2020, 8, 747–755 CrossRef CAS PubMed.
- R. Xue, J. Qiu, S. Wei, M. Liu, Q. Wang, P. Wang, B. Sha, H. Wang, Y. Shi, J. Zhou, J. Rao and L. Lu, Lycopene alleviates hepatic ischemia reperfusion injury via the Nrf2/HO-1 pathway mediated NLRP3 inflammasome inhibition in Kupffer cells, Ann. Transl. Med., 2021, 9, 631 CrossRef CAS PubMed.
- Y. Zhao, M.-Z. Li, Y. Shen, J. Lin, H.-R. Wang, M. Talukder and J.-L. Li, Lycopene prevents DEHP-induced Leydig cell damage with the Nrf2 antioxidant signaling pathway in mice, J. Agric. Food Chem., 2020, 68, 2031–2040 CrossRef CAS PubMed.
- S. Li, Y. Ding, Q. Niu, S. Xu, L. Pang, R. Ma, M. Jing, G. Feng, J. X. Tang, Q. Zhang, X. Ma, Y. Yan, J. Zhang, M. Wei, H. X. Wang, F. Li and S. Guo, Lutein has a protective effect on hepatotoxicity induced by arsenic via Nrf2 signaling, BioMed. Res. Int., 2015, 315205 Search PubMed.
- H. Li, C. Huang, J. Zhu, K. Gao, J. Fang and H. Li, Lutein suppresses oxidative stress and inflammation by Nrf2 activation in an osteoporosis rat model, Med. Sci. Monit., 2018, 24, 5071–5075 CrossRef CAS PubMed.
- K. Sahin, C. Orhan, F. Akdemir, M. Tuzcu, N. Sahin, I. Yilmaz and V. Juturu, β-Cryptoxanthin ameliorates metabolic risk factors by regulating NF-κB and Nrf2 pathways in insulin resistance induced by high-fat diet in rodents, Food Chem. Toxicol., 2017, 107, 270–279 CrossRef CAS PubMed.
- X.-D. Wang, Lycopene metabolism and its biological significance, Am. J. Clin. Nutr., 2012, 96, 1214S–1422S CrossRef CAS PubMed.
- F. Lian and X.-D. Wang, Enzymatic metabolites of lycopene induce Nrf-2 mediated expression of phase II detoxifying/antioxidant enzymes in human bronchial epithelial cells, Int. J. Cancer, 2008, 123, 1262–1268 CrossRef CAS PubMed.
- T. Bohn, Bioactivity of carotenoids-chasms of knowledge, Int. J. Vitam. Nutr. Res., 2017, 87, 5–9 CrossRef CAS PubMed.
- T. Bohn, A. R. de Lera, J. F. Landrier and R. Ruhl, Carotenoid metabolites, their tissue and blood concentrations in human and further bioactivity via retinoid receptor-mediated signaling, Nutr. Res. Rev., 2022, 16, 1–14 Search PubMed.
- K. Linnewiel, H. Ernst, C. Caris-Veyrat, A. Ben-Dor, A. Kampf, H. Salman, M. Danilenko, J. Levy and Y. Sharoni, Structure activity relationship of carotenoid derivatives in activation of the electrophile/antioxidant response element transcription system, Free Radicals Biol. Med., 2009, 47, 659–667 CrossRef CAS PubMed.
- L. Wu, X. Guo, W. Wang, D. M. Medeiros, S. L. Clarke, E. A. Lucas, B. J. Smith and D. Lin, Molecular aspects of β,β-carotene-9′,10′-oxygenase 2 in carotenoid metabolism and diseases, Exp. Biol. Med., 2016, 241, 1879–1887 CrossRef CAS PubMed.
- C. J. Thomas, R. G. Mirza and M. K. Gill, Age-related macular degeneration, Med. Clin. North Am., 2021, 105, 473–491 CrossRef PubMed.
- P. Mitchel, G. Liew, B. Gopinath and T. Y. Wong, Age-related macular degeneration, Lancet, 2018, 392, 1147–1159 CrossRef PubMed.
