DOI:
10.1039/D1TC05027C
(Paper)
J. Mater. Chem. C, 2022,
10, 4886-4893
A deep blue thermally activated delayed fluorescence emitter: balance between charge transfer and color purity†
Received
19th October 2021
, Accepted 31st January 2022
First published on 31st January 2022
Abstract
An efficient blue organic light-emitting diode (OLED) device with high color purity is a challenging issue with technological relevance. Herein, we demonstrated the role of the donor substitution position in a thermally activated delayed fluorescence (TADF) emitter to achieve deep blue emission with improved color purity without reducing the device performance. A novel (4-(3,6-di-tert-butyl-9H-carbazol-9-yl)phenyl)(6-(3,6-di-tert-butyl-9H-carbazol-9-yl)pyridin-3-yl)methanone (3BPy-pDTC) was synthesized, where two tert-butyl carbazolyl (DTC) donors linearly connected at the para position of the benzoyl pyridine (3BPy) acceptor core. The 3BPy-pDTC emitter shows a low ΔEST value of 0.19 eV with a subsequent overlap between holes and electrons which leads to a high photoluminescence quantum yield (PLQY) of 93%. Besides, this emitter exhibits narrow emission spectra with a full-width at half maximum (FWHM) of 58 nm. The OLED device using the 3BPy-pDTC dopant provided a high external quantum efficiency (EQE) of 25% with deep blue emission at λmax of 458 nm. 3BPy-pDTC shows color purity in deep blue emission as compared to its meta substituted counterpart (3BPy-mDTC) due to the locked geometry via intramolecular H-bonding and weak excited state charge transfer (CT) interactions. The current study paves the way for developing deep-blue TADF emitters with high efficiency and color purity.
1. Introduction
Metal-free organic emitters with an electron-donating (D) unit connected via a π-linker to an electron-accepting (A) unit emerged as important materials for optoelectronic devices.1–4 The optical and electrochemical properties of donor–acceptor (D–A) molecules can be tuned by controlling the excited-state intramolecular charge transfer (ICT).2,5–9 The excited-state CT proceeds with drastic conformational changes, mainly the rotation at the D–A bond leading to the formation of a twisted intramolecular charge transfer (TICT) state.6,10 Consequently, spectral broadening, large Stokes shifts, and lowering of the bandgap are observed in CT-based organic emitters.2,11 However, achieving high color purity in the devices is one of the critical issues due to spectral broadening.12–14 The broadening in emission (i.e. full width at half maximum, FWHM) can be tuned by monitoring the CT interactions in D–A-based organic emitters.15
Recently, D–A based chromophores have attracted much attention due to a lower singlet (S1)–triplet (T1) energy gap (ΔEST), which facilitates the photon upconversion from the low-lying T1 to the S1 excited-state. This leads to delayed fluorescence which is commonly known as thermally activated delayed fluorescence (TADF).1,3,16–22 Therefore, 100% internal quantum efficiency (IQE) of OLED devices can be achieved based on these metal-free TADF emitters. Hence, highly efficient, lightweight, flexible, and environment-friendly OLED devices are achievable without the use of noble metals such as Ir and Pt.23–28 Amid several recent reports, the development of blue TADF emitters with high color purity and high efficiency remains a challenge.12,29–32
The decoupling of the highest occupied molecular orbital (HOMO) and the lowest unoccupied molecular orbital (LUMO) is important to reduce the ΔEST. Hence, several D–A-based TADF emitters with twisted molecular geometry were designed to separate the HOMO and LUMO and obtain a low ΔEST to promote exciton upconversion from T1 to the S1 excited state.18,19 However, a more twisted structure results in the broadening of emission spectra, generally a FWHM of over 100 nm, which directly impacts the color purity of the devices.33 Conversely, a complete planar structure gives a narrow emission but it shows poor device performance due to large ΔEST values. In order to achieve high device performance with high color purity, the above trade-off needs to be solved. Therefore, an optimum molecular design strategy is required to balance the low ΔEST for efficient photon upconversion and a narrow FWHM in emission for color purity.
Recently, a multi-resonance TADF (MR-TADF) emitter was found to be an ideal molecular design for OLEDs due to its small ΔEST, high PLQY, and narrow emission.34–37 However, nearly all MR-TADF emitters are based on boron/nitrogen, which limits the molecular design flexibility and scope of these emitters.15,38 Further, most of the MR-TADF based emitters suffer from poor luminance.39,40 In order to overcome this limitation, conventional TADF emitters need to be designed and focused to achieve high color purity along with high device brightness as well as performance. Recently, several attempts were made to improve color purity and device performance using conventional TADF molecular design (Table S8, ESI†).
