DOI:
10.1039/D2CC06286K
(Feature Article)
Chem. Commun., 2023,
59, 3024-3039
Fluorophore-based host–guest assembly complexes for imaging and therapy
Received
20th November 2022
, Accepted 26th January 2023
First published on 30th January 2023
Abstract
Recently, supramolecular chemistry with its unique properties has received considerable attention in many fields. Supramolecular fluorescent systems constructed on the basis of macrocyclic hosts are not only effective in overcoming the limitations of imaging and diagnostic reagents, but also in enhancing their performances. This paper summarizes the recent advances in supramolecular fluorescent systems based on host–guest interactions and their application in bioimaging and therapy as well as the challenges and prospects in developing novel supramolecular fluorescent systems.
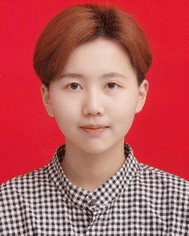
Qian Wu
| Qian Wu is currently a PhD candidate in Chemistry at Hunan University under the supervision of Prof. Lin Yuan. She received her BS degree from Chengdu University of technology in 2018. Her current research focuses on the design of functional supramolecular fluorophores for imaging and cancer therapy. |
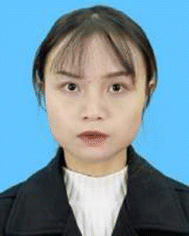
Qian Lei
| Qian Lei received a master's degree in Pharmaceutical Sciences from Central South University in 2022. Then, she started her doctoral work at Hunan University under the supervision of Prof. Lin Yuan in the area of Molecular Imaging. |
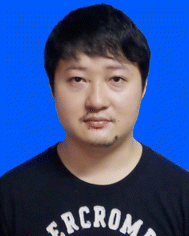
Yao Sun
| Yao Sun is a Professor at Central China Normal University, China. His research interests mainly focus on the synthesis of emissive supramolecules for chemical biology, fluorescence imaging, bioimaging and phototherapy. |
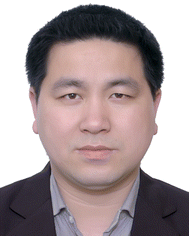
Lin Yuan
| Lin Yuan is a Professor at Hunan University, China. He received his PhD degree from Hunan University and later worked as a postdoctoral researcher at the National University of Singapore. His research mainly focuses on the design and synthesis of fluorescent dyes and probes for sensing and imaging. |
1. Introduction
Supramolecular chemistry, defined as “chemistry beyond molecules”, focuses on the study of complex, ordered and functionally specific assemblies of molecules bonded by non-covalent bonds with weak interactions (e.g. H bonds, π–π interactions, electrostatic interactions, van der Waals interactions, etc.).1 Since the Nobel Prize in Chemistry was awarded to the three scientists C. J. Pedersen, D. J. Cram and J.-M. Lehn in 1987, the importance of supramolecular chemistry has gradually been gradually recognized by researchers.2 Unlike molecular chemistry, which concentrates on covalent bonding, supramolecular chemistry is devoted to the exploration of relatively weak non-covalent interactions between molecules. Non-covalent interactions have the inherent properties of being dynamic and reversible,3 which is like a double-edged sword. On the one hand, stimulus-responsive supramolecular materials can be easily constructed in special microenvironments; on the other hand, the dynamic and reversible non-covalent interactions make supramolecular materials prone to dissociation or reconstruction in complex biological environments. In recent years, supramolecular chemistry has been integrated with many fields such as physics, chemistry, nanotechnology, biology and materials, and gradually developed into a highly interdisciplinary new discipline.4,5 In particular, the host–guest chemistry, which is part of supramolecular chemistry, has greatly influenced the development and application of dye chemistry in the past decade.6–9 In this review, we detail this 'major influence' and its latest manifestations (Fig. 1).
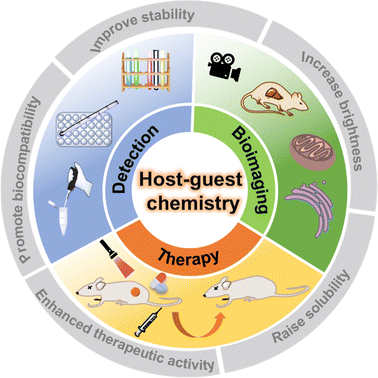 |
| Fig. 1 The biomedical applications of supramolecular fluorescent systems based on host–guest interactions. | |
Host–guest chemistry is the study of the host, guest and host–guest complexes involved in the process of host–guest complexation. To date, five classical synthetic macrocyclic molecules (Fig. 2), including crown ethers,10 cyclodextrins (CDs),11,12 calix[n]arene (CAs),13,14 cucurbit[n]uril (CBs)15,16 and pillar[n]arene (PAs),17,18 have been well documented as the main macrocyclic host, while the guest molecules can be a wide variety of ions, dyes, drugs, biomolecules, etc.19,20 Host–guest complexes are formed when two or more molecules or ions combine in a particular structural relationship by non-covalent interactions. As a result, we usually describe this non-covalent interaction as a host–guest interaction. The host–guest interaction is not a specifically fundamental non-covalent interaction, but benefits from the synergy of multiple essential non-covalent interactions, such as hydrophobic or hydrophilic attraction, electrostatic interactions, hydrogen-bonding interactions, ion pairing, π–π stacking interactions, van der Waals force, etc.21 Through host–guest interactions, a supramolecular system can be integrated simply between the macrocyclic host and the functionalized guest dye without cumbersome synthesis steps and complex purification processes.22 And this supramolecular system shows great potential to overcome the limitations of dyes. For example, the chemical- and photo-stability of the dye is significantly improved by the encapsulation of macrocyclic cavities,23 and the hydrophilic outer cavities of the macrocycles greatly enhance the solubility of the guest molecules, leading to good biocompatibility.24 Such host–guest complexes, formed by the combination of two or more chemical groups in a convenient and reversible manner, offer great possibilities for the design and construction of novel supramolecular structures. Accordingly, researchers can select macrocycles with different properties to encapsulate and modify fluorophores according to their needs, thus achieving optimization of fluorophore properties.
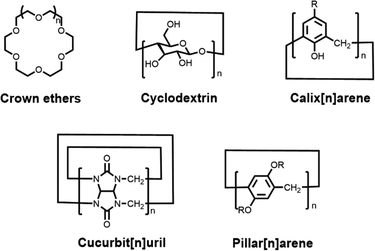 |
| Fig. 2 Chemical structures of five representative macrocyclic hosts. | |
In view of these advantages, macrocyclic-fluorophore supramolecular complexes based on host–guest interactions have currently been extensively developed.25,26 The construction of protein assemblies and DNA–macrocyclic host conjugates has been reviewed in other sections and will not be covered.27,28 Similarly, supramolecular drug systems and supramolecular chemodynamic therapies have been previously reviewed.29,30 Additionally, macrocycle-based materials are also excluded.31,32 Furthermore, for details about the molecular mechanism and sensing system of detection methods, we highly recommend an excellent review published by Biedermann and co-workers in 2022.33 Although there have been many excellent reviews of supramolecular systems in recent years,34,35 few articles have focused on supramolecular fluorescent complexes constructed on the basis of host–guest interactions and their biomedical applications. Accordingly, in this review, we summarize the progress of research on fluorophore-based host–guest complexes and their biomedical applications over the last five years and discuss the challenges and prospects for the future design of novel supramolecular fluorescent systems and the exploration of their potential clinical applications. We sincerely hope that this paper will be of great interest to researchers from various fields, including dye chemistry, supramolecular chemistry, biology and medicine, leading to the design of better performing supramolecular fluorescent materials capable of being used in clinical medicine.
2. Background
2.1. Macrocyclic host molecules
Over the last few decades, a range of macrocyclic molecules and their derivatives have been developed, including calix[n]arene (CAs),13,14 crown ethers,10 cyclodextrins (CDs),11,12 cucurbit[n]uril (CBs),15,16 pillar[n]arene (PAs)17,18 (Fig. 2). These molecules possessing cavities capable of encapsulating the guest are defined as macrocyclic hosts. In particular, cyclodextrins, cucurbit[n]uril and calix[n]arene were increasingly developed and have attracted attention due to their good biocompatibility and modifiability, especially for their application in the biomedical field. Cyclodextrins (CDs),11,12 as second-generation macrocyclic host compounds after crown ethers, are cyclic compounds formed by several D-glucopyranose units linked by α-1,4-glycosidic bonds. Among them, α-, β- and γ-CDs containing 6, 7 and 8 glucose molecular units, respectively, are the most widely studied and applied. On the one hand, the presence of hydroxyl groups on the CDs not only allows for facile chemical modification, but also enables the formation of hydrogen bonds with solvent molecules, providing cyclodextrins with excellent solubility. On the other hand, the interior of the CD cavity offers a hydrophobic region due to the shielding effect of the C–H bond, which is well suited to encapsulate lipid-soluble fluorescent dyes. Hence CD-based host–guest complexes have relatively good stability and can reach a binding constant of 104 M−1.36 As shown in Fig. 2, calix[n]arene (CAs)13,14 are a category of cyclic oligomers consisting of phenol units linked by methylene groups adjacent to phenolic hydroxyl groups. CAs exhibit excellent thermal- and chemical stability, whose synthesis and derivation are easy. One of the disadvantages of CAs is their poor water solubility, which can be further improved by structural modification.37 Most water-soluble CA derivatives contain sulphonic acid groups, thus constituting an upper port with a negative charge density that enables preferential binding to the cationic guest.38 This is a unique advantage for fluorescent dyes, as most common dyes have a positive charge. In contrast, cucurbit[n]uril (CBs),15,16 with a pumpkin shape, are a class of highly symmetrical cage-like macrocyclic compounds linked by methylene bridges with glyburide as the unit. CBs have rigid hydrophobic cavities with port carbonyl groups of a certain polarity (Fig. 2). The diameter of the ports and the size of the cavities vary for different degrees of polymerization. Owing to the uniqueness of the CB structure, the binding constants of CBs to the guest are usually higher than those of other classes of macrocyclic hosts.39 For example, Isaacs and co-workers reported that CB[7] has a binding constant of up to 7.2 × 1017 M−1 with the diamantine quaternary diammonium ion derivative in pure D2O,40 which goes beyond avidin–biotin affinity interactions. Additionally, pillar[n]arene have been extensively investigated in recent years due to their symmetrical structure and tunable functionalization, and therefore, the relevant biomedical applications of pillar[n]arene will also be discussed in this paper.17,18,41
2.2. Properties and applications of fluorophore–macrocycle complexes
In a typical supramolecular host–guest fluorescence system, the host molecule contains a cavity and the functional group as a guest forms supramolecular complexes through host–guest interactions, avoiding the tedious synthesis and complex purification processes. Usually, upon complexation of a fluorescent dye with a macrocyclic compound, the properties of the fluorophore such as stability, fluorescence intensity and biocompatibility are altered to varying degrees,42–44 and this change can also in turn provide a complexation signal between the host and the guest. It is well known that although the optical characteristics of different fluorescent dye molecules differ, they all tend to be susceptible to the environment in which they are placed.45 When the organic dye guest binds with the macrocycles in the hydrophobic cavities, the fluorophores can be protected by the nesting of the macrocyclic cavities, which can effectively prevent exposure of the fluorophores to the external environment (e.g., oxygen and water), thus improving the stability of the corresponding fluorophore.23 The complexation of organic dyes with macrocycles usually changes the fluorescence signal in two ways: first, the migration of the fluorophore into the hydrophobic cavity of the host molecule is able to avoid fluorescence quenching in an aqueous environment;46 second, the free rotation and vibration of the fluorophore are bound by the geometry and cavity of the macrocycle, thus reducing non-radiative attenuation.47 In addition, the aggregation of the dye is hindered due to encapsulation, resulting in a significant enhancement of the physicochemical properties, and more importantly, the water-soluble macrocycles can further enhance the biocompatibility of the complex.
