DOI:
10.1039/D0EE02967J
(Perspective)
Energy Environ. Sci., 2021,
14, 293-301
Laboratory protocols for measuring and reporting the performance of luminescent solar concentrators†
Received
15th September 2020
, Accepted 25th November 2020
First published on 11th December 2020
Abstract
Luminescent solar concentrators (LSCs) show great potential for both broadening the spectral response of photovoltaic devices and facilitating their deployment in urban environments. However, the recent success of LSCs has brought to light severe deficiencies in reporting protocols: direct comparison between lab-scale LSCs is not possible due to inconsistencies in the experimental measurements and reporting of device performance. Here, we make the case for treatment of LSCs as photonic devices rather than photovoltaic cells and identify best practice guidelines for the measurement and reporting of LSC performance.
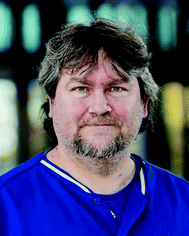
Michael G. Debije
| Michael holds a MS in High Energy Physics from Iowa State University, USA and a PhD in Biophysics from the University of Rochester, USA. He is currently an Assistant Professor at the Eindhoven University of Technology in the Department of Chemical Engineering and Chemistry where he has been researching novel materials and devices for application in the built environment with special interest in luminescent solar concentrators. |
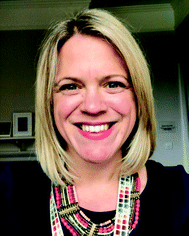
Rachel C. Evans
| Rachel is a Reader (Associate Professor) in Materials Chemistry at the University of Cambridge (UK). She obtained her MChem (2002) and PhD in Physical Chemistry (2007) from Swansea University (UK), and held an FCT research fellowship between the Universities of Coimbra and Aveiro (Portugal), followed by an Assistant, then Associate Professorship in the School of Chemistry at Trinity College Dublin (Ireland) from 2010–2017. Rachel's research is focused on the development of new photoactive materials and devices for harvesting solar and artificial light, solar-thermal energy storage and stimuli-responsive soft matter. |
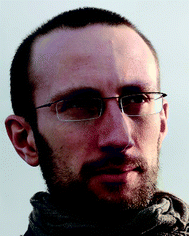
Gianmarco Griffini
| Gianmarco is currently Senior Assistant Professor (with tenure track) in Materials Science and Technology at Politecnico di Milano (Italy), where he received his MSc degree in Chemical Engineering in 2005 and his PhD degree in Materials Engineering in 2012. He has held visiting positions at University College London (UK), University of California at Berkeley (USA), and Universidad de Castilla-La Mancha (Spain). His main research interests are focused on: materials and devices for solar light harvesting, management and conversion; polymers for energy storage applications; stimuli-responsive (bio)polymeric materials for advanced manufacturing technologies. |
Broader context
The luminescent solar concentrator (LSC) has attracted worldwide attention as a possible complement to standard photovoltaic panels for the generation of electricity with a visually pleasing aspect in the urban setting. In addition to its use as a sunlight-converter in building integrated photovoltaic structures, this photonic device can find application in other areas, including distribution of colour-tuned light for enhancing plant growth in greenhouses, as a producer of fine chemicals in photomicroreactors, and in dynamic ‘smart’ windows for control of light entering room spaces. What is absent in LSC research is a universal standard for comparing device performance worldwide, which makes evaluation of different devices difficult or even impossible, as standard protocols applied for photovoltaic cell evaluation are inappropriate for use in LSC devices. In this work, we propose a standard protocol based on a series of robust and transparent measurements to fully characterise the LSC performance. These measurements are designed to be accessible to almost every laboratory working on LSCs. We believe that their adoption will bring consistency and eventually legitimacy to the field and be a major step towards commercialisation of these versatile devices.
|
Solar photovoltaic (PV) is the world's fastest-growing renewable energy technology, with higher performance cells and modules constantly being developed, forcing increasingly cost-competitive manufacturing. However, the PV efficiency remains intrinsically limited by its restricted spectral response, which allows only a fraction of the solar spectrum to be effectively harvested. In addition, deployment in buildings as integrated PV (BIPV) is confined primarily to rooftops, due to reduced cell efficiencies in diffuse sunlight and aesthetic limitations.
Luminescent solar concentrators (LSCs) offer a straightforward strategy to harvest, spectrally convert and optically concentrate solar photons. LSCs are most commonly composed of a luminescent species (luminophore) deployed as a coating on or as dopant of a transparent polymer or glass plate acting as a lightguide. The luminophores absorb both direct and indirect sunlight1 and re-emit it via a photoluminescence process (usually fluorescence) as photons of lower energies. The downshifted photons have energies that can be efficiently exploited by a mounted PV cell coupled to one or more edges or faces of the lightguide, thus harvesting the spectrally converted and optically concentrated photoluminescence photo transported there via total internal reflection (Fig. 1a).