The enhanced molecular restriction from ortho (oPTC, FWHM = 97 nm) to meta (mPTC, FWHM = 86 nm) substitution of aryl groups in the phenoxazine–benzonitrile dyad led to better color purity as reported by Zhang and coworkers.41 Cheng and coworkers developed two isomeric TADF emitters and realised improved color purity with device performance for one isomer over the other. However, the emission maximum was in the sky-blue region and CIEy was over 0.18, and hence the emission spectrum needs to be blue-shifted to bring down the CIEy value below 0.15 for practical applications.3 Further, Kieffer and coworkers demonstrated that balanced CT interactions leading to hole/electron (h/e) overlap represent the key parameter for reducing the FWHM and color purity.42 The donor substitution at the meta and para positions to the pyridoquinoxaline (PQ) acceptor core significantly affects the CT interactions and optical properties demonstrated by Patra and coworkers.43
Very few attempts were made to design a conventional TADF emitter with a narrow emission bandwidth in the blue region.44 However, a unified molecular design strategy to achieve high efficiency with color purity in the deep blue region is not clearly represented. Our group developed 3BPy-mDTC as the TADF emitter in the cyan/sky-blue region by substituting donors at the meta position of the 3BPy acceptor core.45 Due to a strong donor–acceptor CT interaction and decoupled HOMO–LUMO, a broad TADF emission was observed in the sky blue region (λmax ∼475 nm) with an EQE of 24.6%. Here, we have designed and synthesised a 3BPy-pDTC TADF emitter. This newly synthesised para-substituted donor at the 3BPy acceptor core (3BPy-pDTC) resulted in a narrow emission (FWHM ∼58 nm) in the deep blue region (λmax = 458 nm) with a very high EQE of 25%. This is due to the balance between the low ΔEST and CT interactions. This suggests that fine-tuning of molecular design is very important to improve the color purity and achieve deep blue emission. This study demonstrated a unique strategy to obtain deep blue emission with improved color purity and retain the high electroluminescence device performance (Scheme 1).
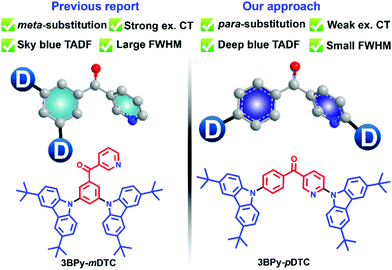 |
| Scheme 1 Molecular design strategy of a blue TADF emitter: a comparative account of the intrinsic ICT interactions of meta and para substituted donors on the 3BPy acceptor core. The molecular structures of meta-substituted 3BPy-mDTC and para-substituted 3BPy-pDTC with DTC as the donor units are shown. | |
2. Results and discussion
2.1 Design strategy and synthesis
An earlier report for the meta substituted donors on the 3BPy acceptor core exhibited strong excited state charge transfer (CT) interactions.45 As a result, sky blue (λem = 476 nm) TADF emission was observed. To develop deep blue emission with high device efficiency, we tuned the excited state CT interactions by synthesizing its positional isomer, consisting of the same terminal DTC as the donor group substituted to the para positions of the central phenyl ring 3BPy acceptor core. The donor substitution at the para positions of the 3BPy acceptor core via the cost-effective Ullmann cross-coupling reaction resulted in 3BPy-pDTC. The details of the synthetic procedure are given in the supplementary information (Section 2, Scheme S1, ESI†). 3BPy-pDTC was purified by temperature gradient high vacuum sublimation and characterized by NMR spectroscopy, high-resolution mass spectrometry and single crystal X-ray diffraction (SCXRD) analysis (Scheme S1 and Fig. S16, S17, ESI†).
2.2 DFT calculation
A time-dependent density functional theory (TDDFT) calculation was carried out using the Gaussian 09W program package. The optimized molecular structures of the two compounds are shown in Fig. 1. 3BPy-pDTC shows an almost planar structure (D–A–D dihedrals, 35° and 49°, respectively) with an energy gap of 3.37 eV, whereas 3BPy-mDTC shows a more twisted structure (50° and 57°) with a lowered energy gap of 3.18 eV. Therefore, 3BPy-pDTC is expected to show deep blue emission in contrast to 3BPy-mDTC, which is a sky-blue emitter.45 HOMO and LUMO distributions are localized on the donor (DTC) and acceptor (3BPy), respectively, for both emitters. Notably, 3BPy-pDTC shows separation between the HOMO and LUMO with a very large overlap, which leads to a large f for 3BPy-pDTC (f = 0.481), and a large f is important for improving the radiative decay channel and maintain a high PLQY. In contrast to 3BPy-mDTC, here the acceptor pyridine and phenyl π-spacer units are involved in both HOMO and LUMO distributions. The main transitions and oscillator strengths of both emitters are listed in Table S1 (ESI†). The excited state hole and electron distributions for S1 and T1 states were analyzed using Multiwfn software.46 The electrons of both S1 and T1 are distributed mainly in the acceptor core (Fig. S1 and Table S2, ESI†). In comparison, the hole for S1 is predominantly distributed over the donor carbazole units and extended to the phenyl π-spacer. However, the hole for T1 is distributed throughout the molecule (Fig. S1a, ESI†).26
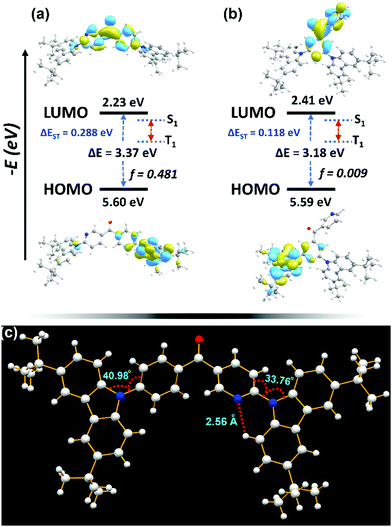 |
| Fig. 1 DFT calculated electron density distributions of the highest occupied molecular orbital (HOMO) and the lowest unoccupied molecular orbital (LUMO) of (a) 3BPY-pDTC and (b) 3BPy-mDTC at the B3LYP/6-311G(d,p) level. (c) The molecular structure and packing diagram of 3BPy-pDTC obtained from single crystal X-ray diffraction (SCXRD) analysis displaying the intramolecular H-bonding interaction and the donor–acceptor dihedral angles. | |
The crystal structure of 3BPy-pDTC obtained from the SCXRD analysis indicates the H-bond formation between the pyridine nitrogen and adjacent H–CH of the donor carbazole unit (N⋯H–CH: 2.56 Å) locking the molecular geometry (Fig. 1c and Fig. S2, Table S3, ESI†). Additionally, low D–A dihedral angles of 33.76° and 40.98°, respectively (Fig. 1c), are observed due to a locked molecular structure. The DFT optimized geometry also indicates a similar trend of twisting angles (Fig. 1a). As a result, a prominent overlap/coupling between the holes and electrons for both S1 and T1 was observed. This facilitates the photon upconversion from the T1 to S1 state through reverse intersystem crossing (RISC). Comparatively, a smaller coupling of holes and electrons in S1 and T1 states was observed for 3BPy-mDTC (Fig. S1b, ESI†). This suggests that 3BPy-mDTC possesses stronger excited-state intramolecular charge transfer interactions as compared to 3BPy-pDTC.
