DOI:
10.1039/D1TC05711A
(Paper)
J. Mater. Chem. C, 2022,
10, 4941-4946
Efficient narrowband electroluminescence based on a hetero-bichromophore thermally activated delayed fluorescence dyad†
Received
27th November 2021
, Accepted 24th January 2022
First published on 25th January 2022
Abstract
A hetero-bichromophore thermally activated delayed fluorescence (TADF) emitter named BOQAO was designed and synthesized, which consists of two multi-resonance TADF (MR-TADF) cores, tBuBO and tBuQAO. The short-range intramolecular charge transfer (ICT) characteristics of tBuBO and tBuQAO weaken its electron-donating and electron-withdrawing ability, respectively. Thus, weak long-range ICT from tBuBO to tBuQAO is established in BOQAO for holding blue fluorescence emission with a slight redshift, keeping a small FWHM, and maintaining the TADF properties, simultaneously. The simple doped devices exhibited high color purity with FWHMs of less than 35 nm in a wide doping ratio range from 1 wt% to 40 wt%. At a 5 wt% doping ratio, the device based on BOQAO exhibited the best device performance with an EQE of 21.8% and an FWHM of 32 nm. This work provides a simple and practical strategy for suppressing aggregation-induced spectral broadening for the development of MR-TADF.
Introduction
Commercial organic light-emitting diode (OLED) technology requires a high device efficiency, good color purity, and a long device lifetime simultaneously.1–5 In the past decade, thermally activated delayed fluorescence (TADF) OLEDs have already demonstrated their potential as the third generation of OLEDs due to their 100% exciton utilization and low cost. In a TADF molecule, the small energy splitting between the lowest excited singlet (S1) and triplet (T1) states, ΔEST, provides a practical pathway for harvesting non-emissive triplet excitons through the spin-flipping reverse intersystem crossing (RISC) process.6,7 To achieve an effective RISC process, the general strategy is to reduce the exchange energy between the highest occupied molecular orbital (HOMO) and the lowest unoccupied molecular orbital (LUMO) by constructing a donor–acceptor (D–A) type emitter with a twisted structure.8–11 However, the subsequent conformation relaxation caused by the twisted structures usually enhances the structure relaxation and leads to a wide emission with a full-width at half maximum (FWHM) of >70 nm, which is unsuitable for commercial displays.12
To withstand the broadened FWHM of conventional D–A-type TADF emitters, Hatakeyama et al. proposed the multi-resonance (MR) TADF emitter named DABNA-1, which exhibited a pure blue emission centered at 459 nm with an external quantum efficiency (EQE) of 13.5% and an impressively small FWHM of 28 nm.13 For DABNA-1, the ortho-positioned boron and nitrogen atoms lead to the multi-resonance effect for facilitating the RISC process; meanwhile, the rigid planar polycyclic aromatic framework ensures the narrow emission. Hereafter, TADF emitters with a narrow FWHM were widely studied and more chromophores with the MR effect were develeoped.14–21 Among them, oxygen-bridged triarylboranes have also been exploited as narrowband emitters, and the parent molecule DABOA14 (2a) exhibited a narrow emission (FWHM = 34 nm) with a λPL of 398 nm in solution.22 In 2019, our group disclosed another successful example, QAO, which bears MR-TADF activity aside from the prevalent B/N or B/O system, which consisted of a triphenylamine having two para-disposed carbonyl bridges, and its electroluminescent device achieved an EQE of 19.4% with a narrow FWHM of 39 nm.23 This result provides a promising candidate for the design of highly efficient and narrowband organic emitters.
