DOI:
10.1039/D0RA09088C
(Paper)
RSC Adv., 2020,
10, 44408-44429
A click-based modular approach to introduction of peroxides onto molecules and nanostructures†
Received
24th October 2020
, Accepted 20th November 2020
First published on 16th December 2020
Abstract
Copper-promoted azide/alkyne cycloadditions (CuAAC) are explored as a tool for modular introduction of peroxides onto molecules and nanomaterials. Dialkyl peroxide-substituted alkynes undergo Cu(I)-promoted reaction with azides in either organic or biphasic media to furnish peroxide-substituted 1,2,3-triazoles. Heterolytic fragmentation of the peroxide to an aldehyde, a side reaction that appears to be related to the formation of the triazole, can be suppressed by use of excess alkyne, the presence of triethylsilane, or by use of iodoalkyne substrates. Complementary reactions of simple alkynes with azido-substituted peroxides are much less efficient. Click reactions of alkynyl peroxyacetals are also reported; reductive fragmentation can be minimized by increasing the distance between the peroxyacetal and the alkyne. The strategy enables modular introduction of dialkyl peroxides and peroxyacetals onto gold nanoparticles, the first such process to be reported.
Introduction
Organic peroxides are capable of a wide range of reaction pathways,1 and have been applied as oxidants,2 radical initiators,3 pharmacophores,4 enzyme inhibitors,5 and synthons for electrophilic transfer of alkoxide.6 Peroxides are also of interest as intermediates and products of oxidative degradation and sources of reactive oxygen species.7 This rich chemistry contrasts with the limited number of reports describing reactivity or application of peroxides at surfaces.8 This discontinuity cannot be completely attributed to concerns for stability or safety. Peroxides are compatible with a surprising variety of synthetic transformations,9 and many peroxides may be safely employed by following established precautions.10 A more significant barrier to broader use of peroxides is the lack of effective methods for their modular introduction under mild conditions.
“Click chemistry”, a family of strategies based upon rapid and specific pairwise reactions of matched functional groups, has become an indispensable tool for modular approaches to molecules, supramolecules, and functionalized surfaces and nanoparticles.11 The most widely applied of these transformations, is the copper-assisted azide/alkyne cycloaddition (CuAAC), now a workhorse reaction for ligation and modification of molecules and supramolecules.12 CuAAC chemistry has been employed to generate derivatives of the peroxide antimalarial artemisinin,13 and we became interested in the potential for modular introduction of peroxides via click reactions. We now describe explorations of the scope of CuAAC chemistry reaction in terms of the nature of the peroxide group, whether the peroxide is linked to the alkyne or azide partner, the distance of the peroxide from the reaction center, and the influence of reaction conditions. The fragmentation of the peroxides observed in some of the click reactions of peroxyacetals demonstrates a potential for controlled generation of reactive oxygen species. We also demonstrate successful application of these ligations to modular introduction of peroxides on the surface of functionalized Au nanoparticles (AuNP), a nanomaterial platform widely applied for sensing, imaging, and biointeraction applications.14
Results
Substrates employed in CuAAC chemistry are illustrated in Fig. 1.
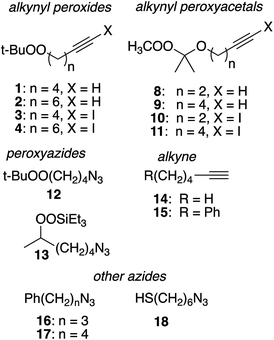 |
| Fig. 1 Substrates used for CuAAC chemistry studies. | |
Unfunctionalized peroxyalkynes 1 and 2 were easily assembled (Scheme 1) via Finkelstein exchange on the corresponding chloroalkyne,15 followed by nucleophilic displacement of the iodide with tert-butyl hydroperoxide in the presence of CsOH.16 Iodination of the terminal alkyne furnished the corresponding iodoalkynes (3 and 4) in good yield.17
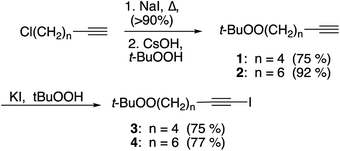 |
| Scheme 1 Synthesis of alkynyl and iodoalkynyl peroxides. | |
Preparation of alkynyl peroxyacetals was based upon trapping of an ozonolysis-derived carbonyl oxide with an alkynol (Scheme 2).18 This strategy, although rooted in the successful preparation of unsaturated hydroperoxyacetals from allyl alcohols,19 proved to be strongly dependent upon the structure of the alkynol. Ozonolysis of 2,3-dimethyl-2-butene in the presence of propargyl alcohol mainly afforded hydroperoxyperoxide 5a, which arises through reaction of the desired hydroperoxyacetal (5) with additional carbonyl oxide. The dimeric product predominates even in the presence of excess propargyl alcohol, presumably reflecting limited alcohol nucleophilicity.20 In contrast, the corresponding reactions of 3-butyn-1-ol and 5-hexyn-1-ol gave moderate and good yields of hydroperoxyacetals 6 and 7, respectively. The hydroperoxyacetals were readily methylated to afford peroxyacetals 8 and 9.21 Iodination of the peroxyacetals furnished iodoalkynyl acetal 10 and 11.