2.3 Photophysical properties
The UV-Vis absorption and PL spectra of 3BPy-pDTC recorded in toluene solution are shown in Fig. 2a and Table 1. 3BPy-pDTC exhibits prominent absorption broad bands at λabs = 375 nm due to the intramolecular charge transfer (ICT) absorption linked with the electron transfer from the donor (tCz) to the acceptor group (BPy). Further, the absorption measurement of 3BPy-pDTC was carried out in solvents with varying polarity (Fig. S3, ESI†). There is no significant change of peak maxima and the shape of the absorption band with varying solvent polarity. The meta-substituted (3BPy-mDTC) isomer shows two absorption bands: the band at 350 nm is due to the π–π* transitions and the weak broad band at 382 nm is due to the weak ground state ICT from the donor to the acceptor (Fig. S4, ESI†).45 As a result, a high molar extinction coefficient was obtained for the CT band of 3BPy-pDTC (ε375nm = 55
560 M−1 cm−1) compared to 3BPy-mDTC (ε385nm = 3330 M−1 cm−1).
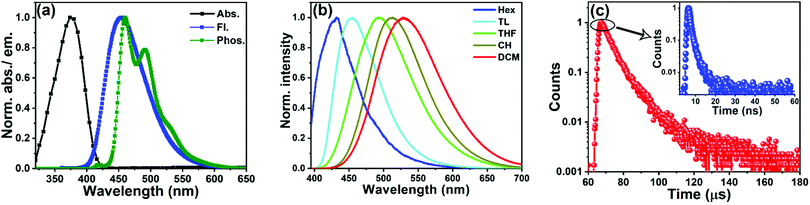 |
| Fig. 2 (a) Normalized absorption, fluorescence (λex = 405 nm) and phosphorescence (λex = 405 nm) spectra of 3BPy-pDTC in toluene (10 μM). (b) Normalized fluorescence (λex = 405 nm) spectra of 3BPy-pDTC in solvents of varying polarity. Hex: hexane (0.009), TL: toluene (0.099), THF: tetrahydrofuran (0.207), CH: chloroform (0.259) and DCM: dichloromethane (0.309). The solvent polarity parameter (ENT value) is mentioned in the parenthesis. (c) Emission decay profile (λex = 400 nm LED source) of 3BPy-pDTC in degassed toluene at room temperature showing delayed fluorescence; inset: decay (λex = 410 nm diode laser source) at the nanosecond timescale indicating prompt fluorescence. | |
Table 1 Photophysical properties of 3BPy-pDTC and 3BPy-mDTC
Compound |
λ
abs
(nm) |
λ
em
(nm) |
Egb (eV) |
HOMOc (eV) |
LUMOd (eV) |
ΔESTe (eV) |
PLQYf (Sol/film, %) |
krg (108 s−1) |
kISCh (108 s−1) |
kRISCi (105 s−1) |
knr,Sj (107 s−1) |
Measured in toluene (10 μM) at room temperature.
Estimated from the onset of the absorption spectrum.
Measured from the oxidation potential in 1 mM DCM solution by cyclic voltammetry.
Calculated from HOMO–Eg.
ΔEST = ES–ET, and ES and ET were obtained from the onset of fluorescence and phosphorescence spectra, respectively.
PL quantum yield evaluated in toluene using the reference dye and in the mCBP film with 7 wt% dopant using an integrating sphere.
Rate constant of fluorescence radiative decay (S1 → S0, kr = Φp/τp).
Rate constant of ISC (S1 → T1, kISC).
Rate constant of RISC (T1 → S1, kRISC).
Rate constant of nonradiative decay in the singlet state.
|
3BPy-pDTC
|
375 |
453 |
3.01 |
−5.86 |
−2.85 |
0.19 |
29/93 |
5.1 |
1.2 |
1.7 |
3.5 |
3BPy-mDTC
|
381 |
480 |
2.90 |
−5.61 |
−2.71 |
0.05 |
7/92 |
1.4 |
0.63 |
1.6 |
0.7 |
The steady-state emission of 3BPy-pDTC lies in the blue region and the peak is centred at 453 nm in toluene. To see the solvent effect on emission, steady-state emission spectra were measured with various polarities of the solvents (from hexane to dichloromethane (DCM)) (Fig. 2b and Fig. S5, S6, ESI†). In contrast to the absorption spectra, the emission spectra are red-shifted in polar solvents and the emission maximum changes from 434 nm in hexane to 530 nm in DCM (Fig. 2b). Both the emission maximum and the FWHM increased dramatically with solvent polarity (Table S4, ESI†). The significant solvatochromic shifts observed in the fluorescence spectra compared to no significant change in the absorption spectra suggest an excited state with a stronger ICT character and larger dipole moments relative to the ground state. The large Stokes shift and spectral broadening indicate a highly polarized ICT state.43 Excited-state CT characteristics were probed using the Lippert–Mataga (L–M) plot for both the compounds (Section 4.3 and Fig. S7, S8, Tables S4–S6, ESI†). A larger Stokes shift and transient dipole (μE–μG) of 3BPy-mDTC were observed as compared to 3BPy-pDTC (Table S6, ESI†). This indicates the relatively weaker excited state CT interactions in 3BPy-pDTC (Fig. S7, S8 and Table S6, ESI†).