Although the above-mentioned MR-TADF cores improve the color purity immensely with FWHMs of less than 40 nm, their RISC rates are still limited, which is caused by the same π–π* transition characteristic for both S1 and T1 states.24 The introduction of general electron donors (arylamines) or acceptors has proven an effective strategy, which creates a long-range intramolecular charge transfer (ICT) state for improving the device efficiency and tuning the bandgap.25–44 This strategy usually improved the color purity due to the vanished shoulder peak. However, such modification for blue MR-TADF emitters usually enhanced the structural relaxation, broadened the emission spectra, and redshifted the emission band. For instance, the introduction of 9,9-dimethyl-9,10-dihydroacridine (DMAC) at the QAO core caused a large dihedral angle and a wide emission band (FWHM > 70 nm).23 Moreover, the utilization of a conventional electron donor with moderate steric hindrance (i.e., carbazole) could also virtually decrease the color purity (FWHM ≈ 50 nm).33 Thus, the introduction of a weak ICT state could be a practical pathway to develop blue MR-TADF emitters.
Herein, we combined two MR-TADF cores (tBuBO and tBuQAO) to construct a hetero-bichromophore dyad named BOQAO. The difference in electron-donating and electron-withdrawing ability of the MR segments drives a long-range ICT interaction, where tBuBO and tBuQAO serve as an electron-donor and electron-acceptor, respectively. Compared with the emission peak of tBuQAO in dilute toluene solution, the slight redshift (7 nm) of BOQAO indicates weak long-range ICT. In doped 4,4′-bis(N-carbazolyl)-1,1′-biphenyl (CBP) films with wide doping ratios (1–30 wt%), BOQAO exhibits high color purity with small FWHMs of less than 40 nm. The doped devices also suggest high color purity within a wide doping ratio range (1–40 wt%). At a 5 wt% doping ratio, the device based on BOQAO exhibited the best device performance with an EQE of 21.8% and an FWHM of 32 nm.
Results and discussion
Synthesis and characterization
The synthetic route for BOQAO is summarized in Scheme 1. For 2,5-dibromo-1,3-dimethylbenzene (1), the dimethyl group can be easily converted to the diester via an oxidation and esterification reaction.45 After the Ullmann reaction, hydrolysis, and the Friedel–Crafts acylation reaction, the bromine-containing tBuQAO segment (6) can be obtained. After the Miyaura borylation reaction, intermediate 7 was obtained with a high yield (84%). The target compound BOQAO was obtained via the Suzuki–Miyaura coupling reaction with an oxygen-bridged triarylborane scaffold (8). The characterization results are presented in Fig. S12–S16 (ESI†). The newly reported compounds were characterized using 1H-NMR, 13C-NMR, and mass spectra. We also evaluate the thermal stability of BOQAOvia thermogravimetric analysis (TGA) and differential scanning calorimetry (DSC). As shown in Fig. S1 (ESI†), the high decomposition temperature (Td, corresponding to a 5% weight loss) of 485 °C suggest its outstanding thermal stability.46–48 No obvious glass transition temperature (Tg) was found in the DSC curve.
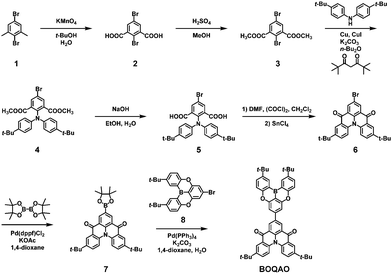 |
| Scheme 1 Synthetic route for BOQAO. | |
Single crystal
As shown in Fig. 1 and Table S3 (ESI†), the single crystal was obtained via the slow liquid diffusion of petroleum ether in a dichloromethane solution of BOQAO at room temperature. The tBuQAO segment exhibits a twisted helicene structure with a dihedral angle of 43.6° between the terminal phenyl rings, and the tBuBO fragment maintains a relatively planar structure with a dihedral angle of 13.4° between the corresponding phenyl rings. In addition, the dyad maintains a torsion angle of 33.7° between the tBuBO and tBuQAO moieties. The dumbbell-shaped structure of BOQAO leads to a mixed parallel H-aggregate and J-aggregate packing.49 In detail, the bulky tert-butyl groups and different helicene-shaped tBuBO and tBuQAO moieties cause a relatively large distance of 3.31 Å for the H-aggregate dimer and a short distance of 3.19 Å between the adjacent J-aggregate dimers, respectively. Such a packing mode makes BOQAO maintain high intermolecular restrictions, which is favorable for high fluorescence efficiency in the aggregation states.