Since the assembly of fluorophores with macrocycles can improve their physicochemical properties, this strategy is currently widely applied not only for the construction of luminescent materials48 and the modulation of fluorophore properties,49,50 but also for designing and developing biomedical reagents.51–55 For example, Cao and co-workers constructed various fluorescent supramolecular organic framework materials56,57 through host–guest interactions, thus controlling and tuning their shape and size. Li et al. successfully developed the supramolecular organic framework that can be employed for nucleic acid delivery58 or to improve the safety of photodynamic therapy reagents and maintain anti-tumour efficacy.59 Besides, many other tunable multicolour luminescent48,60,61, room-temperature phosphorescent62,63 and responsive materials64 were also engineered through host–guest interactions. Simultaneously, due to the inherent cavity of the macrocycles which can well protect the inclusion, Yao and co-workers created a pillar[5]arene-based host–guest system19,65 to improve the stability and fluorescence intensity of the dyes, and Ma et al. used the same approach to brighten and enlarge the emission of Au nanoclusters and for cancer cell imaging.46 Most importantly, owing to the considerable biocompatibility and inherent inclusion of macrocycles, strategies based on host–guest assembly have been widely used in recent years for constructing drug delivery systems,66,67 synthesizing antimicrobial materials,44,68–70 and designing biomedical reagents.71–74 This paper will focus on the development and biological applications of host–guest strategies in fluorophore-based bioimaging and therapeutic reagents and their potential challenges and prospects.
3. Bioimaging and therapy applications
3.1. Bioimaging
Bioimaging provides indispensable information for the early diagnosis of diseases. An ideal bioimaging reagent should satisfy several requirements, including good biocompatibility, robust signal generation, and high chemical-/photo-stability.75 The application of supramolecular fluorescent systems to bioimaging can enhance the properties of the bioimaging reagents owing to their high brightness and insensitivity to the micro-environment, resulting in the successful visualization of live cells, tissues and organisms.7 As a kind of traditional dye, porphyrins are widely used in imaging, diagnosis, and treatment of diseases due to their excellent photophysical properties.76 However, porphyrins tend to aggregate in aqueous media driven by hydrophobic interactions and π–π stacking, causing fluorescence quenching and limiting cellular uptake and intracellular accumulation. To optimize the function of these dyes, Zhang and co-workers designed a supramolecular porphyrin compound by wrapping porphyrin in CB[10] with a larger cavity for near-infrared cell imaging (Fig. 3).23 Relative to the porphyrin monomer, this supramolecular structure reduces its susceptibility to oxidation and exhibits lower cytotoxicity, brighter fluorescence signal, and better photostability. This strategy is expected to solve the problems of other fluorescent dyes and expand their versatility. However, since common fluorophores are limited by the phenomenon of aggregation-induced quenching, Prof. Tang developed organic fluorophores with aggregation-induced emission (AIE) properties providing a novel platform to construct nano-aggregates for the detection of specific substances and imaging.77 Thus, Zhao et al. constructed two-dimensional supramolecular nanostructures with distinct AIE effects by the host–guest interaction of pyridinium salt-modified triphenylamine (TPE) derivatives and CB[8], which was successfully applied to the visualization of intracellular DNA with a high signal-to-noise ratio (Fig. 4a).78 Notably, borrowing triphenylamine derivatives with the AIE effect as a guest, they obtained two complexes of CB[n]-mediated [1 + 3] and [2 + 3] self-assembly (Fig. 4b).79 Interestingly, 1_CB[7] can light up both the nucleus and cytoplasm, while 1_CB[8] mainly stays in the cytoplasm.
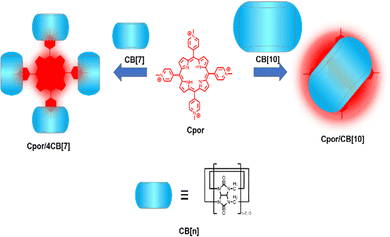 |
| Fig. 3 Schematic representation of the fabrication of supramolecular porphyrins. | |
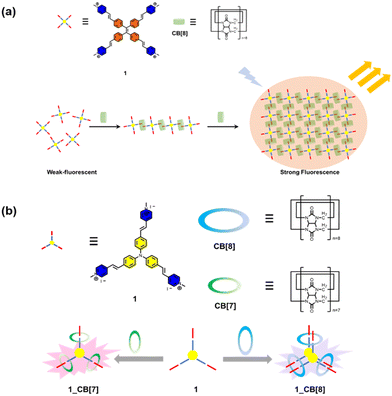 |
| Fig. 4 (a) Chemical structures of the guest molecule 1 and the host CB[8] and schematic representation of the formation of a 2D supramolecular structure in a head-to-tail self-assembled mode and stepwise fluorescence enhancement. (b) Schematic representation structures of guest molecule 1 and CBs and photographs of the complex solution. | |
To embody more possibilities of the AIE group, Huang et al. designed two four-armed TPE derivatives of electron-rich naphthalene (TPE-NP) and electron-deficient paraquat (TPE-PQ),80 which self-assembles into one-dimensional nanostructures under charge transfer (CT) action. Negatively charged bifunctional water-soluble pillar[6]arene was promoted to cap the PQ through host–guest interactions and reduce its biotoxicity (Fig. 5). The slightly acidic environment of cancer cells can trigger the dissociation of host–guest molecules, leading to the formation of CT complexes between naked TPE-PQ and TPE-NPs, which enables AIE-enhanced fluorescence imaging of cancer cells. However, the safety evaluation of drugs needs to be further investigated in vivo. Afterward, Ding et al. reported host–guest complexed supramolecular nanodots between CB[5] and AIEgens.47 Both intramolecular motion and ISC transitions (ROS generation) of AIEgens are greatly restricted due to their host–guest interactions, resulting in the formation of ultrabright S-AIE dots in water (Fig. 6a). Such supramolecular AIE dots with low toxicity can serve as excellent fluorescent bio-probes for ultrasensitive fluorescence image-guided cancer surgery.
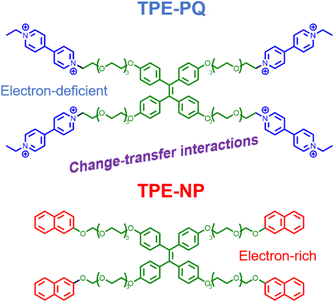 |
| Fig. 5 Chemical structures of TPE-PQ and TPE-NP. | |
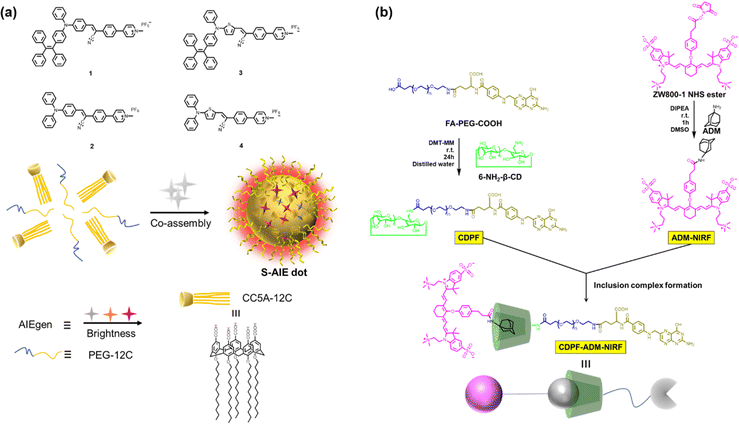 |
| Fig. 6 (a) Chemical structures of the AIEgens and CC5A-12C; and the illustration of the co-assembly. (b) Chemical structures and the inclusion complex formation of the host and guest. | |
Precisely targeted tumor imaging and specific drug delivery are the prerequisites for effective tumor treatment and reduction of toxic and side effects.81 Based on this, Hyun and co-workers reported a self-assembled supramolecular structure for specific imaging of breast cancer.82 β-CD, Polyethylene glycol (PEG), and folic acid (FA) were sequentially coupled to form a complex CDPF, which combined adamantane (Ad) with a near-infrared fluorophore (ADM-NIRF) to construct supramolecular compounds for realizing precise tumor imaging (Fig. 6b). FA could strongly bind to the overexpressed folate receptor in the MCF-7 cell membrane. Therefore, this study provides a smart platform for the preparation of simple and efficient nanocarriers for cancer imaging and therapy. Besides, accurate imaging of dynamic changes in the tumor microenvironment (TME) enables early tumor detection and non-invasive assessment, thereby facilitating personalized therapy and improving patient survival.83 Thus, utilizing the special properties of TME to formulate fluorescent probes activated by endogenous substances has become the mainstream method for detecting tumor cells.84 Indeed, Zhang and co-workers85 developed a two-photon excited, H2O2-activated nanoprobe (TPFN) using adamantane (Ad)-fluorophore conjugates as guests and a β-CD polymer as the host (Fig. 7). Notably, this supramolecule can significantly amplify the fluorescence intensity of the guest molecule (21-fold increase), resulting in a detection limit of 0.127 µM for H2O2, which is much lower than that of the free guest molecule (11.98 µM) and a 94-fold increase in sensitivity. TPFN provides ratiometric two-photon imaging of H2O2 in live cells and extra-liver tissue. Thus, with this simple supramolecular self-assembly strategy, TPFN can serve as a powerful nanoplatform for ratiometric imaging of various species.