The PLQY was measured in oxygen-free toluene solutions, the obtained quantum yield is 29% and the value decreases to 24% in the presence of oxygen (ESI,† Section 4.4). The decrease in the PLQY in the presence of oxygen supports that this emitter has TADF properties. The T1 state of this emitter is readily quenched by triplet ground state oxygen molecules, which leads to a decrease in RISC and subsequently the photoluminescence intensity. The PLQY of 7 wt% 3BPy-pDTC doped in the mCBP thin film is 93%, which was measured using an integrating sphere under a N2 atmosphere (Table 1). Surprisingly, the PLQY value of the thin film is much higher than that of the solution. This is because of the strong molecular packing in the crystalline or solid phase via intramolecular H-bonding (C–H⋯N, 2.56 Å, Fig. 1c) interactions locking the molecular geometry and restricted rotation at around the D–A bond (dihedral angles, 33.76° and 40.98°, Fig. 1c). As a result, we observed a relatively higher PLQY in the solid film of 3BPy-pDTC compared to the solution phase.
In order to know the nature of phosphorescence emission and ΔEST, the emission spectrum of 3BPy-pDTC was measured at liquid nitrogen temperature (77 K, Fig. 2a). At room temperature, considerable emission from only the S1 state was observed in the solution phase. On freezing the solution phase sample to 77 K, intramolecular rotations about the D–A bonds are mainly restricted due to the increased rigidity of the molecular environment. This, in turn, inhibits the nonradiative decay from T1 and thermally activated nonradiative decay channels and results in substantial phosphorescence emission from T1. The steady-state emission spectra, including the phosphorescence spectrum of 3BPy-pDTC, are shown in Fig. 2a. Structured emission was observed in the phosphorescence spectrum of 3BPy-pDTC (Fig. 2a), and this suggests that the phosphorescence emission from the LE state of T1. The singlet and triplet energy gaps were calculated from the onset of room temperature fluorescence (S1 = 3.01 eV) and low temperature (77 K) phosphorescence (T1 = 2.82 eV) spectra in toluene solvent. The calculated ΔEST is 0.19 eV (Fig. 2a, Table 1 and Table S2, ESI†). A small ΔEST = 0.19 eV indicates facile photon upconversion from T1 to S1 RISC, leading to TADF properties.
2.4 Time-resolved spectroscopy
To confirm the TADF properties of this emitter, the transient decay of 3BPy-pDTC was recorded in degassed toluene at room temperature (Fig. 2c and Table S7, ESI†). The decay profiles in the nanosecond (ns) and microsecond (μs) channels were found to be biexponential. The average lifetime (τavg) of emission decay in the nanosecond timescale is τavg = 1.5 ns, whereas the long-lived lifetime is τavg = 7.7 μs (Fig. 2c, Table 1 and Table S7, ESI†).47 The short component is assigned to be the prompt fluorescence (PF), and the long-lived species is due to the delayed fluorescence (DF), the photon upconversion from T1 to S1 and subsequent radiative decay from the S1 state. These short and long component radiative decays confirm that this emitter is in TADF nature (vide infra). To know the emission lifetime in the thin film, the emission spectra and transient decay of 3BPy-pDTC were also recorded in 10 wt% doped PMMA matrix (Fig. S9 and Table S7, ESI†). The biexponential decay of 3BPy-pDTC in the PMMA matrix with an average lifetime of τavg = 25.9 μs was observed (Fig. S9 and Table S7, ESI†).
2.5 Electrochemical and thermal properties
The electrochemical properties of 3BPy-pDTC were examined using cyclic voltammetry (CV), and the results are shown in Table 1 and Fig. S10 (ESI†). The HOMO energy level was calculated to be −5.86 eV from the oxidation potential, and the LUMO energy level was estimated to be −2.85 eV for 3BPy-pDTC. The lower LUMO level of 3BPy-pDTC compared to 3BPy-mDTC (2.71 eV) is due to a better accumulation of charges on the acceptor part. Similarly, the higher HOMO level in 3BPy-pDTC compared to 3BPy-mDTC (HOMO = −5.61 eV) is due to the electron-donating carbazole units linked in the para position of the acceptor core, leading to the distribution of the donor electron to the acceptor through the resonance (+R) effect.43 The electrochemical stability of 3BPy-pDTC was studied in DCM solution by recording the cyclic voltammograms up to 100 cycles at a positive potential.49,50 Fig. S11 (ESI†) shows no significant change of peak current and shape of CV traces up to 100 cycles indicating the high electrochemical stability of 3BPy-pDTC. The electrochemical properties are summarized in Table 1.