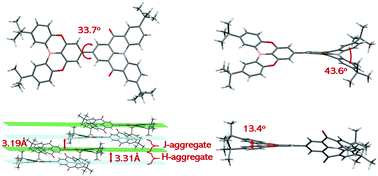 |
| Fig. 1 Single crystal structure and packing mode of BOQAO. | |
Simulation and electrochemical properties
Density functional theory (DFT) and time-dependent DFT (TD-DFT) were employed to evaluate the molecular configurations and orbitals (Fig. S2 in the ESI†). The highest occupied molecular orbital (HOMO) and the lowest unoccupied molecular orbital (LUMO) of BOQAO are located at the tBuBO and tBuQAO segments, respectively. Such well-separated frontier molecular orbitals (FMOs) feed the requirement for typical D–A-type TADF emitters with a small ΔEST, which also indicated that ICT from tBuBO to tBuQAO could occur for fluorescence emission.50 Furthermore, BOQAO exhibits a small structural relaxation, which is supported by the calculated S0 and S1 geometries.51 Besides, the dipole moment of BOQAO of the S0 state is as small as 3.017 Debye, which is favorable for suppressing the redshift caused by intermolecular interactions.
Furthermore, the energy levels of BOQAO were evaluated through cyclic voltammetry (CV) measurements (Fig. 2). The quasi-reversible oxidation and irreversible reduction potentials of BOQAO are 1.49 and −1.22 V, respectively. Furthermore, the corresponding HOMO and LUMO energy levels of BOQAO are calculated to be −5.77 and −3.06 eV, respectively. The bandgap for BOQAO is 2.71 eV. The wide bandgap suggests that BOQAO would still maintain blue fluorescence. The CV curves of the parents tBuBO and tBuQAO were also measured for comparison (Fig. 2). Evaluating the positions and curve shapes, the tBuBO and tBuQAO moieties in BOQAO serve as electron-donor and -acceptor, respectively, which agrees with the calculated HOMO and LUMO distributions.46,48
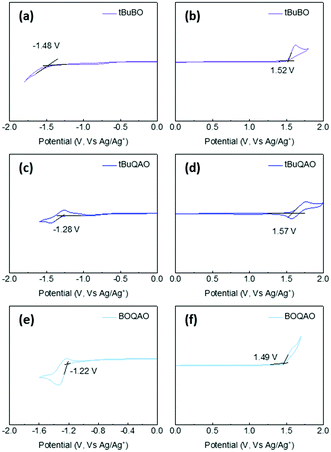 |
| Fig. 2 Cyclic voltammetry (CV) curves of (a and b) tBuBO, (c and d) tBuQAO, and (e and f) BOQAO. | |
Photophysical properties
The photophysical properties of BOQAO and their parent segments tBuBO and tBuQAO were investigated simultaneously in solution (Fig. 3 and Fig. S5–S7 in the ESI†). In degassed dilute toluene solution, BOQAO exhibits a blue emission peak maximum at 474 nm and an FWHM of 28 nm. Compared with the fluorescence spectra of tBuQAO (Fig. S5c, ESI,† peak at 467 nm and an FWHM of 29 nm), the slight redshift (7 nm) indicates that the long-range ICT from tBuBO to tBuQAO is weak and the electron-donating ability of tBuBO and the electron-withdrawing ability tBuQAO in BOQAO are weakened by the short-range ICT of the segments. The symmetrical absorption and fluorescence spectra with small FWHM values suggest the strong low-frequency vibrionic coupling in this system.52 Furthermore, the small Stokes shift of 925 cm−1 indicates that the dyad still maintained a small reorganization energy between the ground and excited conformations, which is consistent with the small configuration difference between the S0 and S1 states (Fig. S2 in the ESI†).51 Compared with the absorption band at 300–400 nm (corresponding to the higher excited state), the lower intensity of the lowest absorption band (centered at 455 nm, corresponding to the lowest excited state) indicates that the long-range ICT does work.53 Furthermore, the lowest absorption band of BOQAO is slightly redshifted but maintains a MR-type