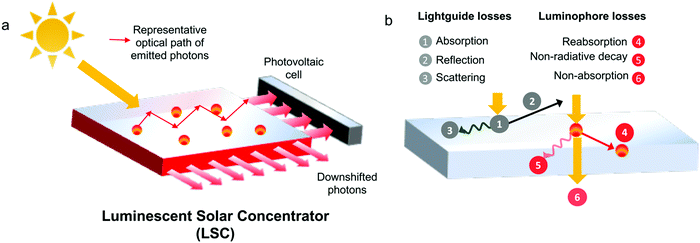 |
| Fig. 1 Device architecture and optical losses in LSCs. (a) In an LSC, luminophores (represented by red spheres) are either coated on or embedded within a lightguide plate. In an ideal device, downshifted photons are transported via total internal reflection to the edge(s) of the lightguide. PV cells may be attached to one or more edges or faces of the plate to harvest the spectrally converted and optically concentrated sunlight. (b) Different optical processes compete with absorption of sunlight, transport of emitted photons and their eventual absorption by the PV cell, leading to a decrease in optical performance of the LSC. | |
LSCs can be integrated directly with finished PVs, avoiding modifications to the electronic structure of the device and limiting physicochemical interactions with the photoactive layers. In addition, their aesthetic potential, colour and shape tunability, combined with their ability to enhance PV response to diffuse light, may provide new opportunities for innovative product design concepts deployable in a variety of market segments as diverse as architecture, agriculture, transportation and other infrastructures.2–4 These characteristics have propelled the rapid growth of the LSC field over the past decade,5 assisting the ongoing transition from small-scale prototypes6–8 to early industrial-scale demonstrations3,9 and start-up companies10 for the commercialisation of LSC-based products. Given the versatility in device structure, the absence of visible electrical interconnections and the working mechanism based purely on optical processes, LSCs are particularly suitable for urban integration, where one could advantageously deploy LSCs on any surface not optimal for standard PV usage, such as north-facing building facades and windows, park benches, kiosk walls, canopies, bus or bike shelters and advertising signage.11–14 In particular, LSCs can play a pivotal role in the transition towards wider-scale urban deployment of BIPV,13 a market already projected to exceed US$ 36 billion by 2025 at a compound annual growth rate of ∼19%.15
However, the rapid resurgence of the LSC field has revealed deficiencies in reporting and measurement protocols. It is often impossible to make a direct comparison between laboratory-scale LSC studies since the experiments are neither performed nor described in a uniform manner, with variations in luminophores, lightguide materials, panel size, surface or edge modifications, and nature of PV attachment. Research groups working on LSCs tend to fall into two main camps in how they view LSCs, inevitably leading to the use of inconsistent metrics for their characterisation: LSCs are regarded either as a particular class of PV devices or as purely photonic systems.
In the first camp, the performance of LSCs is mostly evaluated using the same metrics already widely adopted by the PV community, relying on the solar-to-electrical power conversion efficiency as the fundamental figure of merit.16–22 This metric certainly becomes important when discussing the commercialisation and deployment of the LSC device into the built environment at the industrial level, as it provides a single, easy-to-understand number that may be used to advertise and sell devices, and gives system designers the means to make calculations as to the impact of the device in their architectures. However, it is much less useful in the research process at the laboratory scale. Specifically, with this approach the performance of the luminophores and lightguide is largely hidden, and device performance is often dominated by the nature of the PV attached to the edge(s), obscuring information about the quality of the luminescent lightguide, the most important part of the device. For example, comparing the performances of two of the LSC devices cited as higher efficiencies in the literature from Slooff et al.22 which used a 5 × 5 cm2 LSC and GaAs PVs, and the 10 × 10 cm2 LSC devices by Desmet et al.23 coupled to Si PVs: which lightguides were actually performing better? It is not possible to determine from the information provided in these reports.
The second camp, which we espouse, allows researchers to directly compare between LSCs employing different luminophores and architectures, and even LSCs employing additional components such as surface photonic layers or structural reflectors. In this approach, the performance of LSC is determined by the optical processes taking place in the device, with its efficiency being directly dependent on the effectiveness of light-harvesting, photon emission and transport through the lightguide, and being independent of either the nature of the light source or PV cell used.24–32
One thing the LSC community does agree on is that internationally recognised conventions for device measurement are urgently required to provide clarity regarding the performance and reproducibility of devices prepared in diverse laboratories. Here, we make the case for the treatment of LSCs as photonic devices and propose a set of best practice guidelines to ensure reliability, reproducibility and transparency of the presented laboratory results. Our hope is that this will at least lead to uniformity in one branch of the LSC field, with the eventual goal of developing international standards accepted by the entire community that will provide a realistic measure of the technology-readiness of a given device, and accelerate the pace of commercialisation of LSC technology.
LSCs as photonic devices
The intrinsic performance of an LSC is defined by the interplay of optical processes including the absorption of sunlight, the transport of emitted photons and (often) their eventual conversion by the PV cell (Fig. 1b). Lightguide losses include parasitic absorption and scattering at optical defects, surface reflection, and non-trapping of photoluminescence during transport. Luminophore losses comprise non-absorption of incident photons (high transmittance), reabsorption of emitted photons by neighbouring optical centres and non-radiative relaxation of the excited state leading to non-unity photoluminescence quantum yield (PLQY). Given the significance of photon-related loss pathways in the LSC, it is clear that the standard metrics designed to report the efficiency of PV devices (most notably the power conversion efficiency) can only provide a partial description of the performance of an LSC. Rather, parameters generally not considered in standard PV protocols, including optical characteristics of the luminescent layer, performance in indirect light, size-scaling factors and appearance, are often as important in determining the potential for deployment of the finished product. Additionally, the metrics described in this work can be seamlessly used to allow comparison between LSCs used in alternative applications including water splitting8,33 as well as non-PV-based applications, for example in daylighting,34 imagers35 or fine-chemical production.36 As a result, it is urgent that experimental approaches, metrics and associated terminology be introduced to clearly differentiate LSC devices from PV cells, as they have very different applications and functions.