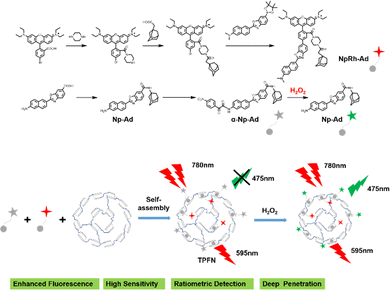 |
| Fig. 7 The synthetic route and the self-assembly illustration of the two-photon excited fluorescent nanoprobe. | |
Certainly, hypoxia is a common characteristic of most solid tumors, and visualization of hypoxic areas contributes to better detection and imaging of tumors. Thus, Gao et al. prepared a simple and efficient bio-oxygen sensor of a β-CD encapsulated hydrophobic Ir(III) complex and Cy7 as a ratiometric optical probe.86 This probe can be used to non-invasively map the hypoxic microenvironment of solid tumors and quantitatively measure oxygen levels in vivo. Then, Guo and co-workers reported a supramolecular host–guest approach based on the complexation of hypoxia-responsive macrocycles (carboxyl-modified azocarboxy[4]arene, CAC4A) with Rho123).87 The fluorescence of Rho123 was quenched by binding with CAC4A, but under hypoxic conditions, the azo group of CAC4A was activated in response leading to a reduction in the selectivity of the macrocycles for the fluorophore and consequent recovery of fluorescence (Fig. 8a). This work not only opens up new avenues for hypoxia imaging but also explores new biomedical applications of azo macrocycles. Besides, abnormal levels of biothiols are thought to be associated with many human diseases. Therefore, the development of methods to real-time monitor their concentrations in living cells is imperative for a deeper understanding of related pathophysiological processes. Liu et al. reported a host–guest interaction between coumarin modified β-CD (rCP-β-CD) and Ad-modified cRGD to construct a reversible tumor-targeting fluorescent probe (Fig. 8b).88 The supramolecular assemblies with good cancer cell permeability can be used for reversible, rapid, and efficient real-time monitoring of biothiols in cancer cells.
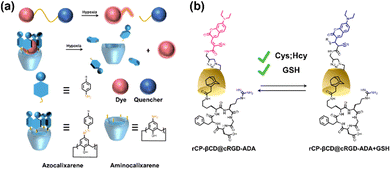 |
| Fig. 8 (a) Schematic illustration of the design principles of the conventional covalent and the proposed noncovalent strategies for creating hypoxia-responsive systems. (b) Construction of rCP-βCD@cRGD-ADA and the reversible Michael addition reactions between rCP-βCD@cRGD-ADA and biothiols. | |
Recently, Kim and co-workers constructed an ultra-stable host–guest complex, enabling direct visualization of two distinct organelles and their subsequent autophagy-related fusion in living cells (Fig. 9).89 CB[7]-Cy3 and AdA-Cy5 were deemed as a FRET supramolecular donor and acceptor. They accumulated in lysosomes and mitochondria, respectively, lighting up different organelles. By the transformation of the FRET signal, the fusion process of organelles can be dynamically observed to further study the degree of autophagy. In principle, this ultra-stable binding can also be applied to other organelles and dyes to visualize different physiological processes, such as the endoplasmic reticulum and lipid droplets in living cells. In the same year, Liu et al. constructed fluorescence-enhanced supramolecular aggregates for lysosomal-targeted imaging by a two-stage assembly of CB[8] and amphiphilic calixarene (Fig. 10).90 4,4′-Anthracene-9,10-diylbis(ethene-2,1-diyl))bis(1-ethylpyridin-1-ium) bromide (ENDT), an organic dye with weak fluorescence, can assemble with CB[8] to form nanorods, and then interact with sulfonatocalix-[4]arene (SC4AD) to form nanoparticles with strong fluorescence. Co-staining experiments with commercial reagent LysoTracker Blue showed that these assembled nanoparticles with near-infrared emission could be used for targeted imaging of lysosomes. Such a dual macrocyclic supramolecular assembly strategy provides a unique idea for the design and construction of imaging reagents in vivo. Compared to the single-modality fluorescence molecular imaging described earlier, multimodal imaging has become an important imaging method in the medical field, which provides important data support for precision medicine.91 Among them, magnetic resonance imaging (MRI) combined with fluorescence imaging has attracted more and more attention due to its ultra-high spatial resolution and sensitivity.92 However, the clinical application of the most common Gd-based contrast agents (CAs) has been hindered by safety concerns such as biocompatibility.93 Notably, the assembly of small molecules and hydrophilic macrocycles seem to solve the above problems.94 Lu et al. reported a well-targeted cyclodextrin nano-bimodal contrast agent cRGD-POSS-βCD(DOTA-Gd)-Cy5 using multiple β-CD-adsorbed POSS (polyhedral oligomeric silane-siloxane) nanospheres as host molecules, and an Ad-modified macrocyclic Gd(III) contrast agent, cRGD targeting ligand, and fluorescent probe Cy5 as guest molecules (Fig. 11).95 The effectiveness of this paradigm for dual-modality molecular imaging was demonstrated in a mouse 4T1 breast cancer model, as well as showing its potential for precise localization of cancer. Recently, Li et al. constructed an AIE effect and MRI functional bimodal supramolecular polymer P4 by host–guest assembly between β-CD and TPE-modified copolymers and Ad-modified Gd-based enantiomers (Fig. 12).96 Notably, Gd(III) in the polymer can be metabolised rapidly from the body, thereby reducing biological toxicity. The amphiphilic polymer can form vesicles in aqueous solution, thereby enhancing the AIE and MR effects. This work provides a new idea of combining AIE properties, magnetic relaxation, and responsiveness to design multimodal probes for future clinical diagnostic applications. In summary, exploiting the host–guest assembly between macrocyclic hosts and fluorophores can significantly improve the performance of bioimaging reagents, as well as being an effective way to design supramolecular fluorescence imaging systems.
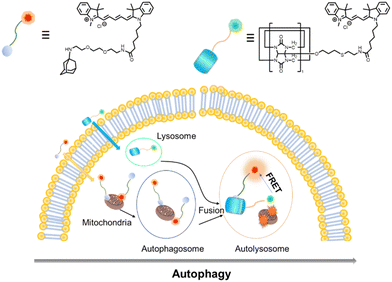 |
| Fig. 9 Chemical structures of CB[7]-Cy3 and AdA-Cy5 and the emergence of FRET after the fusion of two different organelles through the process of autophagy. | |
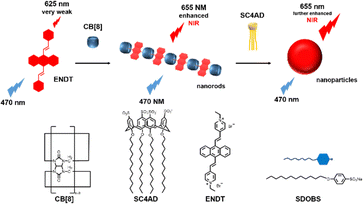 |
| Fig. 10 Illustration of the process of near-infrared fluorescence supramolecular assembly and associated supramolecular aggregation. | |
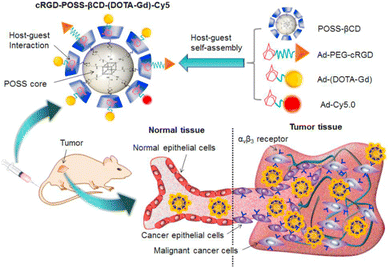 |
| Fig. 11 A targeted host–guest MRI contrast agent, cRGD-POSS-βCD-(DOTA-Gd)-Cy5, self-assembled from POSS- β CD, Ad-PEG-cRGD, Ad-(DOTA-Gd) and Ad-Cy5 by host–guest interactions. Reproduced with permission from ref. 95. Copyright 2016, Elsevier Ltd. | |
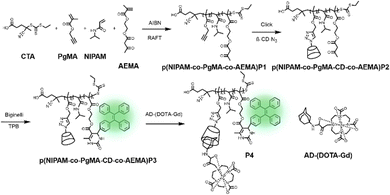 |
| Fig. 12 Synthesis of p(NIPAM-co-PgMA-CD-co-AEMA-TPE) and the chelation with Ad-(DOTA-Gd), and self-assembly and disassembly of P4. | |
3.2. Therapy
To achieve good tumor inhibition and minimal side effects of drugs in treatment, various emerging non-invasive therapeutic strategies have been developed in recent years, including photothermal therapy and photodynamic therapy.
3.2.1. Photodynamic therapy (PDT).
PDT refers to a combination of spatiotemporal light irradiation with photosensitizers (PSs) to generate singlet oxygen (1O2) or other types of ROS, which can be used to kill cancer cells, bacteria or fungi.97 Light irradiation's non-invasiveness and spatiotemporal selectivity enable PDT to be well-controllable compared with other traditional treatment options.98 However, the always-on state and low metabolic rate of traditional PSs may cause adverse damage after PDT therapy.99 Therefore, how to rapidly inactivate PSs after PDT treatment is of great significance in both scientific research and clinical trials. In order to reduce the dark toxicity of PSs, Zhang et al. proposed a supramolecular strategy for regulating the activity of PS by cationic BODIPY derivatives and CB[7] assembly to construct a novel system with enhanced reactive ROS generation efficiency and accelerated auto-degradation (Fig. 13a).100 Compared with conventional PSs, supramolecular PSs exhibit better photodynamic therapeutic efficiency and higher biocompatibility and can be degraded by self-generated ROS. Once treatment ends, its activity is lost, which can reduce the side effects of PDT without sacrificing treatment efficiency. This work provides a new strategy for designing smart PDT to further improve the safety of the therapy. Although many PSs have efficient singlet oxygen conversion capacity, their poor water solubility and aggregation-induced quenching of photodynamic activity hinder their biological applications in PDT.101 Therefore, supramolecular chemistry technology stands out in photodynamic tumor therapy as a new method to solve such problems. Pei et al. designed a supramolecular PS system (WP6-MB) via host–guest interactions between the water-soluble WP6 and methylene blue (MB), which can achieve durable PDT (Fig. 13b).102 Without further modification, the complexation of WP5 and MB can significantly reduce the dark toxicity of MB, effectively overcome photobleaching, and prolong the time for MB to generate ROS under light irradiation, thereby further enhancing the efficacy of PDT.