shape compared with the absorption band of the parents tBuBO and tBuQAO (Fig. S5 in the ESI†), which indicates that the weak long-range ICT interactions between the two MR segments does not have a significant influence on the FWHM.54 The fluorescence spectra in different polar solvents were also investigated (Fig. 3b and Fig. S6, Table S1 in the ESI†). An obvious redshift and broadened spectra can be observed. For instance, BOQAO exhibits a blue emission with a peak at 456 nm and an FWHM of 24 nm in cyclohexane solution and a sky-blue emission with a peak at 484 nm and an FWHM of 38 nm in dichloromethane solution. To evaluate the S1 and T1 energy levels, we also measured the fluorescence and phosphorescence spectra of BOQAO and its parent segments tBuBO and tBuQAO in toluene at 77 K (Fig. S7 in the ESI†). The calculated S1 and T1 energy levels of BOQAO are 2.68 and 2.46 eV, respectively. Thus, the BOQAO maintains a relatively small ΔEST of 0.22 eV, which is comparable to tBuBO (ΔEST = 0.19 eV) and tBuQAO (ΔEST = 0.22 eV), to support the RISC process for triplet exciton utilization. The triplet spin density of BOQAO (Fig. S3 in the ESI†) indicates that the tBuBO segment partly contributes to the triplet excited states.
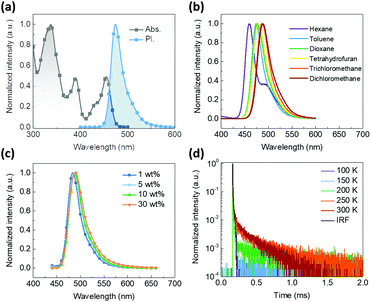 |
| Fig. 3 Photophysical properties of BOQAO. (a) Absorption and fluorescence spectra of BOQAO in dilute toluene solution (10−5 mol L−1) at room temperature. (b) Fluorescence spectra of BOQAO in different solutions. (c) Fluorescence spectra of BOQAO in doped CBP films. (d) Transient spectra of 5 wt% BOQAO in CBP at different temperatures. | |
Moreover, BOQAO exhibited narrowband sky-blue emission with a negligible redshift when doped in CBP with the doping ratio increasing from 1 to 30 wt% (Fig. 3c). The emission peaks of BOQAO at 1, 5, 10, and 30 wt% in CBP are 483, 487, 489, and 490 nm with FWHMs of 31, 33, 34, and 38 nm, respectively. Such properties are uncommon among MR-TADF emitters, which are a result of low dipolar moment, highly molecular rigidity, and intermolecular restrictions in the aggregation state.26,55,56 Furthermore, these results also indicate that our strategy can effectively suppress spectral broadening. The TADF characteristics of BOQAO were further investigated via their fluorescence decay curves at 5 wt% in CBP at different temperatures (Fig. 3d). The prompt and delayed fluorescence lifetimes are 6 ns and 214 μs, respectively. Moreover, the decayed fluorescence exhibits obvious temperature-dependent properties. In addition, the absolute PL quantum yields (PLQY) of the doped films of 5 wt% tBuBO, tBuQAO, and BOQAO in CBP were measured to be 97.0%, 93.1%, and 98.7%, respectively, which indicate that the hetero-bichromophore emitter BOQAO exhibited improved the PLQY. The fluorescence decay curves and PLQY values of tBuBO and tBuQAO doped in CBP films (Fig. S9 and Tables S3, S4 in the ESI†) were also measured to evaluate the kinetics of the excited states. Compared with tBuBO and tBuQAO, BOQAO maintains a comparable singlet radiative transition rate constant (kSr = 3.2 × 107) and RISC rate constant (kRISC = 2.4 × 104). Furthermore, BOQAO exhibits less triplet energy loss, which is supported by the small triplet nonradiative rate constant (kTnr = 7.5 × 101).