Key performance metrics
A key parameter for comparing the quality of the light-guiding process, independent from the luminophore's absorption range and incident light spectrum, is the ratio of photons reaching the lightguide edge(s) to the number of luminophore-absorbed photons. This figure of merit gives direct insight into the efficiency of the photon-transport process within the LSC as an aggregate measure of luminophore emission performance (Stokes shift, PLQY) and lightguide quality. As such, it provides unbiased, comparative information on the effect on performance variations of re-absorption losses, parasitic absorption by the lightguide, internal scattering or escape-cone losses without being affected by the nature of the illumination source, thus being directly useful to researchers to improve the performance of their own devices.
Despite being widely adopted, in the literature this parameter is simultaneously referred to as optical quantum efficiency,29 internal quantum efficiency28 and external quantum efficiency,37,38 among others. To avoid ambiguity and promote direct comparison of results, we define this performance indicator unequivocally as the internal photon efficiency, ηint:
Experimentally,
ηint is measured by irradiating the top surface of the LSC with all edges uncovered, with white (often solar simulated) or monochromatic light and collecting edge-emitted photons with an integrating sphere attached to a photodiode detector, or similar device. This experimental configuration is accessible to most laboratories, but the considerable design flexibility of LSCs can lead to ambiguity in the reported values. To improve transparency in the obtained results, we recommend reporting the number of photons emitted from an entire single lightguide edge measured with an integrating sphere for devices shaped as regular polygons, as for these configurations the total photon flux exiting each LSC edge will be essentially equivalent. However, individual edge values or an aggregate total thereof must be reported for asymmetric structures
32 or for devices with a non-uniform surface coverage of luminescent species.
39 The total number of absorbed photons may be determined by convolution of the absorption spectrum of the lightguide measured on a standard spectrophotometer and the spectrum of the incident light source. Since photon transport losses scale with device dimension,
40 the effect of poor lightguide quality or device construction may be difficult to ascertain for smaller samples, and the true impact may only be obvious upon scale-up. However, for the purpose of evaluation of luminophore quality and potential device performance, measurement of
ηint will allow direct comparison to be made between lab-scale devices, independent of the overlap between the luminophore absorption and incident light spectra.
Together with ηint, the external photon efficiency, ηext, should also be reported, defined as
This figure of merit is complementary to
ηint as it simultaneously captures both the light-harvesting ability of the LSC and the optical losses occurring during the photon propagation process in the LSC waveguide. As such,
ηext is a relevant figure for assessing the potential of deploying the device as a whole, giving an aggregated description of different photonic processes occurring within the LSC. Analogous to
ηint, this parameter can be measured experimentally by illuminating the top surface of the LSC with all edges uncovered with a light source of given spectral distribution and collecting edge-emitted photons with a suitable detector/integrating sphere. To avoid misrepresentation of this parameter, measurement of
ηext requires accurate reporting of the illumination source, and of the wavelength range addressed. Some lamps generate emission spectra ideal (but artificially suited) for absorption by luminophores used in LSCs, while producing less photons at wavelengths disadvantageous to them. This “trick” enhances the apparent external efficiency, while remaining unrepresentative of true potential for the LSC under natural sunlight. Similarly, reporting efficiencies only over wavelength ranges accessible to the luminophore or up to wavelengths outside the absorption band of the dye results in dramatic differences, making direct performance comparisons based on
ηext impracticable. Therefore, the wavelength window for integration must be standardised, and light sources closely approximating the solar spectrum are desired for reporting
ηext. Since standard integrating spheres typically use calibrated silicon diode array detectors with cut-off limits of around 1100 nm, an integration range of 350–1000 nm for determining the total number of incident and edge-emitted photons is sufficiently realistic and allows fair comparison between all but the most exotic luminophores. Finally, the concentration factor,
C =
Gηext should be reported, where
G is defined as the ratio between LSC surface area and LSC edge area and is referred to as the geometric gain.
C may also be readily calculated from
ηext and the physical description of the lightguide, if not explicitly stated.