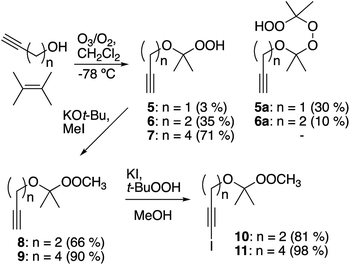 |
| Scheme 2 Synthesis of alkynyl- and iodoalkynyl peroxyacetals. | |
Preparation of peroxide-substituted azides is summarized in Scheme 3. Selective nucleophilic substitution of 1-bromo-4-chlorobutane afforded 1-chloro-4-azidobutane,22 which underwent CsOH-promoted substitution with tert-butyl hydroperoxide to generate azidoalkyl peroxide 12. The synthesis of a secondary peroxide/azide began with conversion of 5-hexene-2-ol to the corresponding iodide. Nucleophilic displacement with sodium azide was followed by Mukaiyama peroxidation to generate peroxy azide 13.23 As 12 and 13 both incorporate two energy-rich groups within a low molecular weight framework,24 initial syntheses were conducted on modest scales (∼1 mmol). Although both molecules proved to be stable to at least 100 °C (open chamber DSC, range limited by volatility), subsequent preparations were limited to ≤1 g and we avoided exposing either molecule to elevated temperatures.10 Preparations of 5-hexynyl benzene (15), azidoalkylbenzenes 16 and 17, and 6-azido hexanethiol (18) are detailed in the Experimental section.
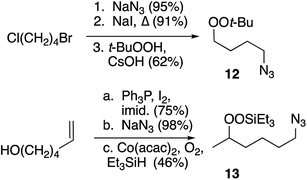 |
| Scheme 3 Synthesis of peroxy azides. | |
Copper(I) catalyzed click reaction with peroxy alkyne
Reaction of peroxyalkyne 1 with azidoalkylbenzenes 16 or 17 under either homogeneous (CuI and NEt3, in THF) or biphasic (CuSO4, sodium ascorbate, methylene chloride/water) conditions led to the rapid (≤15 min) appearance of triazoles 19 or 20 (Table 1);12,25 the presence of a peroxide was evident based upon a redox-sensitive TLC indicator.26 These initial reactions were stopped after brief reaction periods (1.5–3 h); longer reaction times led to consumption of starting material but also contamination with a nearly inseparable byproduct later established to be the tetrazole aldehyde (21, vide infra). A small amount of the corresponding 5-iodotriazole 20i, easily distinguished from 20 by the 13C signal for C5 (120.7 ppm for C–H vs. 78.0 ppm for C–I) was isolated from the reaction employing CuI.27
Table 1 Conditions for click chemistry with peroxy alkynes
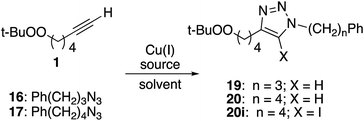
|
Azide |
Cu(I) source |
Solv. |
t (h) |
Conv. |
Prod. (yield, %) |
16 |
CuCl, NEt3 |
THF |
3 |
89% |
19 (25%) |
17 |
CuI, NEt3 |
THF |
3 |
80% |
20 (37%) |
20i (5%) |
17 |
CuSO4, Na ascorbate |
CH2Cl2/H2O |
1.5 |
85% |
20 (42%) |
The relationship between reaction time and aldehyde formation was examined using the reaction of 1 and 17 (Table 2). Ratios of peroxide products (20, 20i) vs. aldehyde (21) were established by NMR; samples of pure aldehyde were also isolated for characterization. The near absence of aldehyde for reactions conducted in the presence of excess alkyne (entries 6 and 9) would emerge as an important tool (vide infra).