Electroluminescence properties
To evaluate the device performance, simple doped devices were fabricated with the structure of indium tin oxide (ITO)/1,4,5,8,9,11-hexaazatriphenylene hexacarbonitrile (HAT-CN, 10 nm)/1,1-bis[4-[N,N-di(p-tolyl)amino]phenyl]-cyclohexane (TAPC, 40 nm)/tris(4-carbazolyl-9-ylphenyl)amine (TCTA, 10 nm)/CBP: x wt% BOQAO (20 nm)/4,6-bis(3,5-di(pyridin-4-yl)phenyl)-2-phenylpyrimidine (TmPyPB, 45 nm)/8-hydroxyquinolinolato lithium (Liq, 2 nm)/Al (100 nm). TAPC and TmPyPB were chosen as the hole-transporting layer and the electron-transporting layer, respectively, and TCTA was adopted as an exciton-blocking layer for confining excitons due to its high triplet energy level. Fig. S10 (in the ESI†) shows the energy level diagram of the devices and the molecular structures of the related functional materials.
As shown in Fig. 4a, the electroluminescence spectra of all the devices with 1, 5, 10 wt% doping ratios maintain a sky-blue emission with peaks at 484 nm and FWHMs of 32 nm, respectively, which match the fluorescence spectra of the corresponding films. The devices with a higher doping ratio (up to 40 wt%) also maintain impressively small FWHMs in the sky-blue region (Fig. S11 in the ESI†). Among these devices, the device with a 5 wt% doping ratio of BOQAO in CBP exhibits the best device performance with a maximum external quantum efficiency (EQEmax) of 21.8%, which is the best performance among the reported QAO derivatives in the blue region (Table S4 in the ESI†).
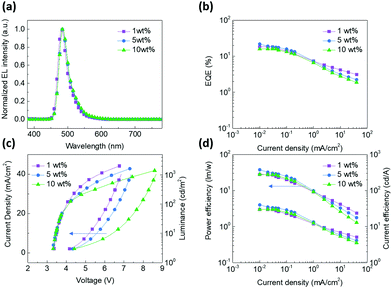 |
| Fig. 4 OLED device performance for BOQAO. (a) Normalized electroluminescence spectrum. (b) EQE–current density curves. (c) Current density–voltage–luminescence curves. (d) Power efficiency–current density–current efficiency curves. | |
Conclusions
In summary, an MR-TADF emitter named BOQAO, which consists of two MR segments tBuBO and tBuQAO, was designed and synthesized. Long-range ICT from tBuBO fragment to tBuQAO fragment in BOQAO, which is weakened by short-range ICT in the segments, was established to tune its photophysical properties. Compared with the parent MR core tBuQAO, BOQAO exhibits a blue emission (peak at 474 nm and FWHM of 28 nm) with a slightly redshifted emission. In doped CBP films with a wide doping ratio range of 1–30 wt%, BOQAO displays a persistent blue emission with small FWHMs of less than 40 nm. This unique property is also demonstrated in the electroluminescence spectra of the doped devices. The optimized device with a 5 wt% doping ratio of BOQAO in CBP realized the best device performance with an EQEmax of 21.8% and an FWHM of 32 nm, which balanced the color purity and device efficiency. This work provides a practical strategy for suppressing spectral broadening for the development of MR-TADF.
Conflicts of interest
There are no conflicts to declare.
Acknowledgements
The authors acknowledge financial support from the National Natural Science Foundation of China (grant no. 51773141, 51873139, 61961160731, 62175171, and 22175124). This project is also funded by the Suzhou Science and Technology Plan Project (SYG202010), Collaborative Innovation Centre of Suzhou Nano Science & Technology (Nano-CIC), and the “111” Project.
References
- C. W. Tang and S. A. VanSlyke, Appl. Phys. Lett., 1987, 51, 913–915 CrossRef CAS.
- P. T. Chou and Y. Chi, Chem. – Eur. J., 2007, 13, 380–395 CrossRef CAS PubMed.
- Y. Tao, K. Yuan, T. Chen, P. Xu, H. Li, R. Chen, C. Zheng, L. Zhang and W. Huang, Adv. Mater., 2014, 26, 7931–7958 CrossRef CAS PubMed.