Despite being primarily photonic devices, LSCs are also often described in combination with PV cells to produce electricity from sunlight, whose performance evaluation provides a direct estimate of the electrical power exiting the LSC assembly with respect to the optical power hitting the top surface upon direct illumination. This parameter gives a quantification of the potential usefulness of a given LSC as an auxiliary device to PVs from a practical application perspective, providing arguments for the eventual commercial exploitation of a particular system. As such, its reporting is desirable when possible, but not essential. Similarly to ηint and ηext, discrepancies in the literature are also significant in both the definition and associated measurement conditions of this parameter, largely preventing direct comparisons between different studies. To avoid misunderstanding, we propose reporting this parameter as device efficiency, ηdev, defined unambiguously as:
When reporting values for
ηdev, one major source of misinterpretation of reported results is related to the type of PV cell employed in the LSC/PV composite system. As the power generated by the LSC device is heavily affected by the electrical characteristics of a given PV cell, the independent performance of the bare PV cells under identical illumination conditions used for LSC characterisation should be provided in the ESI, including the external quantum efficiency (EQE) spectrum where possible, as well as PV cell type and manufacturer, size and surface area. This information is critical to perform sensible comparisons between LSCs employing different PV cells. In addition, it is also important to report the LSC/PV cell interface, whether coupled with an index-matching agent,
28,38,41 mechanical attachment
42 or simply left as an air gap,
29 since these different attachment methods affect the fraction of light that can be extracted from the lightguide into the edge-coupled PV. To standardise the experimental routine used to measure
ηdev, conventional
J–
V curve tests should be carried out under controlled illumination (1 sun, AM 1.5G – accessible to almost all research laboratories working in the field) to ensure third-party reproducibility of the claimed results. To avoid overestimation of the performance, care must be taken to ensure that the PV cells themselves are not directly illuminated during the measurement, as also recently highlighted.
18
The metrics identified above (ηint, ηext, C, ηdev) can all be obtained through characterisation of the samples using standard laboratory apparatus and require no exotic setups and thus become a universal set of parameters to maintain uniformity of approach across laboratories worldwide (Fig. 2). Both thin-film luminescent layers applied to transparent lightguides and doped lightguide LSC devices can be evaluated and compared using the same approach, as the optics of the LSC are not affected by these different device architectures.
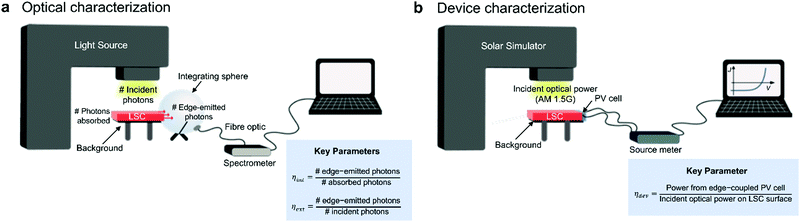 |
| Fig. 2 Experimental configurations used for the characterization of LSCs. (a) The optical characterisation of LSCs is carried out by irradiating the top surface of the LSC with a calibrated light source of known intensity and measuring the edge-emitted light during exposure by means of an integrating sphere coupled to a suitable detector. The key parameters extracted are the internal photon efficiency ηint and the external photon efficiency ηext, as defined in the light-blue box and discussed in the main text. (b) The device characterization of LSCs is performed by recording the J–V curve of edge-mounted solar cells of known spectral response under controlled illumination of the LSC top surface (typically, AM 1.5G simulated solar light). The key parameter extracted is the device efficiency ηdev, as defined in the light-blue box and discussed in the main text. | |
Standardisation of measurement conditions
All standard measurements on LSCs should be carried out using, at minimum, a black, absorbing background or a mounting preventing, as much as possible, reflections of unabsorbed light or luminophore-emitted light escaping the lightguide rear surface from re-entering the LSC. While the use of scattering or reflective backgrounds attached to or in the proximity of the vacant LSC edges to “boost” the measured efficiency is widespread and can be employed in commercial application of the devices, it is our opinion that this is counterproductive when trying to evaluate the intrinsic lightguide performance. Indeed, this practice enhances LSC performance due to the effect of light scattered or reflected by surrounding or background surfaces (including sample holders) which tend to scale nonlinearly with device size, overstating the performance of smaller devices.43 Even standard black materials have a degree of reflection, so care should be taken to select suitable backgrounds to minimise this effect. When measurements are conducted using a white scattering background, comparative results obtained using a black absorbing background on the same LSC under the same illumination conditions should be provided for reference. The experimental conditions during these measurements also need to be clearly reported to ensure reproducibility and correct evaluation of the performance: the distance of the scattering/background layer with respect to the bottom surface of the LSC; its specular and diffuse reflectance; its size relative to the LSC surface area; and the conditions of interaction with the lightguide (that is, whether the scattering layer is in optical contact with the lightguide or separated from it by an air gap).
The size and shape of the LSC should be clearly stated. There is considerable debate among the LSC community as to the proper size such an object should be for performance measurements. Ideally, samples of 10 × 10 cm2 or greater would be most desirable.44 However, as evidenced by the very few representative examples in the literature of fabrication and measurements of larger LSCs (in a recent review by Roncali,44 by our count only 16 of the 49 ‘modern’ devices listed were of a ‘larger’ size: of these, three needed to use outdoor measurements and roughly only five labs measured samples ≥100 cm2 used full-sample illumination in any controlled way), it is apparent the specialised equipment needed to manufacture, illuminate and measure emissions of such large samples is simply not available to the standard research laboratory. Alternative options, including measurements of long strips of lateral dimensions compatible with conventional integrating sphere ports (5 mm) or partial illumination of larger devices and collection of emitted photons at the centre of the edges (either with a PV cell or integrating sphere), are not reliable since photon output fluctuates considerably along the length of the LSC edge,45 meaning extrapolation can lead to an inaccurate description of the scaled device.