Table 2 Influence of reaction time on byproduct formation
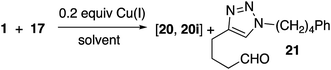
|
Entry |
Catalyst |
Solvent |
t (h) |
21 (yielda, %) |
Ratios assessed by 1H NMR. 1.2 equiv. 1. 0.4 equiv. Cu(I). |
1 |
CuI/Et3N |
THF |
1 |
6% |
2 |
〃 |
〃 |
3 |
8% |
3 |
〃 |
〃 |
8 |
13% |
4 |
〃 |
〃 |
18 |
20% |
5 |
〃 |
〃 |
27 |
30% |
6b |
〃 |
〃 |
3 |
<2% |
7 |
CuSO4/sodium ascorbate |
CH2Cl2/H2O |
4 |
20% |
8c |
〃 |
〃 |
4 |
38% |
9 |
〃 |
〃 |
18 |
57% |
10b |
〃 |
〃 |
1.5 |
5% |
11b |
〃 |
〃 |
4 |
22% |
Resubjecting isolated triazole 20 to either set of reaction conditions led to formation of aldehyde 21 (eqn (1)); in the presence of CuSO4/ascorbate, the aldehyde became the major product.
|
| (1) |
In an effort to better understand the factors leading to aldehyde formation, we probed the reactivity of triazole 20 towards reaction components (Table 3). No decomposition was observed in the presence of base or CuSO4 or ascorbate. However, treatment with CuI, CuI/triethylamine, or CuSO4/Na ascorbate generated significant amounts of aldehyde; the same was true for reaction with either Cu(I) source and azide. No aldehyde was observed in the presence of mixtures containing added alkyne (entries 5, 6, 11 and 12) which did not result in aldehyde formation. Interestingly, the 5-iodotriazole (20i) was unaffected by conditions which rapidly degraded 20 (entry 13).
Table 3 Stability of peroxide tetrazoles to reaction components
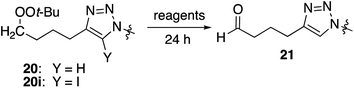
|
Entry |
S. mat |
Reagents |
Azide (equiv.) |
Alkyne (equiv.) |
21 (yielda, %) |
NMR yields; isolated yield in parentheses. |
1 |
20 |
Et3N |
— |
— |
Nr |
2 |
20 |
CuI |
— |
— |
95% |
3 |
20 |
CuI, Et3N |
— |
— |
70% |
4 |
20 |
〃 |
1.0 |
— |
50% |
5 |
20 |
〃 |
1.0 |
1.0 |
0% |
6 |
20 |
〃 |
— |
1.0 |
0% |
7 |
20 |
Na ascorbate |
— |
— |
0% |
8 |
20 |
CuSO4, Na ascorbate |
— |
— |
43% |
9 |
20 |
CuSO4 |
— |
— |
0% |
10 |
20 |
CuSO4, Na ascorbate |
1.0 |
— |
61% |
11 |
20 |
CuSO4, Na ascorbate |
1.0 |
1.0 |
0% |
12 |
20 |
CuSO4, Na ascorbate |
|
1.0 |
0% |
13 |
20i |
CuI, Et3N |
— |
— |
0% |
The results in Table 3 suggest that the formation of aldehyde may be accelerated in the presence of the neighboring triazole. Supporting this hypothesis, alkynyl peroxide 1 proved inert towards reaction with copper iodide in THF (eqn (2)).
|
| (2) |
Consistent with literature reports,28 click reactions proceeded more rapidly in the presence of tris[(1-benzyl-1H-1,2,3-triazol-4-yl)methyl]amine (TBTA), a Cu(I) ligand (Table 4). However, aldehyde formation continuing to be a problem and the overall yields of clicked peroxide were no greater, and sometimes less, than for reactions in the absence of the ligand. In contrast, performing the CuI or CuSO4/ascorbate-catalyzed reactions in the presence of excess alkyne completely suppressed aldehyde formation. This led to the hypothesis that the lack of byproduct might reflect the ability of free alkyne to protonate the C5-cuprated triazole intermediate in CuAAC chemistry.12,29 We also investigated this reaction in the presence of a silane, a functionality also known to quench organocopper species,30 and again observed suppression of aldehyde formation. Finally, replacement of the terminal alkyne with an iodide furnished only the 5-iodoalkyl peroxide; no aldehyde was observed. These reactions, although allowed to proceed for 18–24 h, were typically complete (TLC) within 30 minutes.