The thermal properties of 3BPy-pDTC were determined by thermogravimetric analysis (TGA) and differential scanning calorimetry (DSC) under a nitrogen atmosphere; the thermograms are shown in Fig. S12 (ESI†). 3BPy-pDTC shows high thermal stability with thermal decomposition temperatures over 413 °C. Glass transition temperatures were measured using differential scanning calorimetry (DSC). Interestingly, 3BPy-pDTC shows a higher glass transition temperature (Tg = 142 °C) as compared to 3BPy-mDTC (Tg = 101 °C) due to a more rigid structure. High Td and Tg values are important for enhanced morphological stability in the film (Fig. S12b, ESI†). Further, the photostability of the 3BPy-pDTC film in PMMA was tested by the continuous irradiation of a 150 W xenon lamp (λex = 375 nm) and the emission was recorded at λem = 455 nm for 2.5 h (Fig. S13, ESI†). The fluorescence intensity of the film remained around 85% up to 2.5 hours of continuous irradiation.
2.6 Electroluminescence performance
The multilayer OLED device was fabricated using 3BPy-pDTC as the emissive layer to examine the electroluminescence performance and a comparison study of its meta counterpart of 3BPy-mDTC was performed. The OLED devices were fabricated using these materials as dopants with the following structures: ITO/NPB (30 nm)/TAPC (20 nm)/mCBP (10 nm)/mCBP:3BPy-pDTC (7 wt%) or 3BPy-mDTC (7 wt%) (30 nm)/DPEPO (5 nm)/TmPyPb (60 nm)/LiF (0.8 nm)/Al (100 nm) (Fig. 3a and b).48,49 The electroluminescence (EL) properties of these two emitters are summarized in Fig. 3 and Table 2. The EQE–luminance curves reveal a maximum EQE (EQEmax) of 25.3% for the 3BPy-pDTC device and 24.6% for the 3BPy-mDTC (Fig. 3c and d). Although both emitters show comparable EQEs, the EL spectra show deep blue emission for 3BPy-pDTC at λem = 458 nm with very good color purity (FWHM = 58 nm) compared to sky blue emission for the 3BPy-mDTC device (λem = 476 nm) with a relatively broad emission band (FWHM = 66 nm) (Fig. 4 and Table 2). The CIE coordinates are (0.14, 0.13) for 3BPy-pDTC and (0.15, 0.28) for 3BPy-mDTC at 8 V. The 3BPy-pDTC based device shows a high brightness of 4568 cd m−2, which is much higher than that of the reported MR-TADF based OLED device (less than 1000 cd m−2).34 Interestingly, this device shows reduced roll-off as compared to the MR-TADF device and the device retains EQEs of 15.2% and 10.4% at 100 cd m−2 and 500 cd m−2, respectively. The reduced device efficiency roll-off of 3BPy-pDTC based devices is due to a small delayed fluorescence lifetime (τDF = 7.7 μs) and a fast kRISC rate (kRISC = 1.7 × 105 S−1). The maximum current and power efficiencies are 29.6 cd A−1 and 23.2 lm W−1, respectively, and these values are also higher than those of reported MR-TADF emitters.34,35 The transient electroluminescence decay of the 3BPy-pDTC device was recorded to confirm the long-lived species originating from efficient reverse intersystem crossing leading to TADF emission (Fig. 3e). Further, electroluminescence spectra were measured at various operating voltages (6–12 V, Fig. S14, ESI†). Both devices show similar emission spectra, and no residual emission is observed even at 12 V. This suggests that the device is very stable and recombination remains within the emissive layer at various operating voltages (Fig. 4, Fig. S14, S15, ESI† and Table 2).
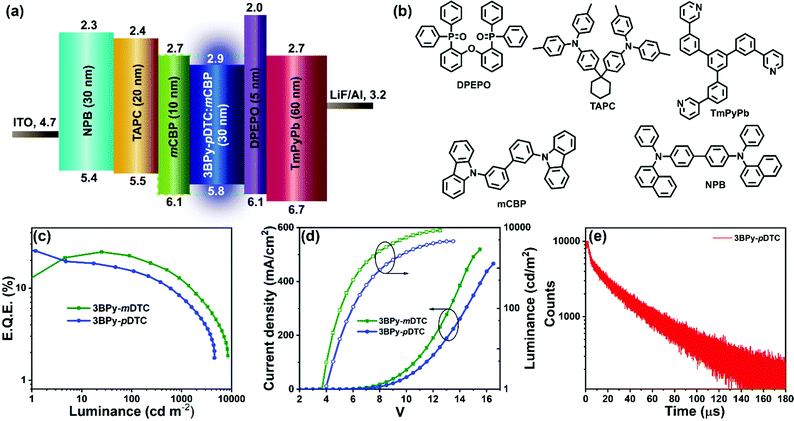 |
| Fig. 3 OLED device fabrication: (a) device architecture, (b) molecular structure of the materials used in the device, (c) luminance vs. EQE, (d) current density and luminance vs. driving voltage and (e) transient electroluminescence characteristics of the 3BPy-pDTC device. | |
Table 2 Electroluminescence performance of 3BPy-pDTC and 3BPy-mDTC
Devicea |
Turn-on voltageb (V) |
EQEc (%) |
Luminance, Cd m−2 d (V) |
CEe (Cd A−1) |
PEf (lm W−1) |
CIEg (8 V) |
ELh (λmax) (nm) |
FWHMi (nm) |
Device configurations: ITO/NPB (30 nm)/TAPC (20)/mCBP (10)/mCBP:3BPy-pDTC 7 wt%) or 3BPy-mDTC (7 wt%) (30 nm)/DPEPO (5 nm)/TmPyPb (60)/LiF (0.8 nm)/Al (100 nm).