- Y. Ozawa, S. Ishida and K. Tsubota, Age-related macular degeneration (AMD); from pathogenesis and approved therapies to proposed treatments for prevention, Anti-Aging Med., 2008, 5, 87–92 CrossRef.
- F. Khachick, F. F. de Moura, D.-Y. Zhao, C.-P. Aebischer and P. S. Bernstein, Transformations of selected carotenoids in plasma, liver and ocular tissues of humans and in nonprimate animal models, Invest. Ophthalmol. Visual Sci., 2002, 43, 3383–3392 Search PubMed.
- N. I. Krinsky, J. T. Landrum and R. A. Bone, Biological mechanism of the protective role of lutein and zeaxanthin in the eye, Annu. Rev. Nutr., 2003, 23, 171–201 CrossRef CAS PubMed.
- J. T. Landrum and R. A. Bone, Lutein, zeaxanthin, and the macular pigment, Arch. Biochem. Biophys., 2001, 385, 28–40 CrossRef CAS PubMed.
- R. A. Bone and J. T. Landrum, Dose-dependent response of serum lutein and macular pigment optical density to supplementation with lutein esters, Arch. Biochem. Biophys., 2010, 504, 50–55 CrossRef CAS PubMed.
- J. T. Landrum, R. A. Bone and M. D. Kilburn, The macular pigment: a possible role in protection from age-related macular degeneration, Adv. Pharmacol., 1996, 38, 537–556 Search PubMed.
- J. M. Stringham and B. R. Hammond Jr., Dietary lutein and zeaxanthin: possible effects on visual function, Nutr. Rev., 2005, 63, 59–64 CrossRef PubMed.
- R. Arunkumar, C. M. Calvo, C. D. Conrady and P. S. Bernstein, What do we know about the macular pigment in AMD: the past, the present, and the future, Eye, 2018, 32, 992–1004 CrossRef PubMed.
- R. Arunkmar, A. Gorusupudi and P. S. Bernstein, The macular carotenoids: A biochemical overview, Biochim. Biophys. Acta, Mol. Cell Biol. Lipids, 2020, 1865, 158617 CrossRef PubMed.
- F. Khachik, P. S. Bernstein and D. L. Garland, Identification of lutein and zeaxanthin oxidation products in human and monkey retinas, Invest. Ophthalmol. Visual Sci., 1997, 38, 1802–1811 CAS.
- S. Richer, AEMD-pilot (case series) environmental intervention data, J. Am. Optom. Assoc., 1990, 70, 24–36 Search PubMed.
- G. Dagnelie, I. S. Zorge and T. M. McDonald, Lutein improves visual function in some patients with retinal degeneration: a pilot study via the Internet, Optometry, 2000, 71, 147–164 CAS.
- S. Richer, W. Stiles, L. Statkute, J. Pulido, J. Frankowski, D. Rudy, K. Pei, M. Tsipursky and J. Nyland, Double-masked, placebo-controlled, randomized trial of lutein and antioxidant supplementation in the intervention of atrophic age-related macular degeneration: the veterans LAST study (Lutein Antioxidant Supplementation Trial), Optometry, 2004, 75, 216–230 CrossRef PubMed.
- T. S. Aleman, J. L. Duncan, M. L. Bieber, E. de Castro, D. A. Marks, L. M. Gardner, J. D. Steinberg, A. V. Cideciyan, M. G. Maguire and S. G. Jacobson, Macular pigment and lutein supplementation in retinitis pigmentosa and Usher syndrome, Invest. Ophthalmol. Visual Sci., 2001, 42, 1873–1881 CAS.
- H. Bahrami, M. Melia and G. Dagnelie, Lutein supplementation in retinitis pigmentosa: PC-based vision assessment in a randomized double-masked placebo-controlled clinical trial [NCT00029289], BMC Ophthalmol., 2006, 6, 23 CrossRef PubMed.