Table 4 Influence of additives
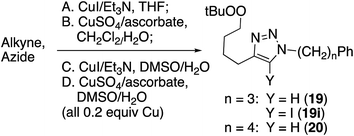
|
Alkyne |
Azide |
Cond. |
Additive (equiv.) |
t (h) |
Product (yield, %) |
1 |
17 |
A |
Alkyne 1 (1.2) |
18 |
20 (55%) |
1 |
17 |
B |
Alkyne 1 (1.2) |
18 |
20 (68%) |
1 |
17 |
C |
TBTA (1%) |
3 |
20 (20%) |
1 |
17 |
D |
TBTA (1%) |
3 |
20 (35%) |
1 |
16 |
A |
Et3SiH (1.2) |
24 |
19 (62%) |
3 |
16 |
A |
— |
24 |
19i (65%) |
Iodoalkynes display enhanced reactivity relative to terminal alkynes in CuAAC reactions,27 and this is also observed in the reactions of the peroxide-substrates. A competition between structurally analogous alkyne derivatives led to preferential consumption of the iodoalkynyl peroxide 3.
|
| (3) |
Proximity of peroxide and alkyne
Click reactions of peroxyoctyne 2 and the corresponding iodoalkyne (4) produced a good yield of peroxy triazoles 22/23 and iodotetrazoles 22i/23i (Table 5). For reactions employing CuI as the copper source, formation of the aldehyde byproducts 24/25 was minimal (2) or did not occur at all (4). In the case of reactions using CuSO4, aldehyde formation was significant for reactions involving alkyne 2 and minimal for iodoalkyne 4.
Table 5 CuAAC reaction of longer dialkyl peroxide
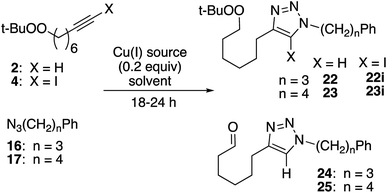
|
Alkyne, azide |
X |
Cu(I)a |
Conv. (%) |
Peroxide (yield, %) |
Aldehyde (yield, %) |
CuI/Et3N (0.2 equiv.), THF; CuSO4/sodium ascorbate, DCM/H2O (biphasic). Significant amount of unidentified polar byproduct. Et3SiH (1.9 equiv.) also present. |
2, 16 |
H |
CuI |
87.6 |
22 (68%) |
24 (11%) |
22i (6%) |
2, 16 |
H |
CuSO4 |
90 |
22 (41%) |
24 (37%) |
4, 16 |
I |
CuI |
94 |
22i (76%)b |
— |
2, 16 |
H |
CuIc |
∼10 |
22 (50%) |
— |
22i (3%) |
2, 17 |
H |
CuI |
87 |
23 (55%) |
25 (29%) |
23i (4%) |
2, 17 |
H |
CuSO4 |
89 |
23 (39%) |
25 (50%) |
Alkynyl peroxyacetals
Cu(I)-promoted reaction of the butynyl peroxyacetal (8) with azides 16 or 17 gave poor results (eqn (4)). In some cases, we observed successful click reaction to form inseparable mixtures of the desired peroxyacetal and the acetate ester derived from peroxyacetal fragmentation. No improvement was observed based upon the presence of excess alkyne, TBTA, or triethylsilane. In the presence of promoters derived from Cu(II), the reactions underwent a color change from dark green to light blue after 30–60 minutes. The color change could be temporarily reversed by the addition of more ascorbate but little or no clicked product was detected. Additionally, minimal progress was observed with the corresponding iodoalkynyl peroxyacetal (10). |
| (4) |
In contrast, the longer chain peroxyacetal (9) and the corresponding iodoalkyne (11) both reacted with azides in the presence of Cu(I) to give peroxy triazoles as the only major product (Table 6). Once again, no reaction was observed in the presence of a promoter derived from CuSO4/ascorbate.