Operating voltage at a brightness of 1 cd m−2.
Maximum external quantum efficiency (EQE).
Luminance.
CE = maximum current efficiency.
PE = maximum power efficiency.
Commission Internationale de l'éclairage (CIE) coordinates.
EL = electroluminescence (λmax = wavelength at which the EL spectrum has the highest intensity.
FWHM is the full width at half maximum of EL spectra.
|
3BPy-pDTC
|
3.8 |
25.3 (4.0) |
4568 (13.5) |
29.6 |
23.2 |
(0.14, 0.13) |
458 |
58 |
3BPy-mDTC
|
3.6 |
24.6 (4.5) |
8637 (12.5) |
48.4 |
38.0 |
(0.16, 0.29) |
477 |
66 |
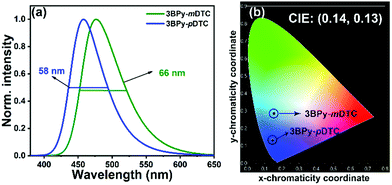 |
| Fig. 4 Blue OLED device based on TADF emitters: (a) electroluminescence (EL) spectra and (b) CIE chromaticity diagram of 3BPy-pDTC and 3BPy-mDTC depicting the color purity based on the narrow FWHM and CIE coordinates towards pure blue emission. | |
Despite the cross-conjugation in the meta substituted donors on the 3BPy acceptor, we observed stronger excited-state charge-transfer interactions in 3BPy-mDTC than the para-substituted isomer 3BPy-pDTC. Consequently, a smaller Stokes shift in all polar mediums along with a prominent hole–electron overlap was observed for 3BPy-pDTC. The intramolecular H-bonding (N⋯H–CH, 2.56 Å, Fig. 1c) plays a crucial role in locking the molecular geometry via restricted rotation in the D–A bond (dihedral angles, 33.76° and 40.98°, Fig. 1c) in para-substituted 3BPy-pDTC. As a result, the less D–A twist led to diminished excited state CT, and a higher ΔEST value for 3BPy-pDTC (0.19 eV) compared to 3BPy-mDTC (0.05 eV) was observed. However, both emitters exhibit almost similar electroluminescence performance with different λmax and spectral broadness. Thus, for better device performance with color purity, the overlap between holes and electrons is equally important, along with low ΔEST values for facile RISC. Further, the balanced CT interactions are also the key parameter to reduce the FWHM.42 Therefore, a combination of lowering excited state CT interactions through an appropriate donor position and the locking of molecular geometry resulted in deep blue TADF emission with a narrow bandwidth for para-substituted 3BPy-pDTC. In addition, the participation of the T2 state along with the T1 state in RISC leads to high device performance for both the emitters (Fig. S1, ESI†). The small energy gaps of T1–T2 (ΔET2–T1 = 0.054 eV) and S1–T2 (ΔES1–T2 = 0.234 eV) in 3BPy-pDTC facilitate the spin upconversion through reverse internal conversion (RIC) and RISC, respectively. The high-performance deep blue OLED device based on 3BPy-pDTC opens up a new avenue for further developing pure blue TADF emitters and provides scope for practical applications in flat panel displays.
3. Conclusions
In summary, two positional isomers (3BPy-pDTC and 3BPy-mDTC) were synthesised and the effects on the emission maximum, color purity, and device performance were studied. Both emitters show TADF properties with high PLQYs over 90%. However, the para substituted emitter (3BPy-pDTC) leads to higher color purity with deep-blue emission compared to the meta counterpart (3BPy-mDTC). The OLED device using the 3BPy-pDTC dopant provided a high EQE of 25% with narrow (FWHM = 58 nm) emission at λem = 458 nm and CIE coordinates of 0.14, 0.13. The para-substituted donor on the 3BPy core (3BPy-pDTC) shows a narrow emission band due to weak charge-transfer (CT) interactions and locked geometry via intramolecular H-bonding. The current study demonstrated new avenues for developing narrow, deep blue OLED devices with high efficiency for practical applications.
Conflicts of interest
There are no conflicts to declare.
Acknowledgements
The authors are grateful to Prof. Chien-Hong Cheng, National Tsing Hua University, Taiwan, for providing OLED device fabrication and characterization facilities. Bahadur thanks the Indian Institute of Science (IISc) Bangalore, India, for IoE C. V. Raman Fellowship. G. P. N. thanks the Council of Scientific & Industrial Research (CSIR) India for the Junior Research Fellowship (JRF). P. R. thanks the IISc for generous financial support and the Science & Engineering Research Board (SERB), India, for the SERB-Power Grant (SPG) (Grant no: SPG/2020/000107). We are thankful to Mr Rajendra Prasad and Mr Sarvesh of IISc for helping in recording the emission spectra and electrochemical studies, respectively.
Notes and references
- H. Uoyama, K. Goushi, K. Shizu, H. Nomura and C. Adachi, Nature, 2012, 492, 234–238 CrossRef CAS PubMed.
- S. Sasaki, G. P. C. Drummen and G. Konishi, J. Mater. Chem. C, 2016, 4, 2731–2743 RSC.
- P. Rajamalli, N. Senthilkumar, P. Y. Huang, C. C. Ren-Wu, H. W. Lin and C. H. Cheng, J. Am. Chem. Soc., 2017, 139, 10948–10951 CrossRef CAS PubMed.
- Y. Liu, C. Li, Z. Ren, S. Yan and M. R. Bryce, Nat. Rev. Mater., 2018, 3, 18020 CrossRef CAS.