- S. Y. Yang, Y. K. Qu, L. S. Liao, Z. Q. Jiang and S. T. Lee, Adv. Mater., 2021, 2104125, DOI:10.1002/adma.202104125.
- M. Romain, D. Tondelier, O. Jeannin, B. Geffroy, J. Rault-Berthelot and C. Poriel, J. Mater. Chem. C, 2015, 3, 9701–9714 RSC.
- Z. Yang, Z. Mao, Z. Xie, Y. Zhang, S. Liu, J. Zhao, J. Xu, Z. Chi and M. P. Aldred, Chem. Soc. Rev., 2017, 46, 915–1016 RSC.
- H. Uoyama, K. Goushi, K. Shizu, H. Nomura and C. Adachi, Nature, 2012, 492, 234–238 CrossRef CAS PubMed.
- Y.-J. Yu, X.-Q. Wang, J.-F. Liu, Z.-Q. Jiang and L.-S. Liao, iScience, 2021, 24, 102123 CrossRef CAS PubMed.
- Y. J. Yu, X. Tang, H. T. Ge, Y. Yuan, Z. Q. Jiang and L. S. Liao, Org. Electron., 2019, 73, 240–246 CrossRef CAS.
- P. Data and Y. Takeda, Chem. – Asian J., 2019, 14, 1613–1636 CrossRef CAS PubMed.
- M. Y. Zhang, Z. Y. Li, B. Lu, Y. Wang, Y. D. Ma and C. H. Zhao, Org. Lett., 2018, 20, 6868–6871 CrossRef CAS PubMed.
- P. Data, P. Pander, M. Okazaki, Y. Takeda, S. Minakata and A. P. Monkman, Angew. Chem., Int. Ed., 2016, 55, 5739–5744 CrossRef CAS PubMed.
- T. Hatakeyama, K. Shiren, K. Nakajima, S. Nomura, S. Nakatsuka, K. Kinoshita, J. Ni, Y. Ono and T. Ikuta, Adv. Mater., 2016, 28, 2777–2781 CrossRef CAS PubMed.
- D. Hall, S. M. Suresh, P. L. dos Santos, E. Duda, S. Bagnich, A. Pershin, P. Rajamalli, D. B. Cordes, A. M. Z. Slawin, D. Beljonne, A. Köhler, I. D. W. Samuel, Y. Olivier and E. Zysman-Colman, Adv. Opt. Mater., 2019, 8, 1901627 CrossRef.
- H. Min, I. S. Park and T. Yasuda, Angew. Chem., Int. Ed., 2021, 60, 7643–7648 CrossRef CAS PubMed.
- M. Nagata, H. Min, E. Watanabe, H. Fukumoto, Y. Mizuhata, N. Tokitoh, T. Agou and T. Yasuda, Angew. Chem., Int. Ed., 2021, 60, 20280–20285 CrossRef CAS PubMed.
- G. Liu, H. Sasabe, K. Kumada, A. Matsunaga, H. Katagiri and J. Kido, J. Mater. Chem. C, 2021, 9, 8308–8313 RSC.
- Y. Kondo, K. Yoshiura, S. Kitera, H. Nishi, S. Oda, H. Gotoh, Y. Sasada, M. Yanai and T. Hatakeyama, Nat. Photonics, 2019, 13, 678–682 CrossRef CAS.
- S. Oda, B. Kawakami, R. Kawasumi, R. Okita and T. Hatakeyama, Org. Lett., 2019, 21, 9311–9314 CrossRef CAS PubMed.
- Y. Zhang, D. Zhang, J. Wei, Z. Liu, Y. Lu and L. Duan, Angew. Chem., Int. Ed., 2019, 58, 16912 CrossRef CAS PubMed.
- S. N. Zou, C. C. Peng, S. Y. Yang, Y. K. Qu, Y. J. Yu, X. Chen, Z. Q. Jiang and L. S. Liao, Org. Lett., 2021, 23, 958–962 CrossRef CAS PubMed.