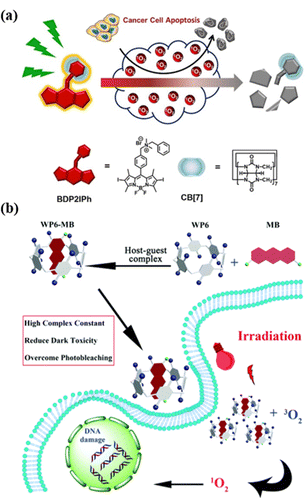 |
| Fig. 13 (a) Representation of a self-degradable photosensitizer for photodynamic therapy. (b) Schematic of the construction of the supramolecular photo-sensitizer system based on the host–guest complexation between WP6 and MB for PDT. Reproduced with permission from ref. 100 and 102. Copyright 2021, 2018 Wiley-VCH, The Royal Society of Chemistry. | |
Similarly, Zhang et al. reported a photosensitizer of porphyrin-conjugated Ad with cyclodextrin to form a linear supramolecular polymer with an alternating porphyrin/cyclodextrin structure in water (Fig. 14).103 This linear alternating structure can not only prevent the aggregation of PSs through steric hindrance, but also enhance the increase of singlet oxygen and improve the water solubility of PSs. Therefore, this linear molecule can also overcome the major drawbacks of other conventional photosensitizers. Applying the same principle, Huang et al. designed and synthesized a supramolecular ROS switch (AIEgenG ⊃ WP5).104 The complexes produced negligible fluorescence and ROS in a neutral environment. However, when the pH value in the environment decreases, benefiting from the sensitivity of WP5, the fluorescence was turned on and ROS generated (Fig. 15). The switch can selectively light up the lysosome, suggesting that it is stable after endocytosis and could be a precision medicine for cancer treatment. Besides, Tang and co-workers constructed low-darkness supramolecular nano-assemblies by host–guest binding of positively charged pyridine-functionalized TPE (TPE-PHO) with p-sulfonatocalix[4]arene (SC4A).105 When TPE-PHO was competitively displaced from the cavity of calixarene by 4,4-benzidine dihydrochloride at the tumor site, its dark cytotoxicity and photoactivity in tumor tissues were restored. SC4A ⊃ TPE-PHO kills cancer cells by highly specific translocation from the cytoplasm to mitochondria during a cascade of supramolecular replacement, successfully enabling in vivo tumor imaging and therapy (Fig. 16). However, short-wavelength fluorescence emission is not conducive to real-time imaging of deep tissues in vivo. Furthermore, Sun et al.106 rationally designed supramolecular photosensitizers (1 ⊃ 2 NPs) via the host–guest assembly of AIEgen and pillar[5]arene modified Pt(II) metallacycle (Fig. 17). The NPs with promising single-state oxygen quantum yields (ΦΔ = 0.97) improved the efficiency of photodynamic inactivation (PDI). Notably, the nanoparticles exhibited in situ fluorescence tracking and precise image-guided therapeutic effects on S. aureus. The combination of metal-ring and host–guest chemistry could provide a rational design platform for the biomedical development of potent photosensitizers. Furthermore, apart from supramolecular photosensitizers based on host–guest assembly, researchers have also explored other supramolecular strategies to enhance the activity of photosensitizers and improve their therapeutic effect.107–109
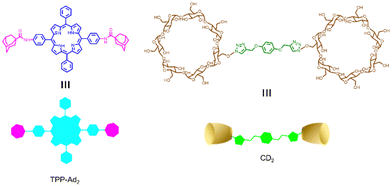 |
| Fig. 14 Chemical structures of the adamantyl-modified tetraphenyl porphyrin (TPP-Ad2) and the cyclodextrin dimer (CD2). | |
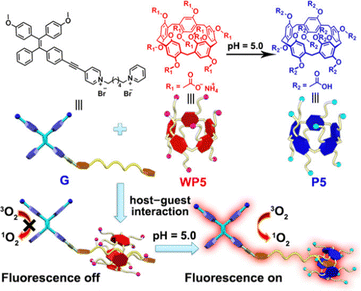 |
| Fig. 15 Chemical structures and cartoon representations of WP5, P5 and G. Adapted with permission from ref. 104. Copyright 2020 Wiley-VCH. | |
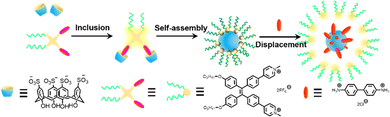 |
| Fig. 16 Schematic diagram of substitution activated phototheranostics, and the proposed mechanism of cytoplasm-to-mitochondrial translocation of TPE-PHO ⊂ SC4A + BZD. | |
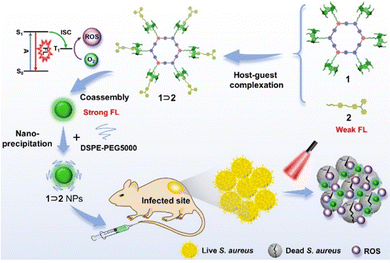 |
| Fig. 17 Schematic diagram of the assembly of metal ring supramolecular photosensitizers and in vivo fluorescence tracking and image-guided efficient photodynamic inactivation of Staphylococcus aureus infection sites. Reproduced with permission from ref. 106. Copyright 2022 Wiley-VCH. | |
3.2.2. Photothermal therapy (PTT).
PTT relies on photothermal agents (PTAs) to convert the absorbed light energy into heat, which increases the local temperature of the tumor, and finally kills it.110 Based on the poor water solubility and photostability of small molecule PTA, supramolecular chemistry provides a new way to improve the photophysical and photochemical properties of organic small molecule dyes.111 Therefore, Zhang et al. reported a new strategy for NIR-II photothermal conversion and photothermal therapy by preparing supramolecular radical dimers.112Fig. 18 shows that the N,N′-dimethylated pyridinium thiazolo[5,4-d]thiazole radical cation (MPT˙+) and CB[8] can self-assemble into a 2
:
1 host–guest complex. This complex exhibits efficient photothermal conversion and excellent stability in the NIR-II biological window as well as exhibits a high inhibition rate against HepG2 cells. This study is expected to provide a new strategy for NIR-II PTT, which has broad application prospects in the fields of luminescent materials, optoelectronic materials, and biosensing. Recently, Guo and co-workers113 used sulfonated azocalix[4]arene (SAC4A) as a hypoxia-responsive macrocyclic host, and further ground it with IR780 to prepare a novel stimuli-responsive supramolecular PTT formulation IR780@SAC4A. After reaching the tumor site, the azo linker of SAC4A is reduced by reductase to form NH2–C4A, resulting in the dissociation of IR780 from the macrocyclic cavity (Fig. 19).
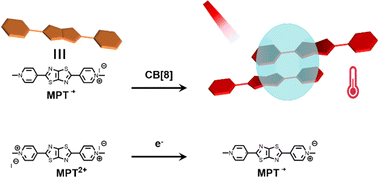 |
| Fig. 18 Illustration of the host–guest structure and its NIR-II photothermal conversion. | |
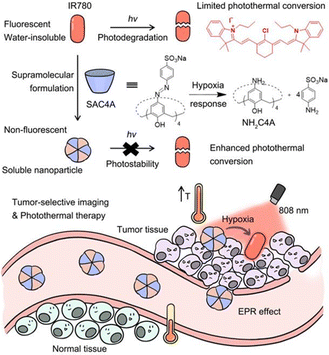 |
| Fig. 19 Schematic illustration of supramolecular PTA formed by host–guest complexation between SAC4A and IR780 and the effect of photothermal therapy on cancer cells. Reproduced with permission from ref. 113. Copyright 2022 Ivy-Spring. | |
With the emergence of various drug carriers, Liu and colleagues constructed two-dimensional host–guest supramolecular nanoassemblies114 with β-CD grafted graphene oxide(GOCD) as the host and tumor-targeting protein-modified peptides and azobenzene as the guests (Fig. 20). The nano-assemblies not only specifically targeted and disrupted tumor cell mitochondria, leading to cell cycle arrest, but also had excellent NIR photothermal efficiency and could significantly inhibit tumour growth. In addition, Wang et al. developed inorganic nanoclusters based on host–guest assembly for enhanced CT imaging and photothermal therapy with highly specific local activation.115 Excitably, this supramolecular strategy can adaptively establish plentiful supramolecular PTAs via different hosts and fluorochromes, providing a general approach for the formulation of smart PTT systems.
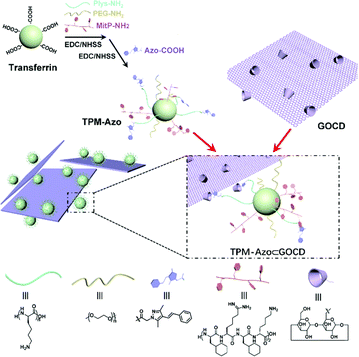 |
| Fig. 20 Synthesis of the TPM-Azo guest, the GOCD host, and the TPM-Azo ⊂ GOCD host–guest supramolecular nanoassemblies. Reproduced with permission from ref. 114. Copyright 2019 The Royal Society of Chemistry. | |
3.2.3. Combination therapy.
At present, monotherapy has many drawbacks such as non-specific effects, drug resistance, and toxic side effects, which hinder their anti-cancer effect and often fail to achieve the desired therapeutic effect.116 However, it is promising and feasible to combine two or more therapeutic modalities to improve tumor suppressive ability.117 Several studies have confirmed that combined therapy can significantly enhance its anticancer efficacy.118,119 For example, Tang and co-workers utilized the host–guest interaction of the photosensitizer TTPY-Py with AIE and Au nanorods modified with CP5 to construct a hybrid therapeutic nanosystem TTPY-Py ⊂ CP5@AuNR (Fig. 21).120 Successful modification of the CP5 macrocycle on the surface of AuNRs can eliminate its cytotoxicity and become a nanocarrier for photosensitizers. This probe combined their respective advantages of efficient reactive oxygen species generation, photoacoustic imaging, and photothermal conversion, which is beneficial for fluorescence-photoacoustic imaging guidance and synergistic PDT-PTT. This study provides a new design idea for the use of supramolecular nanomaterials in multimodal integrative therapy.
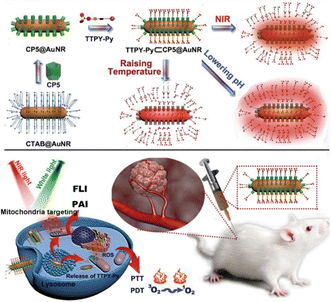 |
| Fig. 21 Schematic illustration for the preparation of the nanomaterials with supramolecular assembly of CP5 and TTPY-Py, and their application in synergistic photodynamic-photothermal therapy with real-time tumor-NIR&PA imaging. Reproduced with permission from ref. 120. Copyright 2021 Wiley-VCH. | |
In anticancer therapy, chemotherapy is an important and widely used clinical antitumor strategy. However, due to the clinical deficiencies of traditional chemotherapy, a combination with other treatments has received considerable attention.121 Zhang et al. obtained a versatile nanoplatform (TPZ@MCMSN-Gd3+) via layer-by-layer assembly of tirapazamine (TPZ)-supported mesoporous silica nanoparticles and the host–guest interaction of β-CD modification with porphyrin–paramagnetic Gd chelates.122 When specifically taken up by tumor cells overexpressing the CD44 receptor, the nanoparticles react with hyaluronidase (HAase) to trigger the release of the therapeutic agent (Fig. 22). In particular, accelerated oxygen depletion by PDT ensures that TPZ (a bioreductive prodrug that is cytotoxic to hypoxic cells) can be effectively released at the tumor site. Similarly, Mao et al.123 designed and fabricated a promising therapeutic nanosystem VK3-CPT@Ru-CD (Fig. 23) by host–guest-driven self-assembly between fluorescent Ad-functionalized Ru(II) complexes and βCD-modified thioketal and alkyl linker, and co-encapsulating the anticancer drug camptothecin (CPT) and vitamin K3 (VK3). With the inherent ROS and overexpression of NQO1 in cancer cells, disruption of the sulfhydryl ketone linker in the drug delivery system releases more VK3, creating a regenerative feedback process of ROS amplification and nanoparticle collapse, which leads to the effective release of CPT. More interestingly, the menadione structure of the encapsulated VK3 can effectively quench the intrinsic fluorescence of Ru-CD. Thus, the fluorescence brightening phenomenon accompanying ROS-triggered drug release can be observed for real-time tracking of drug release in vitro and in vivo. Notably. Stang et al. reported the formation of a bifunctional system M ⊃ OEP by utilizing well-defined cavities of discrete organoplatinum metal containers (M) to encapsulate the photosensitizer octaethylporphyrin (OEP).124 A host–guest complex was further encapsulated by amphiphilic copolymers, promoting the formation of MNPs with the ability to co-deliver chemotherapeutic drugs and photosensitizers (Fig. 24). The combined therapy exhibited superior antitumor properties in drug-resistant tumor models. Light-triggered ROS generation and cascaded prodrug activation have attracted great attention.125 Zhao et al. successfully developed a new strategy for optimizing the ratio of PS and prodrug based on host–guest interactions.126 A diblock copolymer (PEG-PGA-CD) was composed of PEG, β-CD-modified poly-L-glutamic acid (PGA), and Ad-modified aza-BODIPY and paclitaxel (PTX) (Fig. 25). The combined PDT and chemotherapy provided by Ad-PTX (60%)-BODIPY (40%)-PNs have been effectively maximized. Therefore, intensive research can continue to improve nanosystems to track the distribution of key components in real-time in vivo.