- H. Tsujimoto, D. G. Ha, G. Markopoulos, H. S. Chae, M. A. Baldo and T. M. Swager, J. Am. Chem. Soc., 2017, 139, 4894–4900 CrossRef CAS PubMed.
- C. J. Chen, R. J. Huang, A. S. Batsanov, P. Pander, Y. T. Hsu, Z. G. Chi, F. B. Dias and M. R. Bryce, Angew. Chem., Int. Ed., 2018, 57, 16407–16411 CrossRef CAS PubMed.
- X. Tang, L. S. Cui, H. C. Li, A. J. Gillett, F. Auras, Y. K. Qu, C. Zhong, S. T. E. Jones, Z. Q. Jiang, R. H. Friend and L. S. Liao, Nat. Mater., 2020, 19, 1332–1338 CrossRef CAS PubMed.
- N. Altinolcek, A. Battal, C. N. Vardalli, M. Tavasli, H. A. Yu, W. J. Peveler and P. J. Skabara, J. Mol. Struct., 2021, 1239, 130494 CrossRef CAS.
- B. Sk, V. Thangaraji, N. Yadav, G. P. Nanda, S. Das, G. Parthasarathy, E. Zysman-Colman and P. Rajamalli, J. Mater. Chem. C, 2021, 9, 15583–15590 RSC.
- R. S. Nobuyasu, J. S. Ward, J. Gibson, B. A. Laidlaw, Z. Ren, P. Data, A. S. Batsanov, T. J. Penfold, M. R. Bryce and F. B. Dias, J. Mater. Chem. C, 2019, 7, 6672–6684 RSC.
- H. Tanaka, K. Shizu, H. Nakanotani and C. Adachi, Chem. Mater., 2013, 25, 3766–3771 CrossRef CAS.
- C.-Y. Chan, M. Tanaka, Y.-T. Lee, Y.-W. Wong, H. Nakanotani, T. Hatakeyama and C. Adachi, Nat. Photonics, 2021, 15, 203–207 CrossRef CAS.
- G. Hong, X. Gan, C. Leonhardt, Z. Zhang, J. Seibert, J. M. Busch and S. Braese, Adv. Mater., 2021, 33, 2005630 CrossRef CAS PubMed.
- S. Oda, W. Kumano, T. Hama, R. Kawasumi, K. Yoshiura and T. Hatakeyama, Angew. Chem., Int. Ed., 2021, 60, 2882–2886 CrossRef CAS PubMed.
- J.-M. Teng, Y.-F. Wang and C.-F. Chen, J. Mater. Chem. C, 2020, 8, 11340–11353 RSC.
- L. Sarala, R. Babu Yathirajula, P. Gopikrishna, E. Elaiyappillai, A. Bella, S. Sundar Manoharan, P. Krishnan Iyer and P. M. Johnson, J. Photochem. Photobiol., 2018, 365, 232–237 CrossRef.
- Y. Tao, K. Yuan, T. Chen, P. Xu, H. Li, R. Chen, C. Zheng, L. Zhang and W. Huang, Adv. Mater., 2014, 26, 7931–7958 CrossRef CAS PubMed.
- Y. Im, M. Kim, Y. J. Cho, J.-A. Seo, K. S. Yook and J. Y. Lee, Chem. Mater., 2017, 29, 1946–1963 CrossRef CAS.
- M. Y. Wong and E. Zysman-Colman, Adv. Mater., 2017, 29, 1605444 CrossRef PubMed.
- E. W. Evans, Y. Olivier, Y. Puttisong, W. K. Myers, T. J. H. Hele, S. M. Menke, T. H. Thomas, D. Credgington, D. Beljonne, R. H. Friend and N. C. Greenham, J. Phys. Chem. Lett., 2018, 9, 4053–4058 CrossRef CAS PubMed.
- D. Barman, R. Gogoi, K. Narang and P. K. Iyer, Front. Chem., 2020, 8, 483 CrossRef CAS PubMed.
- P. Rajamalli, D. Chen, S. M. Suresh, Y. Tsuchiya, C. Adachi and E. Zysman-Colman, Eur, J. Org. Chem., 2021, 2285–2293 CAS.
- K. Kawasumi, T. Wu, T. Y. Zhu, H. S. Chae, T. Van Voorhis, M. A. Baldo and T. M. Swager, J. Am. Chem. Soc., 2015, 137, 11908–11911 CrossRef CAS PubMed.
- W. Huang, M. Einzinger, T. Zhu, H. S. Chae, S. Jeon, S.-G. Ihn, M. Sim, S. Kim, M. Su, G. Teverovskiy, T. Wu, T. Van Voorhis, T. M. Swager, M. A. Baldo and S. L. Buchwald, Chem. Mater., 2018, 30, 1462–1466 CrossRef CAS.
- S. Jhulki, M. W. Cooper, S. Barlow and S. R. Marder, Mater. Chem. Front., 2019, 3, 1699–1721 RSC.
- L.-S. Cui, A. J. Gillett, S.-F. Zhang, H. Ye, Y. Liu, X.-K. Chen, Z.-S. Lin, E. W. Evans, W. K. Myers, T. K. Ronson, H. Nakanotani, S. Reineke, J.-L. Bredas, C. Adachi and R. H. Friend, Nat. Photonics, 2020, 14, 636–642 CrossRef CAS.
- R. Keruckiene, M. Guzauskas, L. Lapienyte, J. Simokaitiene, D. Volyniuk, J. Cameron, P. J. Skabara, G. Sini and J. V. Grazulevicius, J. Mater. Chem. C, 2020, 8, 14186–14195 RSC.