While we could insist that measurements on smaller samples should not be acceptable because of the potential of impact from scattering effects and the like, it would seem contrary to the goal of providing a universal, inclusive measurement protocol by excluding a significant fraction of researchers interested in participating in LSC research efforts, which would only, again, curtail progress in the field. Therefore, to allow maximal participation in LSC research, a reasonable standard size of LSC device for enabling direct comparison between independent laboratories is a 50 × 50 mm2 surface area device. This size is sufficient to allow reabsorption losses to assert themselves to enable a reliable evaluation of performance.43 At the same time, it is amenable for measurements on integrating spheres and illumination sources of sizes accessible to most laboratories operating in the field. Arguments that the use of larger devices would enable more complete evaluation of reabsorption and/or scattering losses in the LSC46 are necessarily accompanied by the requirement for larger, more expensive illumination sources and integrating spheres to perform these measurement, which as described earlier are quite rare and would prevent inclusiveness and accessibility of the technology. Generally, luminophore- and lightguide-related losses can be reliably assessed using dedicated optical experiments that do not necessarily require dedicated large-area testing equipment (see ESI†). In addition, simple observation of the edge emission spectra can identify the impact of scattering on device performance by the appearance of features in wavelength regions outside the normal emission spectrum of the luminophore. Similarly, reabsorption effects manifest themselves as bathochromic shifts in the emission peak position and as distortion in the shape of the emission spectrum. On balance, the first priority for the field should be to establish a standard measurement protocol accessible to the entire LSC community to maximise the potential for widespread adoption. The reporting of larger samples (even through additional optoelectronic characterisation based on the value of ηdev relative to the power conversion efficiency of the bare PV cell under direct illumination) or the extrapolation of their eventual performance via theoretical modelling are certainly welcome and should be included when possible, but optical measurements of a 50 × 50 mm2 device also need to be included in the results.
The thickness of the lightguide must also be reported, with 5 mm as a common and convenient practical standard and a minimum of 3 mm. LSCs in thin-film configurations should be deposited on 5 mm glass or other transparent substrate.47 While in general the use of 1–3 mm thick devices, for example, can be fairly well corrected for,29 inhomogeneous thickness along the entire slab becomes increasingly significant for thinner samples, which may lead to non-negligible effects on photon distribution.45 Moreover, in terms of end-use in electrical power generation, it is difficult to access commercially-available PV devices that can fit LSC edge thicknesses less than 3 mm. While examples of LSC plates thicker than 5 mm exist in the literature,3 they are relatively rare and often impractical.
The manufacturer and type of light source, detector and integrating sphere used should be stated in the Experimental section, along with spectral response limits for each component. Due to the variety of light sources used, we recommend that it should be standard practice to publish a measured spectrum of the light source over the relevant LSC spectrum used for the evaluation of ηext and ηdev in the ESI of a publication to ensure reproducibility of the results and allow sensible comparisons among studies.
A detailed checklist of the key metrics, measurements and experimental parameters that should be reported to enable more accurate assessment of the performance and reproducibility of lab-scale LSCs is provided in Table 1, while representative calculations for each parameter are reported in the ESI.†
Table 1 A practical checklist: key figures-of-merit to be considered when reporting the performance of an LSC
Parameter |
Equation |
Information obtained |
Experimental notes |
Internal photon efficiency |
|
Essential parameter – describes combined performance of luminophore and lightguide. |
Standard conditions: |
• LSC: 50 × 50 mm2 irradiation surface area; thickness – 5 mm is ideal. |
• State LSC shape and thickness. |
• Black absorbing background. |
• State light source. |
• Single edge output for symmetrical devices, individual output of all edges for asymmetric devices. |
|
External photon efficiency |
|
Essential parameter – describes performance of LSC under specific illumination conditions. The concentration factor, C, may be readily derived from the external photon efficiency and the lightguide dimensions. |
Standard conditions: |
• LSC: 50 × 50 mm2 irradiation surface area; thickness – 5 mm is ideal. |
• State LSC shape and thickness. |
• Black absorbing background. |
• State light source and provide spectrum. |
• State wavelength range (350–1000 nm as standard). |
• Single edge output for symmetrical devices, individual output of all edges for asymmetric devices. |
|
Device efficiency |
|
Optional parameter – describes performance of the integrated LSC-PV system under solar (simulated) irradiation. |
Standard conditions: |
• J–V characteristics under 1 sun, AM 1.5G illumination – avoid direct illumination of PV cell. |
• State LSC shape, size and thickness. |
• State active area, type and manufacturer of PV cell. |
• Black absorbing background. |
• Provide spectrum of light source and PV cell spectral response (EQE). |
Additional considerations
While often applied in similar situations, the LSC itself should not be considered simply as a PV device. This popular depiction, driven through the historical use of a single figure of merit ηdev to define its performance, can only partially describe its merits while overlooking the essence of the LSC being a photonic device, ultimately perpetuating confusion as to the role it could play in the urban landscape. In particular, the relative insensitivity to the nature of incident light distribution, the exciting possibility of their enhanced visual appeal, and finally, the additional stability challenges should also be considered in the evaluation of new LSCs. In order for application opportunities to be better identified, there are additional considerations of LSC performance that should be described. We will briefly introduce the discussion on each of these aspects here, leaving a more detailed analysis to future accounts.