- S. P. Richer, W. Stiles, K. Graham-Hoffman, M. Levin, D. Ruskin, J. Wrobel, D.-W. Park and C. Thomas, Randomized, double-blind, placebo-controlled study of zeaxanthin and visual function in patients with atrophic age-related macular degeneration: the zeaxanthin and visual function study (ZVF) FDA IND#78,973, Optometry, 2011, 82, 667–680 CrossRef PubMed.
- T. J. McGill, L. M. Renner and M. Neuringer, Elevated fundus autofluorescence in monkeys deficient in lutein, zeaxanthin and omega-3 fatty acids, Invest. Ophthalmol. Visual Sci., 2016, 57, 1361–1369 CrossRef PubMed.
- Age-Related Eye Disease Study Research Group, A randomized, placebo-controlled, clinical trial of high-dose supplementation with vitamins C and E, β-carotene, and zinc for age-related macular degeneration and vision loss, Arch. Ophthalmol., 2001, 119, 1417–1436 CrossRef PubMed.
- Age-Related Eye Disease Study Research Group, Lutein + zeaxanthin and omega-3 fatty acids for age-related macular degeneration: The age-related Eye disease study 2 (AREDS2) randomized clinical trial, J. Am. Med. Assoc., 2013, 309, 2005–2015 CrossRef PubMed.
- E. Y. Chew, T. E. Clemons, E. Agron, A. Dormalpally, T. D. L. Keenan, S. Vitale, C. Weber, D. C. Smith and W. Christen, Long-term outcomes of adding lutein/zeaxanthin and w-3 fatty acids to the AREDS supplements on age-related macular degeneration progression: AREDS2 report 28, JAMA Ophthalmol., 2022, 140, 692–698 CrossRef PubMed.
- H. Masaki, T. Atumi and H. Sakurai, Detection of hydrogen peroxide and hydroxyl radicals in murine skin fibroblasts under UVB irradiation, Biochem. Biophys. Res. Commun., 1995, 206, 474–479 CrossRef CAS PubMed.
- G. T. Wondrak, M. K. Jacobson and E. L. Jacobson, Endogenous UVA-photosensitizers: mediators of skin photodamage and novel targets for skin photoprotection, Photochem. Photobiol. Sci., 2006, 5, 215–237 CrossRef CAS PubMed.
- W. Baumler, J. Regensburger, A. Knak, A. Felgentrager and T. Maisch, UVA and endogenous photosensitizers – the detection of singlet oxygen by its luminescence, Photochem. Photobiol. Sci., 2012, 11, 107–117 CrossRef PubMed.
- F. Liebel, S. Kaur, E. Ruvolo, N. Kollias and M. D. Southall, Irradiation of skin with visible light induces reactive oxygen species and matrix-degrading enzymes, J. Invest. Dermatol., 2012, 132, 1901–1907 CrossRef CAS PubMed.
- M. S. Driscoll and R. F. Wagner Jr., Clinical management of the acute sunburn reaction, Cutis, 2000, 66, 53–58 CAS.
- M. E. Darvin, W. Sterry, J. Lademann and T. Vergou, The role of carotenoids in human skin, Molecules, 2011, 16, 10491–10506 CrossRef.
- W. Stahl, U. Heinrich, H. Jungmann, J. von Larr, M. Schietzel, H. Sies and H. Tronnier, Increased dermal carotenoid levels assessed by noninvasive reflection spectrophotometry correlate with serum levels in women ingesting Betatene, J. Nutr., 1998, 128, 903–907 CrossRef CAS PubMed.
- H. Sies and W. Stahl, Carotenoids and UV protection, Photochem. Photobiol. Sci., 2004, 3, 749–752 CrossRef CAS PubMed.
- W. Stahl, U. Heinrich, H. Jungmann, H. Sies and H. Tronnier, Carotenoids and carotenoids plus vitamin E protect against ultraviolet light-induced erythema in humans, Am. J. Clin. Nutr., 2000, 71, 795–798 CrossRef CAS PubMed.
- U. Heinrich, C. Gartner, M. Wiebusch, O. Eichler, H. Sies, H. Tronnier and W. Stahl, Supplementation with β-carotene or a similar amount of mixed carotenoids protects humans from UV-induced erythema, J. Nutr., 2003, 133, 98–101 CrossRef CAS PubMed.