Table 6 Click chemistry of hexynyl peroxyacetals
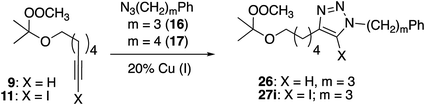
|
Alkyne |
Azide |
Cu(I) source |
t (h) |
Product (yielda, %) |
Isolated. |
9 |
17 |
CuI, Et3N |
3 |
26 (45%) |
9 |
17 |
CuSO4, ascorbate |
24 |
Nr |
11 |
16 |
CuI, Et3N |
18 |
27i (33%) |
A separate control experiment (eqn (5)) made obvious the stability of the alkynyl peroxyacetals towards Cu(I) in the absence of other reaction components.
|
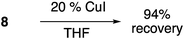 | (5) |
CuAAC reactions of peroxyalkyl azides
Reactions of simple alkynes with peroxide-containing azide 12 proceeded in modest yield using CuI/Et3N (Table 7); the corresponding reactions with CuSO4/ascorbate provided lower yields and required substantially longer reaction times. In both series, significant amounts of the peroxyazide were recovered. Although analysis of these reactions was complicated by the presence of inseparable byproducts, we could verify that little or no aldehyde was generated under these conditions.
Table 7 CuAAC chemistry with peroxyalkyl azides
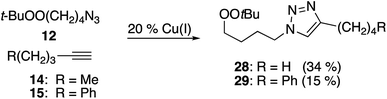
|
Alkyne |
Reagents |
t (h) |
Conv. (12) |
Product (yield, %) |
14 |
CuI/Et3N |
3 |
71% |
28 (34%) |
14 |
CuSO4/Na ascorbate |
24 |
80% |
28 (29%) |
15 |
CuI/Et3N |
3 |
76% |
29 (15%) |
15 |
CuSO4/Na ascorbate |
24 |
42% |
29 (≤5%) |
Secondary silyl peroxide 13 failed to give clicked products (eqn (6)); instead, producing a mixture of reduction and fragmentation products.1a,31 In the absence of alkyne, 13 was stable to both the CuI or CuSO4 reagent systems (not shown).
|
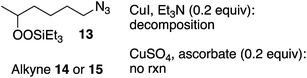 | (6) |
Influence of peroxides on the CuAAC reaction
The lower reactivity and yields observed with the peroxy-substituted azides led us to investigate whether the presence of a peroxide was exerting a general dampening influence on the CuAAC reaction. The reaction of 1-hexyne (14) and phenylalkyl azide 17, which went to completion in just a few minutes under our typical reaction conditions, failed to proceed at all in the presence of a stoichiometric amount of peroxide 13 (eqn (7)). |
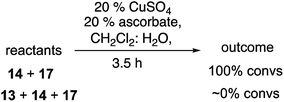 | (7) |
Nanoparticle functionalization
Having identified useful conditions for CuAAC reactions of alkynyl peroxides and peroxyacetals, we became interested in applying the chemistry to functionalization of nanoparticles. CuAAC-based functionalization of AuNPs, which exploits the high affinity of thiols for gold and the resulting ability to create azide-functionalized nanoparticles, has been widely applied to a variety of applications in both organic and aqueous media,11,14a,32–37 and we focused our attention on this system.