Illumination conditions
As LSCs have been suggested as an appealing technology to accelerate the deployment of BIPV, it is relevant to report the performance of LSC devices also under illumination conditions mimicking those existing in urban areas. To this end, performance under diffuse light may be important as it can provide additional information on the potential benefits of LSCs over PV cells in some particular irradiation conditions.1 This can be easily accomplished in any laboratory by employing standard optical diffusers of different transmittance, placed between the light source (solar simulator) and the LSC during device testing. To aid comparison between laboratories, the optical characteristics of the diffuse filter used should be reported, including specular/diffuse reflectance spectra, transmittance spectrum and haze. This information is usually provided by the manufacturer but can also be extracted by simple optical measurements. Also, evaluating LSC operation under different incident light angles (not only orthogonal) can provide important insight into the performance of these devices in conditions close to real life operation, when tilting of the incident angle is experienced during daylight illumination.
Alternative emission characterization
While we espouse the use of side-mounted LSC devices to measure single-edge emissions, it is also possible to derive similar results by inserting the LSC lightguide within the integrating sphere.48 Placing the LSC device inside the sphere also allows access to additional parameters, including direct measurement of surface losses and the effect of indirect lighting. However, care must be taken in this case to properly mask the lightguide edges for top and bottom surface loss measurements, which are needed for accurate edge-emission determination. Similarly, single-edge measurements are less reliable when the LSC is placed within the integrating sphere as masking is required to isolate individual edges, which may result in additional contributions from light reflected from masked edges that would not be present in the ‘edge-mounted’ configuration.
Aesthetic appearance
Given the propensity of papers suggesting the deployment of LSC systems as optical windows28,29,37,38,49 and architectural elements,2,3 it becomes important to report the visual coloration of the devices, so that similarly-shaded devices can also be compared.50,51 In studies suggesting the use of these devices as window structures, reporting of the x,y colour coordinates (Commission Internationale de l’Eclairage, CIE) of transmitted light from a solar simulator is highly recommended. CIE x,y colour coordinates can be directly determined from the transmission or reflectance spectra and many spectrophotometer manufacturers now include this option in their software. Inclusion of a representative photograph is also encouraged to provide a visual impression of the device under daylight and other illumination conditions (where appropriate).
Stability and long-term performance
As recently highlighted for perovskite PV,52 we argue the lifetime of LSC solar devices should be a research topic in its own right and not simply an accessory to studies primarily focused on efficiency enhancement. Therefore, we give here only an account of best practices to be followed in this field, rather than providing definitive guidelines for setting up reliable protocols to assess the stability of LSC devices. Stability tests should employ stress factors relevant to the target application. While long-term durability tests (either accelerated or on-field) of several hundreds of hours are certainly desirable, the same considerations used for the analysis of the durability of traditional PV panels or concentrators (IEC 61215-1:2016, IEC 61215-2:2016, IEC 62108:2016) are not necessarily meaningful for all LSC devices, as these can often be designed for indoor use or deployed in diffuse light conditions. We suggest stability studies should provide at minimum a detailed account of the operational conditions, including light source (UV, white light, solar simulator, monochromatic with related emission spectrum and power density), environmental conditions (inert atmosphere vs. air, temperature, humidity) and exposure time. In any case, results obtained on timescales of just a few hours cannot be considered representative of real-life operation, and should be omitted from reports. Ideally, tests on both durability of component materials (e.g., spectroscopic analysis, physical and chemical characterization of both luminophore and lightguide material) and device performance (efficiency decay over aging time) should be reported so as to provide a sufficiently thorough account of the experimental evidence and to allow possible structure–property correlations that may enable predictive determination of system lifetime.
Sustainability
The end-of-life fate of manufactured devices is becoming increasingly relevant, and renewable energy devices are no different. There have only been a few studies related to the life cycle assessment of LSC devices to date,53,54 but commentary relating to the potential for recycling and reuse of LSC components would be a most welcome component in future publications.
Conclusions
LSC devices are on the brink of commercialisation. Here, we make the case for LSCs being characterised as photonic rather than photovoltaic devices and have outlined a series of parameters to be routinely measured and reported in any publication of LSC-based devices, prescribing dimensions and approaches that should be readily available in any well-equipped university laboratory. One thing is evident: without a standard set of measurements, the LSC will continue to flounder in relative obscurity, the devices unable to be compared between labs and, ultimately, unable to provide the confidence to the adopter that is essential to enable entry into a market dependent on guarantees for quality and ability to compare across technologies. It is hoped this article will encourage debate and eventual adoption of universal, standardised, and internationally recognised performance parameters that can be associated with individual devices, ultimately enabling certification of performance to the fledgling industry similar to those found in the PV panel industry.
Conflicts of interest
There are no conflicts to declare.
Acknowledgements
R. C. E. acknowledges funding from the European Research Council (ERC) under the European Union's Horizon 2020 research and innovation programme (Grant Agreement No. 818762 – SPECTRACON). G. G. acknowledges financial support from the Italian Ministry of University and Research (MIUR) through grant PRIN2017 – BOOSTER (protocol number 2017YXX8AZ).
References
- M. G. Debije and V. A. Rajkumar, Sol. Energy, 2015, 122, 334–340 CrossRef.