- W. Stahl, U. Heinrich, S. Wiseman, O. Eichler, H. Sies and H. Tronnier, Dietary tomato paste protects against ultraviolet light-induced erythema in humans, J. Nutr., 2001, 131, 1449–1451 CrossRef CAS PubMed.
- M. Rizwan, I. Rodriguez-Blanco, A. Harbottle, M. A. Birch-Machin, R. E. B. Watson and L. E. Rhodes, Tomato paste rich in lycopene protects against cutaneous photodamage in human in vivo: a randomized controlled trial, Br. J. Dermatol., 2011, 164, 154–162 CrossRef CAS PubMed.
- M. C. Meinke, A. Friedrich, K. Tscherch, S. F. Haag, M. E. Darvin, H. Vollert, N. Groth, J. Lademann and S. Rohn, Influence of dietary carotenoids on radical scavenging capacity of the skin and skin lipids, Eur. J. Pharm. Biopharm., 2013, 84, 365–373 CrossRef CAS PubMed.
- T. M. Callaghan and K.-P. Wilhelm, A review of ageing and examination of clinical methods in the assessment of ageing skin. Part I: Cellular and molecular perspectives of skin ageing, Int. J. Cosmet. Sci., 2008, 30, 313–322 CrossRef CAS PubMed.
- M. Wlaschek, K. Briviba, G. P. Striklin, H. Sies and K. Scharffetter-Kochanek, Singlet oxygen may mediate the ultraviolet A-induced synthesis of interstitial collagenase, J. Invest. Dermatol., 1995, 104, 194–198 CrossRef CAS PubMed.
- T. Polte and R. M. Tyrrell, Involvement of lipid peroxidation and organic peroxides in UVA-induced metalloproteinase-1 expression, Free Radicals Biol. Med., 2004, 36, 1566–1574 CrossRef CAS PubMed.
- Y. Minami, K. Kawabata, Y. Kubo, S. Arase, K. Hirasaka, T. Nikawa, N. Bando, Y. Kawai and J. Terao, Peroxidized cholesterol-induced matrix metalloproteinase-9 activation and its suppression by dietary β-carotene in photoaging of hairless mouse skin, J. Nutr. Biochem., 2009, 20, 389–398 CrossRef CAS PubMed.
- J. Terao, Y. Minami and N. Bando, Singlet molecular oxygen-quenching activity of carotenoids: relevance to protection of the skin from photoaging, J. Clin. Biochem. Nutr., 2011, 48, 57–62 CrossRef CAS PubMed.
- S. Zerres and W. Stahl, Carotenoids in human health, Biochim. Biophys. Acta, Mol. Cell Biol. Lipids, 2020, 1865, 158588 CrossRef CAS PubMed.
- M. Stacewicz-Sapuntzakis and P. E. Bowen, Role of lycopene and tomato products in prostate health, Biochim. Biophys. Acta, 2005, 1740, 202–205 CrossRef CAS PubMed.
- R. Chan, K. Lok and J. Woo, Prostate cancer and vegetable consumption, Mol. Nutr. Food Res., 2009, 53, 201–216 CrossRef CAS PubMed.
- V. A. Kirsh, S. T. Mayn, U. Peters, N. Chatterjee, M. F. Leitzmann, L. B. Dixon, D. A. Urban, E. D. Crawford and R. B. Hayes, A prospective study of lycopene and tomato product intake and risk of prostate cancer, Cancer Epidemiol. Biomarkers Prev., 2006, 15, 92–98 CrossRef CAS PubMed.
- D. Ilic, K. M. Forbes and C. Hassed, Lycopene for the prevention of prostate cancer, Cochrane Database Syst. Rev., 2011, 11, CD008007 Search PubMed.