- H. Min, I. S. Park and T. Yasuda, Angew. Chem., Int. Ed., 2021, 60, 7643–7648 CrossRef CAS PubMed.
- Y. Wada, Y. Wakisaka and H. Kaji, ChemPhysChem, 2021, 22, 625–632 CrossRef CAS PubMed.
- T.-A. Lin, T. Chatterjee, W.-L. Tsai, W.-K. Lee, M.-J. Wu, M. Jiao, K.-C. Pan, C.-L. Yi, C.-L. Chung, K.-T. Wong and C.-C. Wu, Adv. Mater., 2016, 28, 6976–6983 CrossRef CAS PubMed.
- J.-H. Lee, C.-H. Chen, P.-H. Lee, H.-Y. Lin, M.-K. Leung, T.-L. Chiu and C.-F. Lin, J. Mater. Chem. C, 2019, 7, 5874–5888 RSC.
- G. Xia, C. Qu, Y. Zhu, J. Ye, K. Ye, Z. Zhang and Y. Wang, Angew. Chem., Int. Ed., 2021, 60, 9598–9603 CrossRef CAS PubMed.
-
J. R. Lakowicz, Principles of Fluorescence Spectroscopy, Springer, New York, 2006 Search PubMed.
- T. Hatakeyama, K. Nakajima, S. Nakatsuka, K. Kinoshita, T. Hatakeyama, K. Shiren, S. Nomura, J. Ni, Y. Ono and T. Ikuta, Adv. Mater., 2016, 28, 2777–2781 CrossRef CAS PubMed.
- Y. Zhang, D. Zhang, J. Wei, Z. Liu, Y. Lu and L. Duan, Angew. Chem., Int. Ed., 2019, 58, 16912–16917 CrossRef CAS PubMed.
- Y. Kondo, K. Yoshiura, S. Kitera, H. Nishi, S. Oda, H. Gotoh, Y. Sasada, M. Yanai and T. Hatakeyama, Nat. Photonics, 2019, 13, 678–682 CrossRef CAS.
- S. M. Suresh, E. Duda, D. Hall, Z. Yao, S. Bagnich, A. M. Z. Slawin, H. Bässler, D. Beljonne, M. Buck, Y. Olivier, A. Köhler and E. Zysman-Colman, J. Am. Chem. Soc., 2020, 142, 6588–6599 CrossRef CAS PubMed.
- J. M. Ha, S. H. Hur, A. Pathak, J.-E. Jeong and H. Y. Woo, NPG Asia Mater., 2021, 13, 53 CrossRef CAS.
- S. Madayanad Suresh, D. Hall, D. Beljonne, Y. Olivier and E. Zysman-Colman, Adv. Funct. Mater., 2020, 30, 1908677 CrossRef CAS.
- F. Huang, K. Wang, Y.-Z. Shi, X.-C. Fan, X. Zhang, J. Yu, C.-S. Lee and X.-H. Zhang, ACS Appl. Mater. Interfaces, 2021, 13, 36089–36097 CrossRef CAS PubMed.
- D. Y. Chen, W. Liu, C. J. Zheng, K. Wang, F. Li, S. L. Tao, X. M. Ou and X. H. Zhang, ACS Appl. Mater. Interfaces, 2016, 8, 16791–16798 CrossRef CAS PubMed.
- R. Ansari, W. Shao, S. J. Yoon, J. Kim and J. Kieffer, ACS Appl. Mater. Interfaces, 2021, 13, 28529–28537 CrossRef CAS PubMed.
- B. Sk, S. Khodia and A. Patra, Chem. Commun., 2018, 54, 1786–1789 RSC.
- H. Lim, H. J. Cheon, S.-J. Woo, S.-K. Kwon, Y.-H. Kim and J.-J. Kim, Adv. Mater., 2020, 32, 2004083 CrossRef CAS PubMed.
- P. Rajamalli, V. Thangaraji, N. Senthilkumar, C.-C. Ren-Wu, H.-W. Lin and C.-H. Cheng, J. Mater. Chem. C, 2017, 5, 2919–2926 RSC.
- T. Lu and F. Chen, J. Comput. Chem., 2012, 33, 580–592 CrossRef CAS PubMed.
- B. Sk, S. Sharma, A. James, S. Kundu and A. Patra, J. Mater. Chem. C, 2020, 8, 12943–12950 RSC.
- J.-J. Lin, W.-S. Liao, H.-J. Huang, F.-I. Wu and C.-H. Cheng, Adv. Funct. Mater., 2008, 18, 485–491 CrossRef CAS.
- P. Rajamalli, N. Senthilkumar, P. Gandeepan, P.-Y. Huang, M.-J. Huang, C.-Z. Ren-Wu, C.-Y. Yang, M.-J. Chiu, L.-K. Chu, H.-W. Lin and C.-H. Cheng, J. Am. Chem. Soc., 2016, 138, 628–634 CrossRef CAS PubMed.
- B. Sk, M. Sarkar, K. Singh, A. Sengupta and A. Patra, Chem. Commun., 2021, 57, 13590–13593 RSC.
Footnote |
† Electronic supplementary information (ESI) available: General information, synthesis and characterization including NMR spectra, computational details, UV-Vis spectra, crystal structure, L–M plot, power and current efficiency vs. luminance. CCDC 2116276. For ESI and crystallographic data in CIF or other electronic format see DOI: 10.1039/d1tc05027c |
|
This journal is © The Royal Society of Chemistry 2022 |