- H. Hirai, K. Nakajima, S. Nakatsuka, K. Shiren, J. Ni, S. Nomura, T. Ikuta and T. Hatakeyama, Angew. Chem., Int. Ed., 2015, 54, 13581–13585 CrossRef CAS PubMed.
- Y. Yuan, X. Tang, X.-Y. Du, Y. Hu, Y.-J. Yu, Z.-Q. Jiang, L.-S. Liao and S.-T. Lee, Adv. Opt. Mater., 2019, 7, 1801536 CrossRef.
- X. Wu, B.-K. Su, D.-G. Chen, D. Liu, C.-C. Wu, Z.-X. Huang, T.-C. Lin, C.-H. Wu, M. Zhu, E. Y. Li, W.-Y. Hung, W. Zhu and P.-T. Chou, Nat. Photonics, 2021, 15, 780–786 CrossRef CAS.
- M. Yang, S. Shikita, H. Min, I. S. Park, H. Shibata, N. Amanokura and T. Yasuda, Angew. Chem., Int. Ed., 2021, 60, 23142 CrossRef CAS PubMed.
- Y. Xu, C. Li, Z. Li, Q. Wang, X. Cai, J. Wei and Y. Wang, Angew. Chem., Int. Ed., 2020, 59, 17442 CrossRef CAS PubMed.
- X. Liang, Z. P. Yan, H. B. Han, Z. G. Wu, Y. X. Zheng, H. Meng, J. L. Zuo and W. Huang, Angew. Chem., Int. Ed., 2018, 57, 11316–11320 CrossRef CAS PubMed.
- Y. Liu, X. Xiao, R. You, Z. Bin and J. You, Chem. Sci., 2021, 12, 9408–9412 RSC.
- M. Yang, I. S. Park and T. Yasuda, J. Am. Chem. Soc., 2020, 142, 19468–19472 CrossRef CAS PubMed.
- J. U. Kim, I. S. Park, C. Y. Chan, M. Tanaka, Y. Tsuchiya, H. Nakanotani and C. Adachi, Nat. Commun., 2020, 11, 1765 CrossRef CAS PubMed.
- D. H. Ahn, S. W. Kim, H. Lee, I. J. Ko, D. Karthik, J. Y. Lee and J. H. Kwon, Nat. Photonics, 2019, 13, 540–546 CrossRef CAS.
- Y. Qi, W. Ning, Y. Zou, X. Cao, S. Gong and C. Yang, Adv. Funct. Mater., 2021, 31, 2102017 CrossRef CAS.
- F. Huang, K. Wang, Y. Z. Shi, X. C. Fan, X. Zhang, J. Yu, C. S. Lee and X. H. Zhang, ACS Appl. Mater. Interfaces, 2021, 13, 36089–36097 CrossRef CAS PubMed.
- D. Karthik, Y. H. Jung, H. Lee, S. Hwang, B. M. Seo, J. Y. Kim, C. W. Han and J. H. Kwon, Adv. Mater., 2021, 33, 2007724 CrossRef CAS PubMed.
- H. Lim, H. J. Cheon, S. J. Woo, S. K. Kwon, Y. H. Kim and J. J. Kim, Adv. Mater., 2020, 32, 2004083 CrossRef CAS PubMed.
- Y. Xu, Q. Wang, X. Cai, C. Li and Y. Wang, Adv. Mater., 2021, 33, 2100652 CrossRef CAS PubMed.
- R. Braveenth, H. Lee, J. D. Park, K. J. Yang, S. J. Hwang, K. R. Naveen, R. Lampande and J. H. Kwon, Adv. Funct. Mater., 2021, 31, 2105805 CrossRef CAS.
- Y. Lee and J. I. Hong, Adv. Opt. Mater., 2021, 9, 2100406 CrossRef CAS.
- X. F. Luo, F. L. Li, J. W. Zou, Q. Zou, J. Su, M. X. Mao and Y. X. Zheng, Adv. Opt. Mater., 2021, 9, 2100784 CrossRef CAS.