- J. Dulinska-Litewka, P. Halubiec, A. Lazarczyk, O. Szafranski, Y. Sharoni, J. A. McCubrey, B. Gasiorkiewicz and T. Bohn, Recent progress in discovering the role of carotenoids and metabolites in prostatic physiology and pathology – A review – Part II: Carotenoids in the human studies, Antioxidants, 2021, 10, 319 CrossRef CAS PubMed.
- A. Kapala, M. Szlendak and E. Motacka, The anti-cancer activity of lycopene: a systematic review of human and animal studies, Nutrients, 2022, 14, 5152 CrossRef CAS PubMed.
- W. Stahl, W. Schwarz, A. R. Sundquist and H. Sies, Cis-trans isomers of lycopene and beta-carotene in human serum and tissues, Arch. Biochem. Biophys., 1992, 294, 173–177 CrossRef CAS PubMed.
- N. P. Gupta and R. Kumar, Lycopene therapy in idiopathic male infertility – a preliminary report, Int. Urol. Nephrol., 2002, 34, 369–372 CrossRef CAS PubMed.
- Y. Yamamoto, K. Aizawa, M. Mieno, M. Karamatsu, Y. Hirano, K. Furui, T. Miyashita, K. Yamazaki, T. Inakuma, I. Sato, H. Suganuma and T. Iwamoto, The effects of tomato juice on male infertility, Asia Pac. J. Clin. Nutr., 2017, 26, 65–71 CAS.
- J. C. Ribeiro, R. Nogueira-Ferreira, F. Amado, M. G. Alves, R. Ferreira and P. F. Oliveira, Exploring the role of oxidative stress in sperm motility: A proteomic network approach, Antioxid. Redox Signal., 2022, 37, 501–520 CrossRef CAS PubMed.
- A. Bahonar, M. Saadatnia, F. Khorvash, M. Maracy and A. Khosravi, Carotenoids as potential antioxidant agents in stroke prevention: A systematic review, Int. J. Prev. Med., 2017, 8, 70 CrossRef PubMed.
- A. Otocka-Kmiecik, Effect of carotenoids on paraoxonase-1 activity and gene expression, Nutrients, 2022, 14, 2842 CrossRef CAS PubMed.
- M. Fathalipour, H. Fathalipour, O. Safa, P. Nowrouzi-Sohrabi, H. Mirkhani and S. Hassanipour, The therapeutic role of carotenoids in diabetic retinopathy: A systematic review, Diabetes, Metab. Syndr. Obes.: Targets Ther., 2020, 13, 2347–2358 CrossRef CAS PubMed.
- L. I. Elvira-Torales, J. Garcia-Alonso and M. J. Periago-Caston, Nutritional importance of carotenoids and their effect on liver health: A review, Antioxidants, 2019, 8, 229 CrossRef CAS PubMed.
- R. D. Clugston, Carotenoids and fatty liver disease: current knowledge and research gaps, Biochim. Biophys. Acta, Mol. Cell Biol. Lipids, 2020, 1865, 158597 CrossRef CAS PubMed.
- Q. Yu, F. Xue, Z. Li, X. Li, L. Ai, M. Jin, M. Xie and Y. Yu, Dietary intake of carotenoids and risk of depressive symptoms; A systematic review and meta-analysis, Antioxidants, 2022, 11, 2205 CrossRef CAS PubMed.
- T. Bohn, Carotenoids and markers of oxidative stress in human observational studies and intervention trials: implication for chronic diseases, Antioxidants, 2019, 8, 179 CrossRef CAS PubMed.
- H. Sies, Oxidative stress: concept and some practical aspects, Antioxidants, 2020, 9, 852 CrossRef CAS PubMed.
- K. Murotomi, A. Umeno, M. Shichiri, M. Tanito and Y. Yoshida, Significance of singlet oxygen molecule in pathologies, Int. J. Mol. Sci., 2023, 24, 2739 CrossRef CAS PubMed.
- J. Fujii, Y. Soma and Y. Matsuda, Biological action of singlet molecular oxygen from the standpoint of cell signaling, injury and Death, Molecules, 2023, 28, 4085 CrossRef CAS PubMed.
|
This journal is © The Royal Society of Chemistry 2023 |