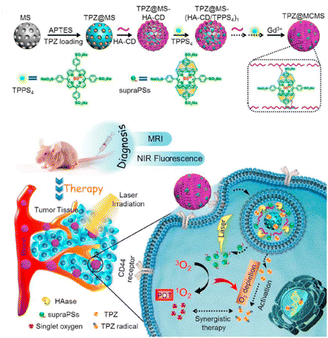 |
| Fig. 22 HA-CD and TPPS4 were assembled layer-by-layer on top of TPZ-loaded MSNs, a diagnostic nanoplatform TPZ@MCMSN-Gd3+ was fabricated by entraining TPZ in the mesoporous core and supraPSs in the multilayer, and its tumor targeting schematic. Adapted with permission from ref. 122. Copyright 2017 Elsevier. | |
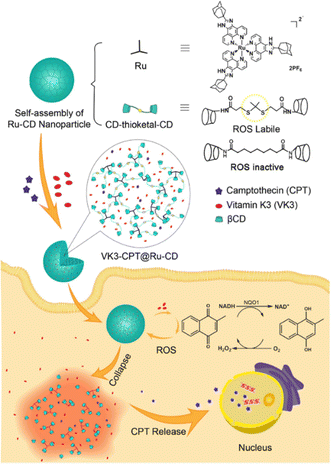 |
| Fig. 23 Schematic illustration of the formation process of nanoparticle VK3-CPT@Ru-CD and VK3-mediated cancer-specific ROS amplification and fluorescence visualization of drug release. Adapted with permission from ref. 123. Copyright 2018 Elsevier. | |
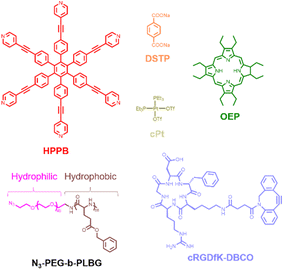 |
| Fig. 24 Chemical structures of different intermediates and cRGDfK-DBCO. | |
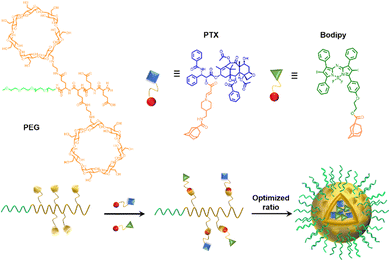 |
| Fig. 25 Schematic illustration of the structures of the polymer, PS, and prodrug, the self-assembly process of the nanoparticles with the optimized ratio. | |
With in-depth research, Wang and co-workers127 prepared a supramolecular system with modular functional nano-aggregates (Fig. 26) by loading drug molecules such as oxaliplatin and baranothraquinone (AQ4N) through the cavity structure of TPE derivative photosensitizer-modified CB[7]. These obtained nanoaggregates specifically light up lysosomes and exhibit enhanced cytotoxicity with photodynamic combination chemotherapy against cancer cells in vitro. Some non-negligible progress has also been made in the combined treatment of photothermal therapy and chemotherapy in phototherapy. Based on different embedding methods, Zhao et al.128 obtained self-assembled supramolecular nanoparticles through a specific host–guest interaction between hydrophilic β-CD functionalized hyaluronic acid and the Ad-linked CPT/naphthalimide dye conjugate, and further encapsulated them with IR825 dye for photothermal and chemotherapy (Fig. 27). In the reducing environment in cancer cells, the disulfide bond is broken, which can release CPT and IR825. The nanoparticles effectively destroyed cancer cells and tumors under laser irradiation. On balance, employing supramolecular chemistry with host–guest interactions to integrally address the problems of traditional therapeutic reagents is a valuable research direction in the biomedical field. Besides, multifunctional novel therapeutic platforms are urgently needed. Considering the advantages of host–guest chemistry, the innovatively designed multifunctional supramolecular systems will help to improve the current therapeutic technology capabilities.
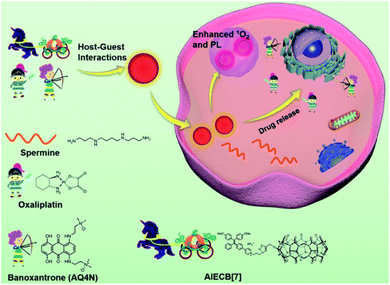 |
| Fig. 26 Schematic illustration of the design of AIECB[7] and its application for imaging-guided, synergistic PDT and supramolecular chemotherapy. Adapted with permission from ref. 127. Copyright 2021 the Royal Society of Chemistry. | |
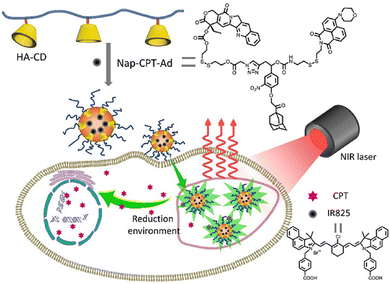 |
| Fig. 27 Schematic illustration of IR825 dye loaded reduction-responsive and fluorescence-enhanced supramolecular nanoparticles for combinational photothermal-chemotherapy of cancer. | |
3.3. Detecting biomarkers in biofluids
The realization of specific identification and visual detection of endogenous substances in biological fluids plays an irreplaceable role in the early diagnosis of human diseases.129 To date, analytical methods using some powerful large-scale instruments, such as high-performance liquid chromatography-mass spectrometry (HPLC-MS), gas chromatography-mass spectrometry (GC-MS), and magnetic resonance imaging (MRI), are commonly used clinical methods for the analysis of biological fluids.130 However, these methods require complicated procedures and handling, which are not conducive to routine diagnostics. So far, molecular recognition-based diagnostics have yielded promising results in sensing technologies, while supramolecular chemistry has also received increasing attention.131 Based on this, the innovative synergistic analysis of synthetic molecular probes and host–guest self-assembled supramolecular sensors provides great potential to develop low-cost and fast-response biosensors suitable for biological fluids (blood, urine, etc.). Therefore, in the follow-up, we mainly introduce a supramolecular sensing system for the detection of biomarkers in biological fluids through supramolecular host–guest interactions.
We have previously mentioned that Biedermann and co-workers have published a comprehensive review of supramolecular biosensors for the interested reader.33 So, we will briefly introduce their significant contributions to the detection of biological fluids in recent years. We all know that many biological small molecules and proteins in biofluids have the ability to bind competitively to the host, thereby causing great interference. Meanwhile, the strong absorption and emission of blood over a wide wavelength range pose additional difficulties for detection. Fortunately, Biedermann et al. provided a proof-of-principle for the in situ and quantitative detection of the Alzheimer's disease drug memantine in serum via indicator-displacement assays (IDAs, Fig. 28a).132 However, this is only possible if the affinity of the interest analyte is higher than that of the interfering substance and the reporter dye has a narrow binding affinity range. Subsequently, in order to search for CB[8] indicator dyes with ultra-high affinity, a new class of [2.2]paracyclophane-based dyes (MPCP) was designed and synthesized with one of the highest host–guest affinity (Ka > 1012 M−1 in water, Fig. 28b) with known supramolecular host–guest chemistry and provides a large Stokes shift (up to 200 nm). A new IDA is then applied to detect memantine in the physiologically relevant sub-to-low micromolar range in serum. Specifically, this is the first working example of a cucurbituril-based sensing assay in serum.
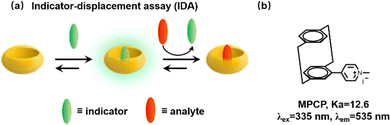 |
| Fig. 28 (a) Schematic representation of an indicator-displacement assay (IDA). (b) The chemical structure of paracyclophane-based dyes (MPCP). | |
Following their further investigations, due to the competitive (or copper ion) cation binding to the carbonyl edge of CB[n] entry, non-covalent interactions between the host and the guest are strongly regulated by salt.133 Thus, two novel single-molecule chemosensors with hydrophilic and bendable linkages were synthesized. In particular, they reported their interaction with biologically relevant molecules based on the study of CB[7] (Fig. 29).134 The conjugates exhibit excellent electrophoresis in both dilution and salt, maintaining chemical sensor functionality even at 106 times more than NaCl. Significantly, a novel CB[7]-dye conjugate was reported for differential analyte sensing via the salt-adaptive behaviour of supramolecular host–guest complexes. Fourteen bioorganic assays were successfully distinguished by simple stepwise addition of salt (Fig. 30).135 This work presents a non-selective binding of a salt-adapted melon-urinary system for sensing biological fluids such as urine, saliva, and serum. Therefore, compared to conventional clinical methods, a supramolecular fluorescent system based on host–guest assembly is a new reliable method for detecting markers in biological fluids.