- A. Reinders, R. Kishore, L. Slooff and W. Eggink, Jpn. J. Appl. Phys., 2018, 57, 08RD10 CrossRef.
- M. Kanellis, M. M. de Jong, L. Slooff and M. G. Debije, Renewable Energy, 2017, 103, 647–652 CrossRef CAS.
- A. M. Detweiler, C. E. Mioni, K. L. Hellier, J. J. Allen, S. A. Carter, B. M. Bebout, E. E. Fleming, C. Corrado and L. E. Prufert-Bebout, Algal Res., 2015, 9, 170–177 CrossRef.
- R. Mazzaro and A. Vomiero, Adv. Energy Mater., 2018, 8, 1801903 CrossRef.
- W. van Sark, P. Moraitis, C. Aalberts, M. Drent, T. Grasso, Y. L’Ortije, M. Visschers, M. Westra, R. Plas and W. Planje, Sol. RRL, 2017, 1, 1600015 CrossRef.
- A. Kerrouche, D. A. Hardy, D. Ross and B. S. Richards, Sol. Energy Mater. Sol. Cells, 2014, 122, 99–106 CrossRef CAS.
- G. Panzeri, E. Tatsi, G. Griffini and L. Magagnin, ACS Appl. Energy Mater., 2020, 3, 1665–1671 CrossRef CAS.
- ENI, https://www.eni.com/en-IT/operations/concentrators-solar-luminescent.html, accessed 15th September 2020.
-
(a) Glass to power s.r.l., http://www.glasstopower.com/en/ (accessed 15th September 2020);
(b) Soliculture, http://www.soliculture.com/ (accessed 15th September 2020);
(c) Ubiquitous Energy, https://ubiquitous.energy (accessed 15th September 2020);
(d) Lusoco B.V. http://lusoco.com/ (accessed 15th September 2020);
(e) ClearVue PV, http://www.clearvuepv.com/, (accessed 15th September 2020);
(f) PHYSEE, http://physee.eu/, (accessed 15th September 2020);
(g) UbiQD, https://ubiqd.com/solar/ (accessed 15th September 2020).
- N. Aste, M. Buzzetti, C. Del Pero, R. Fusco, F. Leonforte and D. Testa, J. Cleaner Prod., 2019, 219, 35–45 CrossRef.
- J. ter Schiphorst, M. L. M. K. H. Y. K. Cheng, M. van der Heijden, R. L. Hageman, E. L. Bugg, T. J. L. Wagenaar and M. G. Debije, Energy Build., 2019, 207, 109625 CrossRef.
- F. Meinardi, F. Bruni and S. Brovelli, Nat. Rev. Mater., 2017, 2, 1–9 Search PubMed.
- R. Zarcone, M. Brocato, P. Bernardoni and D. Vincenzi, Energy Procedia, 2016, 91, 887–896 CrossRef CAS.
-
I. Grand View Research, Building Integrated Photovoltaic (BIPV) Market – Market Estimates & Trend Analysis from 2014 to 2025, 2019.
- X. Liu, B. Luo, J. Liu, D. Jing, D. Benetti and F. Rosei, J. Mater. Chem. A, 2020, 8, 1787–1798 RSC.
- C. Yang, M. Moemeni, M. Bates, W. Sheng, B. Borhan and R. R. Lunt, Adv. Opt. Mater., 2020, 8, 1901536 CrossRef CAS.
- C. Yang, D. Liu and R. R. Lunt, Joule, 2019, 3, 2871–2876 CrossRef.
- M. Rafiee, S. Chandra, H. Ahmed and S. J. McCormack, Opt. Mater., 2019, 91, 212–227 CrossRef CAS.
- B. Zhang, P. Zhao, L. J. Wilson, J. Subbiah, H. Yang, P. Mulvaney, D. J. Jones, K. P. Ghiggino and W. W. H. Wong, ACS Energy Lett., 2019, 4, 1839–1844 CrossRef CAS.
- K. Nikolaidou, S. Sarang, C. Hoffman, B. Mendewala, H. Ishihara, J. Q. Lu, B. Ilan, V. Tung and S. Ghosh, Adv. Opt. Mater., 2016, 4, 2126–2132 CrossRef CAS.
- L. H. Slooff, E. E. Bende, A. R. Burgers, T. Budel, M. Pravettoni, R. P. Kenny, E. D. Dunlop and A. Büchtemann, Phys. Status Solidi RRL, 2008, 2, 257–259 CrossRef CAS.
- L. Desmet, A. J. M. Ras, D. K. G. de Boer and M. G. Debije, Opt. Lett., 2012, 37, 3087 CrossRef CAS.
- M. G. Debije and P. P. C. Verbunt, Adv. Energy Mater., 2012, 2, 12–35 CrossRef CAS.
- B. McKenna and R. C. Evans, Adv. Mater., 2017, 29, 1606491 CrossRef.
- G. Griffini, Front. Mater., 2019, 6, 1–8 CrossRef.
- M. Portnoi, T. J. Macdonald, C. Sol, T. S. Robbins, T. Li, J. Schläfer, S. Guldin, I. P. Parkin and I. Papakonstantinou, Nano Energy, 2020, 70, 104507 CrossRef CAS.
- K. Wu, H. Li and V. I. Klimov, Nat. Photonics, 2018, 12, 105–110 CrossRef CAS.