Azide-functionalized nanoparticles (N3Au) were prepared using a variant of a reported procedure in which Au nanoparticles (AuNP) are subsequently reacted with a passivating and then a functionalized thiol (Scheme 4).38 Addition of a slight excess of pentanethiol to a biphasic mixture of HAuCl3 and tetraoctylammonium bromide resulted in a white suspension which reacted with freshly prepared aqueous sodium borohydride to generate a dark and opaque suspension of pentanethiolate-functionalized nanoparticles (C5SAu). The partially passivated nanoparticles were isolated by centrifugation.11c TEM (Fig. 2) spectra confirmed the presence of 2–5 nm particles. The presence of the alkylthio chains was evident by the 1H signals for a terminal methyl group and the methylene groups at C3/C4 (ESI-Fig. 1†).39 XPS analysis revealed the surface region of the nanoparticles to consist of 50.1% Au, 43.9% C, and 6.0% S, suggesting that approximately 75% of the Au surface remained accessible for further functionalization (ESI-Fig. 2–4†).40 Repetition of the procedure using dodecanethiol, the preliminary passivating agent described in the original report, generated nanoparticles with similar properties (ESI-Fig. 5–8†).11c
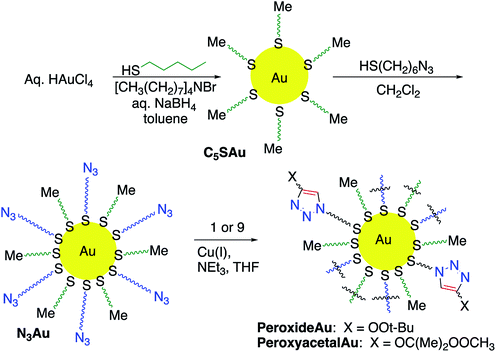 |
| Scheme 4 Synthesis of peroxide-functionalized nanoparticles. | |
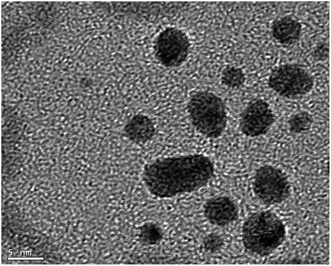 |
| Fig. 2 TEM of C5SAu. Scale bar = 5 nm. | |
Treatment of the C5SH-Au with excess 6-azidohexane thiol furnished the azide-modified nanoparticle (N3Au) which was isolated and purified using centrifugation and resuspension. The presence of the azide was evident from the strong IR absorbance at 2094 cm−1 (Fig. 3, compare panels a and b) and by the new 1H NMR signal at 3.31 ppm corresponding to CH2N3 (ESI-Fig. 9†); this signal was easily distinguished from signal for unbound azide (3.50 ppm), which was also observed in inadequately washed samples. The ∼2
:
3 ratio of the integrals for the signals corresponding to CH2N3 vs. CH2CH3 suggests a similar extent of coverage. XPS suggested a surface composition of 54.0% Au, 34.2% C, 8.5% S, and 3.3% (ESI-Fig. 10–13†).
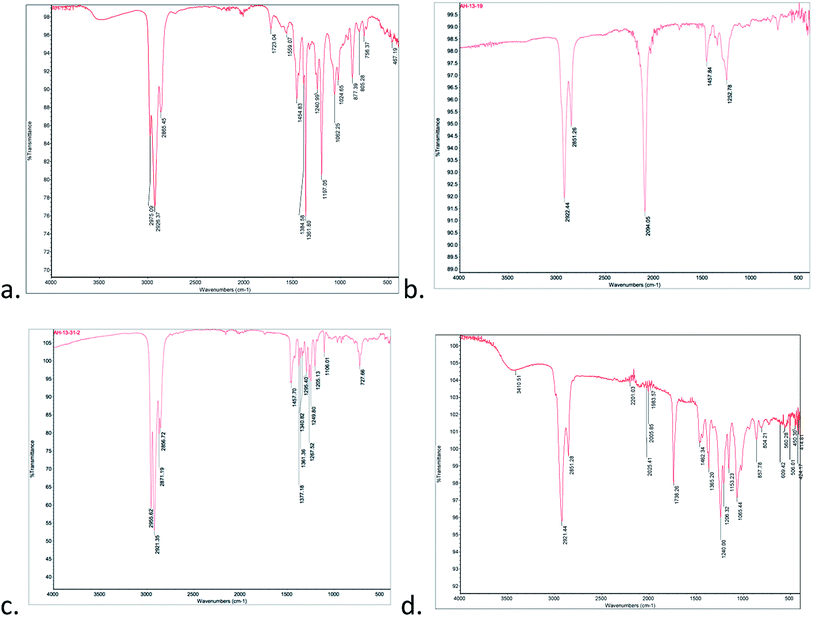 |
| Fig. 3 IR of nanoparticles: (a) C5SAu; (b) N3Au; (c) after click with peroxyalkyne 1; (d) after click with alkynyl peroxyacetal 9. | |
Peroxide functionalization of nanoparticles
Addition of triethylamine and cat. copper iodide into a THF solution containing peroxyalkyne 1 and N3Au led to the immediate appearance of a new peroxide-active spot on TLC (see Experimental details).26 After being quenched with aq. NH4Cl, the reaction was concentrated and the residue purified away from residual reactant by repeated resuspension (MeOH) and centrifugation. The azide stretch was now absent in the IR spectrum (Fig. 3d), which instead showed weak tetrazole-related stretches at 1723 cm−1 and 1559 cm−1 and only minimal absorptions associated with an aldehyde (1705–1710 cm−1). Evidence for the click reaction could also be seen in the 1H NMR (ESI-Fig. 14†) as the loss of the δ 3.31 signal corresponding to the CH2N3 and new signals at 1.27 (t-Bu) and 3.99 (OO). XPS established a product composition of 26.4% Au and 73.6% pentanethiol and peroxide triazole (ESI-Fig. 15–19†).