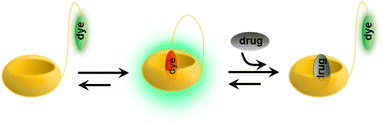 |
| Fig. 29 Schematic representation of analyte-induced conformational changes of CB[7]-dye conjugates that can be detected by emission spectroscopy. | |
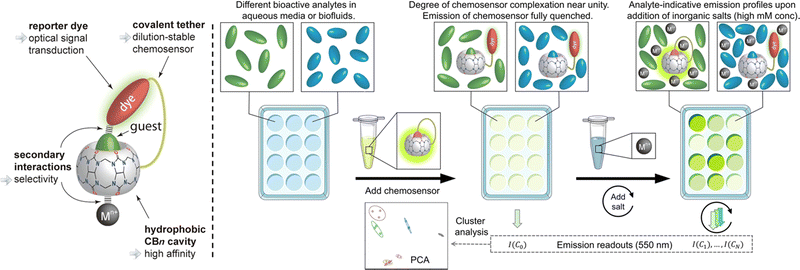 |
| Fig. 30 Schematic representation of the unimolecular CBn-based chemosensor that undergoes salt-induced adaptation and that can be used for the distinction of bioorganic analyte in aqueous media and biofluids. Reproduced with permission from ref. 135. Copyright 2022 American Chemical Society. | |
4. Summary and outlook
In recent years, fluorescent sensors based on macrocyclic molecules have received great attention in various fields of chemistry, biology and clinical medicine due to their excellent photophysical properties and simplicity of synthesis, while researchers have devoted great efforts to biomedical applications of supramolecular systems based on host–guest construction. Therefore, focusing on the interaction between macrocyclic molecules and chromophores, this review discussed the construction of macrocyclic-fluorophore systems based on host–guest assembly and their recent progress in biomedical applications, mainly involving the detection of biofluids, bioimaging, photodynamic therapy, photothermal therapy and combination therapy.
There is no doubt that these studies have contributed to the development of novel supramolecular fluorescent systems.136–139 The biomedical application of macrocyclic-chromophore complexes starts with facile host–guest assemblies and their modification of functional group properties. On the one hand, the assembly optimizes the physicochemical properties of the fluorophore (e.g., stability, water solubility, brightness and biocompatibility) and on the other hand it can improve the activity of the therapeutic reagents.140,141 In addition, host–guest assembly chemistry has also been used to improve the performance of chemiluminescent dyes/probes and then for detection and imaging, e.g., the adamantane group inherent in adamantane-1,2-dioxane chemiluminescent probes can act as a well-bound guest molecule for assembly with cyclodextrins or cucurbit[n]uril.142–144 Despite the large number of scientific studies published in recent years on the biomedical applications of supramolecular systems, the clinical application of supramolecular systems still faces many practical challenges. In particular, the biosafety of supramolecular systems in vivo, including the biodegradability, metabolism and long-term toxic effects of various macrocycles and their derivatives, needs to be further investigated.
As the deep understanding of the process of tumour development progresses, novel tumor therapy methods such as molecular targeted therapy, gene therapy and immunotherapy are emerging. In our view, the combination of supramolecular therapy systems with emerging therapeutic approaches is expected to gain great advances in medical applications in the future. Meanwhile, constructing longer wavelength supramolecular fluorescent systems and supramolecular materials with multimodal imaging capabilities can better promote the development of supramolecular fluorescence chemistry.
Conflicts of interest
There are no conflicts to declare.
Acknowledgements
This work was supported by the National Natural Science Foundation of China (22074036, 21877029).
References
- J.-M. Lehn, Angew. Chem., Int. Ed. Engl., 1988, 27, 89–112 CrossRef.
- J. M. Lehn, Chem. Soc. Rev., 2017, 46, 2378–2379 RSC.
- K. Liu, Y. Kang, Z. Wang and X. Zhang, Adv. Mater., 2013, 25, 5530–5548 CrossRef CAS PubMed.
- Z. Zhang, Y. Luo, J. Chen, S. Dong, Y. Yu, Z. Ma and F. Huang, Angew. Chem., Int. Ed., 2011, 50, 1397–1401 CrossRef CAS PubMed.
- Z. Zhou, X. Yan, T. R. Cook, M. L. Saha and P. J. Stang, J. Am. Chem. Soc., 2016, 138, 806–809 CrossRef CAS PubMed.
- R. N. Dsouza, U. Pischel and W. M. Nau, Chem. Rev., 2011, 111, 7941–7980 CrossRef CAS PubMed.
- X. Ma and Y. Zhao, Chem. Rev., 2015, 115, 7794–7839 CrossRef CAS PubMed.
- D. Li, Z. Feng, Y. Han, C. Chen, Q. W. Zhang and Y. Tian, Adv. Sci., 2022, 9, e2104790 CrossRef PubMed.
- D. Li, Y. Han, Y. Jiang, G. Jiang, H. Sun, Z. Sun, Q. W. Zhang and Y. Tian, ACS Appl. Mater. Interfaces, 2022, 14, 1807–1816 CrossRef CAS PubMed.
- B. Zheng, F. Wang, S. Dong and F. Huang, Chem. Soc. Rev., 2012, 41, 1621–1636 RSC.
- Z. Liu and Y. Liu, Chem. Soc. Rev., 2022, 51, 4786–4827 RSC.
- G. Crini, Chem. Rev., 2014, 114, 10940–10975 CrossRef CAS PubMed.
- D. S. Guo and Y. Liu, Chem. Soc. Rev., 2012, 41, 5907–5921 RSC.
- Y. C. Pan, X. Y. Hu and D. S. Guo, Angew. Chem., Int. Ed., 2021, 60, 2768–2794 CrossRef CAS PubMed.
- S. J. Barrow, S. Kasera, M. J. Rowland, J. del Barrio and O. A. Scherman, Chem. Rev., 2015, 115, 12320–12406 CrossRef CAS PubMed.
- J. Lagona, P. Mukhopadhyay, S. Chakrabarti and L. Isaacs, Angew. Chem., Int. Ed., 2005, 44, 4844–4870 CrossRef CAS PubMed.
- T. Ogoshi, T. A. Yamagishi and Y. Nakamoto, Chem. Rev., 2016, 116, 7937–8002 CrossRef CAS PubMed.
- Y. Mei, Q. W. Zhang, Q. Gu, Z. Liu, X. He and Y. Tian, J. Am. Chem. Soc., 2022, 144, 2351–2359 CrossRef CAS PubMed.
- Y. Ding, C. Wang, B. Lu and Y. Yao, Front. Chem., 2021, 9, 775436 CrossRef CAS PubMed.
- Y. F. Ding, C. H. T. Kwong, S. Li, Y. T. Pan, J. Wei, L. H. Wang, G. S. P. Mok and R. Wang, Biomater. Sci., 2021, 9, 3804–3813 RSC.
- M. Raynal, P. Ballester, A. Vidal-Ferran and P. W. van Leeuwen, Chem. Soc. Rev., 2014, 43, 1660–1733 RSC.
- K. L. Kim, G. Sung, J. Sim, J. Murray, M. Li, A. Lee, A. Shrinidhi, K. M. Park and K. Kim, Nat. Commun., 2018, 9, 1712–1723 CrossRef PubMed.
- H. Wang, Y. Yang, B. Yuan, X. L. Ni, J. F. Xu and X. Zhang, ACS Appl. Mater. Interfaces, 2021, 13, 2269–2276 CrossRef CAS PubMed.
- J. Gao, J. Li, W. C. Geng, F. Y. Chen, X. Duan, Z. Zheng, D. Ding and D. S. Guo, J. Am. Chem. Soc., 2018, 140, 4945–4953 CrossRef CAS PubMed.
- K. Yang, Z. Zhang, J. Du, W. Li and Z. Pei, Chem. Commun., 2020, 56, 5865–5876 RSC.
- L. You, D. Zha and E. V. Anslyn, Chem. Rev., 2015, 115, 7840–7892 CrossRef CAS PubMed.
- C. Hou, Z. Huang, Y. Fang and J. Liu, Org. Biomol. Chem., 2017, 15, 4272–4281 RSC.
- X. Zhou, P. Pathak and J. Jayawickramarajah, Chem. Commun., 2018, 54, 11668–11680 RSC.
- M. J. Webber and R. Langer, Chem. Soc. Rev., 2017, 46, 6600–6620 RSC.
- J. Zhou, G. Yu and F. Huang, Chem. Soc. Rev., 2017, 46, 7021–7053 RSC.
- L. Voorhaar and R. Hoogenboom, Chem. Soc. Rev., 2016, 45, 4013–4031 RSC.
- X. Yan, F. Wang, B. Zheng and F. Huang, Chem. Soc. Rev., 2012, 41, 6042–6065 RSC.
- J. Kramer, R. Kang, L. M. Grimm, L. De Cola, P. Picchetti and F. Biedermann, Chem. Rev., 2022, 122, 3459–3636 CrossRef PubMed.
- H. Nie, Z. Wei, X. L. Ni and Y. Liu, Chem. Rev., 2022, 122, 9032–9077 CrossRef CAS PubMed.
- Y. Liu, Z. Wang and X. Zhang, Chem. Soc. Rev., 2012, 41, 5922–5932 RSC.
- B. Zhang and R. Breslow, J. Am. Chem. Soc., 1993, 115, 9353–9354 CrossRef CAS.
- R. Kumar, A. Sharma, H. Singh, P. Suating, H. S. Kim, K. Sunwoo, I. Shim, B. C. Gibb and J. S. Kim, Chem. Rev., 2019, 119, 9657–9721 CrossRef CAS PubMed.
- D.-S. Guo and Y. Liu, Acc. Chem. Res., 2014, 47, 1925–1934 CrossRef CAS PubMed.
- W. Liu, S. K. Samanta, B. D. Smith and L. Isaacs, Chem. Soc. Rev., 2017, 46, 2391–2403 RSC.
- L. Cao, M. Sekutor, P. Y. Zavalij, K. Mlinaric-Majerski, R. Glaser and L. Isaacs, Angew. Chem., Int. Ed., 2014, 53, 988–993 CrossRef CAS PubMed.
- W. Feng, M. Jin, K. Yang, Y. Pei and Z. Pei, Chem. Commun., 2018, 54, 13626–13640 RSC.
- P. Wang, C. Zhang, H.-W. Liu, M. Xiong, S.-Y. Yin, Y. Yang, X.-X. Hu, X. Yin, X.-B. Zhang and W. Tan, Chem. Sci., 2017, 8, 8214–8220 RSC.
- H. Wang, K. F. Xue, Y. Yang, H. Hu, J. F. Xu and X. Zhang, J. Am. Chem. Soc., 2022, 144, 2360–2367 CrossRef CAS PubMed.
- J. B. Jiao, G. Z. Wang, X. L. Hu, Y. Zang, S. Maisonneuve, A. C. Sedgwick, J. L. Sessler, J. Xie, J. Li, X. P. He and H. Tian, J. Am. Chem. Soc., 2020, 142, 1925–1932 CrossRef CAS PubMed.
- T. B. Ren, W. Xu, Q. L. Zhang, X. X. Zhang, S. Y. Wen, H. B. Yi, L. Yuan and X. B. Zhang, Angew. Chem., Int. Ed., 2018, 57, 7473–7477 CrossRef CAS PubMed.