- I. S. Park, H. Min, J. U. Kim and T. Yasuda, Adv. Opt. Mater., 2021, 9, 2101282 CrossRef CAS.
- C. Wu, W. Liu, K. Li, G. Cheng, J. Xiong, T. Teng, C. M. Che and C. Yang, Angew. Chem., Int. Ed., 2021, 60, 3994–3998 CrossRef CAS PubMed.
- M. Yang, S. Shikita, H. Min, I. S. Park, H. Shibata, N. Amanokura and T. Yasuda, Angew. Chem., Int. Ed., 2021, 60, 23142–23147 CrossRef CAS PubMed.
- Y. Chen, N. Li, Z. Huang, G. Xie and C. Yang, Chem. Eng. J., 2022, 430, 133078 CrossRef CAS.
- J. Hwang, H. Kang, J.-E. Jeong, H. Y. Woo, M. J. Cho, S. Park and D. H. Choi, Chem. Eng. J., 2021, 416, 129185 CrossRef CAS.
- S. K. Surampudi, G. Nagarjuna, D. Okamoto, P. D. Chaudhuri and D. Venkataraman, J. Org. Chem., 2012, 77, 2074–2079 CrossRef CAS PubMed.
- L. Sicard, C. Quinton, F. Lucas, O. Jeannin, J. Rault-Berthelot and C. Poriel, J. Phys. Chem. C, 2019, 123, 19094–19104 CrossRef CAS.
- H. Yang, Q. Liang, C. Han, J. Zhang and H. Xu, Adv. Mater., 2017, 29, 1700553 CrossRef PubMed.
- M. Romain, D. Tondelier, B. Geffroy, A. Shirinskaya, O. Jeannin, J. Rault-Berthelot and C. Poriel, Chem. Commun., 2015, 51, 1313–1315 RSC.
- N. J. Hestand and F. C. Spano, Chem. Rev., 2018, 118, 7069–7163 CrossRef CAS PubMed.
- Y. C. Liu, C. S. Li, Z. J. Ren, S. K. Yan and M. R. Bryce, Nat. Rev. Mater., 2018, 3, 18020 CrossRef CAS.
- Y. Zhang, D. Zhang, T. Huang, A. J. Gillett, Y. Liu, D. Hu, L. Cui, Z. Bin, G. Li, J. Wei and L. Duan, Angew. Chem., Int. Ed., 2021, 60, 20498 CrossRef CAS PubMed.
- X. Qiu, G. Tian, C. Lin, Y. Pan, X. Ye, B. Wang, D. Ma, D. Hu, Y. Luo and Y. Ma, Adv. Opt. Mater., 2021, 9, 2001845 CrossRef CAS.
- S. Y. Yang, S. N. Zou, F. C. Kong, X. J. Liao, Y. K. Qu, Z. Q. Feng, Y. X. Zheng, Z. Q. Jiang and L. S. Liao, Chem. Commun., 2021, 57, 11041–11044 RSC.
- X. C. Fan, K. Wang, Y. Z. Shi, J. X. Chen, F. Huang, H. Wang, Y. N. Hu, Y. Tsuchiya, X. M. Ou, J. Yu, C. Adachi and X. H. Zhang, Adv. Opt. Mater., 2021, 10, 2101789 CrossRef.
- Y. Zhang, J. Wei, D. Zhang, C. Yin, G. Li, Z. Liu, X. Jia, J. Qiao and L. Duan, Angew. Chem., Int. Ed., 2021, 61, e202113206 Search PubMed.
- P. Jiang, J. Miao, X. Cao, H. Xia, K. Pan, T. Hua, X. Lv, Z. Huang, Y. Zou and C. Yang, Adv. Mater., 2021, 2106954, DOI:10.1002/adma.202106954.
Footnotes |
† Electronic supplementary information (ESI) available. CCDC 2057629. For ESI and crystallographic data in CIF or other electronic format see DOI: 10.1039/d1tc05711a |
‡ These authors contributed equally. |
|
This journal is © The Royal Society of Chemistry 2022 |