- F. Meinardi, S. Ehrenberg, L. Dhamo, F. Carulli, M. Mauri, F. Bruni, R. Simonutti, U. Kortshagen and S. Brovelli, Nat. Photonics, 2017, 11, 177–185 CrossRef CAS.
- S. F. H. Correia, P. P. Lima, E. Pecoraro, S. J. L. Ribeiro, P. S. André, R. A. S. Ferreira and L. D. Carlos, Prog. Photovoltaics, 2016, 24, 1178–1193 CAS.
- R. W. MacQueen and T. W. Schmidt, J. Phys. Chem. Lett., 2013, 4, 2874–2879 CrossRef CAS.
- P. P. C. Verbunt, A. Kaiser, K. Hermans, C. W. M. Bastiaansen, D. J. Broer and M. G. Debije, Adv. Funct. Mater., 2009, 19, 2714–2719 CrossRef CAS.
- G. Liu, B. Sun, H. Li, Y. Wang and H. Zhao, J. Mater. Chem. A, 2019, 7, 18529–18537 RSC.
- A. A. Earp, G. B. Smith, J. Franklin and P. Swift, Sol. Energy Mater. Sol. Cells, 2004, 84, 411–426 CrossRef CAS.
- A. Koppelhuber, S. Fanello, C. Birklbauer, D. Schedl, S. Izadi and O. Bimber, Opt. Express, 2014, 22, 29531 CrossRef.
- D. Cambié, J. Dobbelaar, P. Riente, J. Vanderspikken, C. Shen, P. H. Seeberger, K. Gilmore, M. G. Debije and T. Noël, Angew. Chem., Int. Ed., 2019, 58, 14374–14378 CrossRef.
- F. Meinardi, A. Colombo, K. A. Velizhanin, R. Simonutti, M. Lorenzon, L. Beverina, R. Viswanatha, V. I. Klimov and S. Brovelli, Nat. Photonics, 2014, 8, 392–399 CrossRef CAS.
- H. Li, K. Wu, J. Lim, H. Song and V. I. Klimov, Nat. Energy, 2016, 1, 1–9 Search PubMed.
- A. Renny, C. Yang, R. Anthony and R. R. Lunt, J. Chem. Educ., 2018, 95, 1161–1166 CrossRef CAS.
- W. R. L. Thomas, J. M. Drake and M. L. Lesiecki, Appl. Opt., 1983, 22, 3440–3450 CrossRef CAS.
- A. Kaniyoor, B. McKenna, S. Comby and R. C. Evans, Adv. Opt. Mater., 2016, 4, 444–456 CrossRef CAS.
- D. Pintossi, A. Colombo, M. Levi, C. Dragonetti, S. Turri and G. Griffini, J. Mater. Chem. A, 2017, 5, 9067–9075 RSC.
- M. G. Debije, J.-P. Teunissen, M. J. Kastelijn, P. P. C. Verbunt and C. W. M. Bastiaansen, Sol. Energy Mater. Sol. Cells, 2009, 93, 1345–1350 CrossRef CAS.
- J. Roncali, Adv. Energy Mater., 2020, 2001907 CrossRef CAS.
- L. J. Brennan, F. Purcell-Milton, B. McKenna, T. M. Watson, Y. K. Gun’ko and R. C. Evans, J. Mater. Chem. A, 2018, 6, 2671–2680 RSC.
- R. A. S. Ferreira, S. F. H. Correia, A. Monguzzi, X. Liu and F. Meinardi, Mater. Today, 2020, 33, 105–121 CrossRef.
- M. R. Kulish, V. P. Kostylyov, A. V. Sachenko, I. O. Sokolovskyi, D. V. Khomenko and A. I. Shkrebtii, Semicond. Phys., Quantum Electron. Optoelectron., 2016, 19, 229–247 CrossRef CAS.
- C. Tummeltshammer, A. Taylor, A. J. Kenyon and I. Papakonstantinou, Sol. Energy Mater. Sol. Cells, 2016, 144, 40–47 CrossRef CAS.
- Y. Zhao and R. R. Lunt, Adv. Energy Mater., 2013, 3, 1143–1148 CrossRef CAS.
- C. Yang and R. R. Lunt, Adv. Opt. Mater., 2017, 5, 1600851 CrossRef.
- C. J. Traverse, R. Pandey, M. C. Barr and R. R. Lunt, Nat. Energy, 2017, 2, 849–860 CrossRef.
- H. J. Snaith and P. Hacke, Nat. Energy, 2018, 3, 459–465 CrossRef.
- A. R. Frias, S. F. H. Correia, M. Martins, S. P. M. Ventura, E. Pecoraro, S. J. L. Ribeiro, P. S. André, R. A. S. Ferreira, J. A. P. Coutinho and L. D. Carlos, Adv. Sustainable Syst., 2019, 3, 1800134 CrossRef.
- M. M. Lunardi, D. R. Needell, H. Bauser, M. Phelan, H. A. Atwater and R. Corkish, Energy, 2019, 181, 1–10 CrossRef CAS.
Footnote |
† Electronic supplementary information (ESI) available. See DOI: 10.1039/d0ee02967j |
|
This journal is © The Royal Society of Chemistry 2021 |