- T. Jiang, G. Qu, J. Wang, X. Ma and H. Tian, Chem. Sci., 2020, 11, 3531–3537 RSC.
- C. Chen, X. Ni, H. W. Tian, Q. Liu, D. S. Guo and D. Ding, Angew. Chem., Int. Ed., 2020, 59, 10008–10012 CrossRef CAS PubMed.
- Y. Wang, H. Wu, W. Hu and J. F. Stoddart, Adv. Mater., 2022, 34, e2105405 CrossRef PubMed.
- L. Ji, Q. He, D. Niu, J. Tan, G. Ouyang and M. Liu, Chem. Commun., 2019, 55, 11747–11750 RSC.
- Y. Yu, Y. Li, X. Wang, H. Nian, L. Wang, J. Li, Y. Zhao, X. Yang, S. Liu and L. Cao, J. Org. Chem., 2017, 82, 5590–5596 CrossRef CAS PubMed.
- Y.-L. Ma, C. Sun, Z. Li, Z. Wang, J. Wei, Q. Cheng, L.-S. Zheng, X.-Y. Chang, K. Li, R. Wang and W. Jiang, CCS Chem., 2022, 4, 1977–1989 CrossRef CAS.
- H. G. Fu, Y. Chen, Q. Yu and Y. Liu, Chem. Commun., 2019, 55, 3148–3151 RSC.
- Y. Q. Cheng, Y. X. Yue, H. M. Cao, W. C. Geng, L. X. Wang, X. Y. Hu, H. B. Li, Q. Bian, X. L. Kong, J. F. Liu, D. L. Kong, D. S. Guo and Y. B. Wang, J. Nanobiotechnol., 2021, 19, 451–473 CrossRef CAS PubMed.
- H. Zheng, C. Yuan, J. Cai, W. Pu, P. Wu, C. Li, G. Li, Y. Zhang, J. Zhang, J. Guo and D. Huang, J. Nanobiotechnol., 2022, 20, 134–152 CrossRef CAS PubMed.
- S. Qi, H. Zhang, X. Zhang, X. Yu, Y. Wang, Q. F. Meng, K. Yang, B. Bai, R. Tian, S. Zhu, L. Rao, F. Huang and G. Yu, Sci. Bull., 2022, 67, 1898–1909 CrossRef CAS PubMed.
- Y. Li, Y. Dong, X. Miao, Y. Ren, B. Zhang, P. Wang, Y. Yu, B. Li, L. Isaacs and L. Cao, Angew. Chem., Int. Ed., 2018, 57, 729–733 CrossRef CAS PubMed.
- Y. Li, C. Qin, Q. Li, P. Wang, X. Miao, H. Jin, W. Ao and L. Cao, Adv. Opt. Mater., 2020, 8, 1902154 CrossRef CAS.
- B. Yang, X.-D. Zhang, J. Li, J. Tian, Y.-P. Wu, F.-X. Yu, R. Wang, H. Wang, D.-W. Zhang, Y. Liu, L. Zhou and Z.-T. Li, CCS Chem., 2019, 1, 156–165 CAS.
- Y. Liu, C. Z. Liu, Z. K. Wang, W. Zhou, H. Wang, Y. C. Zhang, D. W. Zhang, D. Ma and Z. T. Li, Biomaterials, 2022, 284, 121467 CrossRef CAS PubMed.
- Q. Wang, Q. Zhang, Q. W. Zhang, X. Li, C. X. Zhao, T. Y. Xu, D. H. Qu and H. Tian, Nat. Commun., 2020, 11, 158–168 CrossRef PubMed.
- H. Wu, Y. Chen, X. Dai, P. Li, J. F. Stoddart and Y. Liu, J. Am. Chem. Soc., 2019, 141, 6583–6591 CrossRef CAS PubMed.
- J. Wang, Z. Huang, X. Ma and H. Tian, Angew. Chem., Int. Ed., 2020, 59, 9928–9933 CrossRef CAS PubMed.
- M. Huo, X. Y. Dai and Y. Liu, Angew. Chem., Int. Ed., 2021, 60, 27171–27177 CrossRef CAS PubMed.
- J. Zhang, H. Zhou, H. Liu, J. Hu and S. Liu, Macromol. Rapid Commun., 2018, 39, 1700225 CrossRef PubMed.
- H. Guo, X. Yan, B. Lu, J. Wang, X. Yuan, Y. Han, Y. Ding, Y. Wang, C. Yan and Y. Yao, J. Mater. Chem. C, 2020, 8, 15622–15625 RSC.
- F. Bi, C. Zhang, G. Yang, J. Wang, W. Zheng, Z. Hua, X. Li, Z. Wang and G. Chen, Polym. Chem., 2021, 12, 3096–3104 RSC.
- B. Li, Z. Meng, Q. Li, X. Huang, Z. Kang, H. Dong, J. Chen, J. Sun, Y. Dong, J. Li, X. Jia, J. L. Sessler, Q. Meng and C. Li, Chem. Sci., 2017, 8, 4458–4464 RSC.
- S. Guo, Q. Huang, Y. Chen, J. Wei, J. Zheng, L. Wang, Y. Wang and R. Wang, Angew. Chem., Int. Ed., 2021, 60, 618–623 CrossRef CAS PubMed.
- Z. H. Yu, X. Li, F. Xu, X. L. Hu, J. Yan, N. Kwon, G. R. Chen, T. Tang, X. Dong, Y. Mai, D. Chen, J. Yoon, X. P. He and H. Tian, Angew. Chem., Int. Ed., 2020, 59, 3658–3664 CrossRef CAS PubMed.
- J. Wang, F. Li, Z. Xu, M. Zang, S. Liu, T. Li, J. Xu, H. Sun, S. Yu and J. Liu, Chem. Eng. J., 2022, 444, 136620 CrossRef CAS.
- B. Xie, H. Zhao, M. Shui, Y. F. Ding, C. Sun, Z. Wang, C. Gao, G. Chen and R. Wang, Small, 2022, 18, e2201971 CrossRef PubMed.
- G. Sun, Z. He, M. Hao, Z. Xu, X. Y. Hu, J. J. Zhu and L. Wang, Chem. Commun., 2019, 55, 10892–10895 RSC.
- H. Duan, F. Cao, M. Zhang, M. Gao and L. Cao, Chin. Chem. Lett., 2022, 33, 2459–2463 CrossRef CAS.
- B. Lu, Z. Zhang, Y. Ji, S. Zhou, B. Jia, Y. Zhang, J. Wang, Y. Ding, Y. Wang, Y. Yao and X. Zhan, Sci. China: Chem., 2022, 65, 1134–1141 CrossRef CAS.
- M.-W. Yang, J.-G. Fan, J.-J. Du, K.-Y. Pu and X.-J. Peng, Chem. Soc. Rev., 2020, 49, 6800–6815 RSC.
- S. Singh, A. Aggarwal, N. V. Bhupathiraju, G. Arianna, K. Tiwari and C. M. Drain, Chem. Rev., 2015, 115, 10261–10306 CrossRef CAS PubMed.
- N. Song, Z. Zhang, P. Liu, Y. W. Yang, L. Wang, D. Wang and B. Z. Tang, Adv. Mater., 2020, 32, e2004208 CrossRef PubMed.
- H. Liu, Q. Pan, C. Wu, J. Sun, T. Zhuang, T. Liang, X. Mu, X. Zhou, Z. Li and Y. Zhao, Mater. Chem. Front., 2019, 3, 1532–1537 RSC.
- H. Liu, M. Lin, Y. Cui, W. Gan, J. Sun, B. Li and Y. Zhao, Chem. Commun., 2021, 57, 10190–10193 RSC.
- G. Yu, G. Tang and F. Huang, J. Mater. Chem. C, 2014, 2, 6609–6617 RSC.
- J. Wang, Y. Li and G. Nie, Nat. Rev. Mater., 2021, 6, 766–783 CrossRef CAS PubMed.
- D.-W. Lee, J. Jo, D. Jo, J. Kim, J.-J. Min, D. H. Yang and H. Hyun, J. Ind. Eng. Chem., 2018, 57, 37–44 CrossRef CAS.
- C. Belli, D. Trapani, G. Viale, P. D'Amico, B. A. Duso, P. Della Vigna, F. Orsi and G. Curigliano, Cancer Treat. Rev., 2018, 65, 22–32 CrossRef CAS PubMed.
- P. Wang, C. Zhang, H. W. Liu, M. Xiong, S. Y. Yin, Y. Yang, X. X. Hu, X. Yin, X. B. Zhang and W. Tan, Chem. Sci., 2017, 8, 8214–8220 RSC.
- C. Zhang, P. Wang, X. Yin, H. W. Liu, Y. Yang, L. Cheng, G. Song and X. B. Zhang, Anal. Chem., 2019, 91, 6371–6377 CrossRef CAS PubMed.
- P. Xiao, C. Liu, T. Ma, X. Lu, L. Jing, Y. Hou, P. Zhang, G. Huang and M. Gao, Adv. Sci., 2021, 8, 2004044 CrossRef CAS PubMed.
- W. C. Geng, S. Jia, Z. Zheng, Z. Li, D. Ding and D. S. Guo, Angew. Chem., Int. Ed., 2019, 58, 2377–2381 CrossRef CAS PubMed.
- Z. Liu, W. Zhou, J. Li, H. Zhang, X. Dai, Y. Liu and Y. Liu, Chem. Sci., 2020, 11, 4791–4800 RSC.
- M. Li, A. Lee, K. L. Kim, J. Murray, A. Shrinidhi, G. Sung, K. M. Park and K. Kim, Angew. Chem., Int. Ed., 2018, 57, 2120–2125 CrossRef CAS PubMed.
- X. M. Chen, Y. Chen, Q. Yu, B. H. Gu and Y. Liu, Angew. Chem., Int. Ed., 2018, 57, 12519–12523 CrossRef CAS PubMed.
- D. E. Lee, H. Koo, I. C. Sun, J. H. Ryu, K. Kim and I. C. Kwon, Chem. Soc.
Rev., 2012, 41, 2656–2672 RSC.
- S. Chen, Y. Yang, H. Li, X. Zhou and M. Liu, Chem. Commun., 2014, 50, 283–285 RSC.
- S. Kaida, H. Cabral, M. Kumagai, A. Kishimura, Y. Terada, M. Sekino, I. Aoki, N. Nishiyama, T. Tani and K. Kataoka, Cancer Res., 2010, 70, 7031–7041 CrossRef CAS PubMed.
- L. Avram and A. Bar-Shir, Org. Chem. Front., 2019, 6, 1503–1512 RSC.
- Z. Zhou, Z. Han and Z. R. Lu, Biomaterials, 2016, 85, 168–179 CrossRef CAS PubMed.
- M. Zhou, L. Li, W. Xie, Z. He and J. Li, Macromol. Rapid Commun., 2021, 42, e2100248 CrossRef PubMed.
- L. Huang, S. Zhao, J. Wu, L. Yu, N. Singh, K. Yang, M. Lan, P. Wang and J. S. Kim, Coord. Chem. Rev., 2021, 438, 213892 CrossRef.
- J. H. Correia, J. A. Rodrigues, S. Pimenta, T. Dong and Z. Yang, Pharmaceutics, 2021, 13, 1332–1349 CrossRef CAS PubMed.
- W. Fan, P. Huang and X. Chen, Chem. Soc. Rev., 2016, 45, 6488–6519 RSC.
- B. Yuan, H. Wu, H. Wang, B. Tang, J. F. Xu and X. Zhang, Angew. Chem., Int. Ed., 2021, 60, 706–710 CrossRef CAS PubMed.
- X. Li, S. Lee and J. Yoon, Chem. Soc. Rev., 2018, 47, 1174–1188 RSC.
- K. Yang, J. Wen, S. Chao, J. Liu, K. Yang, Y. Pei and Z. Pei, Chem. Commun., 2018, 54, 5911–5914 RSC.
- J. Tian, L. Xia, J. Wu, B. Huang, H. Cao and W. Zhang, ACS Appl. Mater. Interfaces, 2020, 12, 32352–32359 CrossRef CAS PubMed.
- L. Shao, Y. Pan, B. Hua, S. Xu, G. Yu, M. Wang, B. Liu and F. Huang, Angew. Chem., Int. Ed., 2020, 59, 11779–11783 CrossRef CAS PubMed.
- H. T. Feng, Y. Li, X. Duan, X. Wang, C. Qi, J. W. Y. Lam, D. Ding and B. Z. Tang, J. Am. Chem. Soc., 2020, 142, 15966–15974 CrossRef CAS PubMed.
- Y. Xu, W. Tuo, L. Yang, Y. Sun, C. Li, X. Chen, W. Yang, G. Yang, P. J. Stang and Y. Sun, Angew. Chem., Int. Ed., 2022, 61, e202110048 CAS.
- I. Roy, S. Bobbala, R. M. Young, Y. Beldjoudi, M. T. Nguyen, M. M. Cetin, J. A. Cooper, S. Allen, O. Anamimoghadam, E. A. Scott, M. R. Wasielewski and J. F. Stoddart, J. Am. Chem. Soc., 2019, 141, 12296–12304 CrossRef CAS PubMed.
- K. X. Teng, L. Y. Niu, Y. F. Kang and Q. Z. Yang, Chem. Sci., 2020, 11, 9703–9711 RSC.
- K. X. Teng, L. Y. Niu, N. Xie and Q. Z. Yang, Nat. Commun., 2022, 13, 6179–6189 CrossRef CAS PubMed.
- X. Li, J. F. Lovell, J. Yoon and X. Chen, Nat. Rev. Clin. Oncol., 2020, 17, 657–674 CrossRef PubMed.
- H. S. Jung, P. Verwilst, A. Sharma, J. Shin, J. L. Sessler and J. S. Kim, Chem. Soc. Rev., 2018, 47, 2280–2297 RSC.
- B. Tang, W. L. Li, Y. Chang, B. Yuan, Y. Wu, M. T. Zhang, J. F. Xu, J. Li and X. Zhang, Angew. Chem., Int. Ed., 2019, 58, 15526–15531 CrossRef CAS PubMed.
- T. X. Zhang, X. Hou, Y. Kong, F. Yang, Y. X. Yue, M. R. Shah, H. B. Li, F. Huang, J. Liu and D. S. Guo, Theranostics, 2022, 12, 396–409 CrossRef CAS PubMed.
- B. Zhang, Q. Yu, Y. M. Zhang and Y. Liu, Chem. Commun., 2019, 55, 12200–12203 RSC.
- Q. Cheng, L. Yue, J. Li, C. Gao, Y. Ding, C. Sun, M. Xu, Z. Yuan and R. Wang, Small, 2021, 17, e2101332 CrossRef PubMed.
- W. Fan, B. Yung, P. Huang and X. Chen, Chem. Rev., 2017, 117, 13566–13638 CrossRef CAS PubMed.
- X. Deng, Z. Shao and Y. Zhao, Adv. Sci., 2021, 8, 2002504 CrossRef CAS PubMed.
- X. Zhen, J. Zhang, J. Huang, C. Xie, Q. Miao and K. Pu, Angew. Chem., Int. Ed., 2018, 57, 7804–7808 CrossRef CAS PubMed.
- J. Yuan, Q. H. Zhou, S. Xu, Q. P. Zuo, W. Li, X. X. Zhang, T. B. Ren, L. Yuan and X. B. Zhang, Angew. Chem., Int. Ed., 2022, 61, e202206169 CAS.
- N. Song, Z. Zhang, P. Liu, D. Dai, C. Chen, Y. Li, L. Wang, T. Han, Y. W. Yang, D. Wang and B. Z. Tang, Adv. Funct. Mater., 2021, 31, 2009924 CrossRef CAS.
- S. Senapati, A. K. Mahanta, S. Kumar and P. Maiti, Signal Transduction Targeted Ther., 2018, 3, 7–27 CrossRef PubMed.
- W. H. Chen, G. F. Luo, W. X. Qiu, Q. Lei, L. H. Liu, S. B. Wang and X. Z. Zhang, Biomaterials, 2017, 117, 54–65 CrossRef CAS PubMed.
- G. G. Yang, H. Zhang, D. Y. Zhang, Q. Cao, J. Yang, L. N. Ji and Z. W. Mao, Biomaterials, 2018, 185, 73–85 CrossRef CAS PubMed.
- G. Yu, B. Zhu, L. Shao, J. Zhou, M. L. Saha, B. Shi, Z. Zhang, T. Hong, S. Li, X. Chen and P. J. Stang, Proc. Natl. Acad. Sci. U. S. A., 2019, 116, 6618–6623 CrossRef CAS PubMed.
- X. Cheng, R. Sun, L. Yin, Z. Chai, H. Shi and M. Gao, Adv. Mater., 2017, 29, 1–6 Search PubMed.
- H. Chen, X. Zeng, H. P. Tham, S. Z. F. Phua, W. Cheng, W. Zeng, H. Shi, L. Mei and Y. Zhao, Angew. Chem., Int. Ed., 2019, 58, 7641–7646 CrossRef CAS PubMed.
- J. Chen, S. Li, Z. Wang, Y. Pan, J. Wei, S. Lu, Q. W. Zhang, L. H. Wang and R. Wang, Chem. Sci., 2021, 12, 7727–7734 RSC.
- Y. Zhang, D. Yang, H. Chen, W. Q. Lim, F. S. Z. Phua, G. An, P. Yang and Y. Zhao, Biomaterials, 2018, 163, 14–24 CrossRef CAS PubMed.
- G. B. Frisoni, M. Boccardi, F. Barkhof, K. Blennow, S. Cappa, K. Chiotis, J.-F. Démonet, V. Garibotto, P. Giannakopoulos, A. Gietl, O. Hansson, K. Herholz, C. R. Jack, F. Nobili, A. Nordberg, H. M. Snyder, M. Ten Kate, A. Varrone, E. Albanese, S. Becker, P. Bossuyt, M. C. Carrillo, C. Cerami, B. Dubois, V. Gallo, E. Giacobini, G. Gold, S. Hurst, A. Lönneborg, K.-O. Lovblad, N. Mattsson, J.-L. Molinuevo, A. U. Monsch, U. Mosimann, A. Padovani, A. Picco, C. Porteri, O. Ratib, L. Saint-Aubert, C. Scerri, P. Scheltens, J. M. Schott, I. Sonni, S. Teipel, P. Vineis, P. J. Visser, Y. Yasui and B. Winblad, Lancet Neurol., 2017, 16, 661–676 CrossRef PubMed.
- P. J. Tighe, R. R. Ryder, I. Todd and L. C. Fairclough, Proteomics Clin Appl, 2015, 9, 406–422 CrossRef CAS PubMed.
- T. L. Mako, J. M. Racicot and M. Levine, Chem. Rev., 2019, 119, 322–477 CrossRef CAS PubMed.
- S. Sinn, E. Spuling, S. Brase and F. Biedermann, Chem. Sci., 2019, 10, 6584–6593 RSC.
- S. Zhang, L. Grimm, Z. Miskolczy, L. Biczok, F. Biedermann and W. M. Nau, Chem. Commun., 2019, 55, 14131–14134 RSC.
- C. Hu, L. Grimm, A. Prabodh, A. Baksi, A. Siennicka, P. A. Levkin, M. M. Kappes and F. Biedermann, Chem. Sci., 2020, 11, 11142–11153 RSC.
- C. Hu, T. Jochmann, P. Chakraborty, M. Neumaier, P. A. Levkin, M. M. Kappes and F. Biedermann, J. Am. Chem. Soc., 2022, 144, 13084–13095 CrossRef CAS PubMed.
- W. Tuo, Y. Xu, Y. Fan, J. Li, M. Qiu, X. Xiong, X. Li and Y. Sun, Coord. Chem. Rev., 2021, 443, 214017 CrossRef CAS.
- C. Li, Y. Xu, L. Tu, M. Choi, Y. Fan, X. Chen, J. L. Sessler, J. S. Kim and Y. Sun, Chem. Sci., 2022, 13, 6541–6549 RSC.
- Y. Xu, C. Li, X. Ma, W. Tuo, L. Tu, X. Li, Y. Sun, P. J. Stang and Y. Sun, Proc. Natl. Acad. Sci. U. S. A., 2022, 119, e2209904119 CrossRef CAS PubMed.
- L. Tu, C. Li, C. Liu, S. Bai, J. Yang, X. Zhang, L. Xu, X. Xiong and Y. Sun, Chem. Commun., 2022, 58, 9068–9071 RSC.
- Y. Fan, C. Li, S. Bai, X. Ma, J. Yang, X. Guan and Y. Sun, Small, 2022, 18, e2201625 CrossRef PubMed.
- Y. Pang, C. Li, H. Deng and Y. Sun, Dalton Trans., 2022, 51, 16428–16438 RSC.
- S. Gnaim, A. Scomparin, A. Eldar-Boock, C. R. Bauer, R. Satchi-Fainaro and D. Shabat, Chem. Sci., 2019, 10, 2945–2955 RSC.
- N. M. Kumar, P. Picchetti, C. Hu, L. M. Grimm and F. Biedermann, ACS Sens., 2022, 7, 2312–2319 CrossRef CAS PubMed.
- L. Chen, Y. Chen, W. Zhou, J. Li, Y. Zhang and Y. Liu, Chem. Commun., 2020, 56, 8857–8860 RSC.
Footnote |
† These authors contributed equally to this work. |
|
This journal is © The Royal Society of Chemistry 2023 |