We also investigated click functionalization of azidoNP with alkynyl peroxy acetal 9. Consumption of the azide was obvious in the lack of the ∼2100 cm−1 IR stretch and the observation of a new C–H stretch at 2921 cm−1 (Fig. 3d). Although ester formation was equally obvious as an IR stretch at 1738 cm−1, the limited magnitude of this peak, combined with the comparison in the 1H NMR (ESI-Fig. 20†) of signals for the MeOO (3.54 ppm) and the gem-dimethyl groups of the acetal (1.57 ppm) vs. the peak group at 3.86 ppm (CH2O) of both acetal and ester, suggested the majority of the functionalized chain remained as peroxyacetal. XPS (ESI-Fig. 21–25†) suggested a surface composition of 20.0% Au and 80.0% pentanethiol and peroxyacetal triazole.
Discussion
Our results demonstrate that both peroxides and peroxyacetals can, under suitable conditions, serve as modular components for Cu-promoted CuAAC click reactions. The click reactions of the alkynyl peroxides and peroxyacetals proceed at rates in line with literature reports, with reactions times of 0.5–1 h for terminal alkynes and ≤0.75 h for 1-iodoalkynes.12,27 This is consistent with Zhu's observation that electron-poor alkynes are excellent substrates for CuAAC reactions.41 An “isomeric” set of click reactions involving simple alkynes and azidoalkyl peroxides is less efficient. The basis for inhibition of azide reactivity at a four-carbon distance from a peroxide remains unclear; however, we should note that we have observed a dampening influence of peroxides on SN2 reactions across a similar span.42
The use of CuI in organic solvents gives more consistent and often superior results compared to the use of CuSO4/ascorbate in biphasic media. The alkynyl peroxides and alkynyl peroxyacetal substrates, although stable towards Cu(I), are both prone to decomposition reactions in the presence of the reaction intermediates (Scheme 5). Interestingly, the efficiency of the reactions, the nature of the decomposition reactions, and the approaches to control of decomposition, are different for the two classes of substrates.
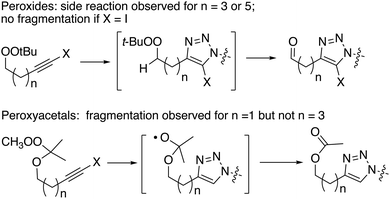 |
| Scheme 5 Overview of decomposition processes. | |
The dialkyl peroxides give modest to moderate yields under traditional CuAAC conditions due to the accumulation of a byproduct derived from fragmentation of the peroxide to an aldehyde. Aldehyde formation can be suppressed by use of an iodinated alkyne substrate, by performance of reactions in the presence of excess alkyne or added silane; under these conditions the yield of clicked peroxide is in the range of 62–68%. For the peroxyacetals, reactions proceed in lower yield, and, in the case of a shorter chain series (8), generate a significant amount of a byproduct in which the peroxyacetal has been fragmented to an acetoxy group. The formation of the ester is largely suppressed in a longer-chain peroxyacetal or for reactions of iodoalkynyl peroxyacetals. However, regardless of conditions, the yields obtained from peroxyacetals do not rival those obtained from the dialkyl peroxides.
An interpretation of our results in the context of the accepted mechanism of the CuAAC reaction (Scheme 6) suggests the decomposition reactions result from interaction of the peroxide or peroxyacetal with a heteroaryl organocopper intermediate generated at C5 of the developing triazole.12 Evidence in support of this hypothesis includes: the instability of the peroxyalkyne to any mixture containing Cu(I) and triazole; the lack of fragmentation observed for iodoalkynes; and the minimization of fragmentation the presence of excess alkyne or added triethylsilane, either of which would be expected to protonate the sp2-RCu.30 The clean formation of aldehyde, and the high barriers associated with electrochemical or chemical reduction of dialkyl peroxides,43 suggest the decomposition is a heterolytic process involving abstraction of the adjacent C–H.1a
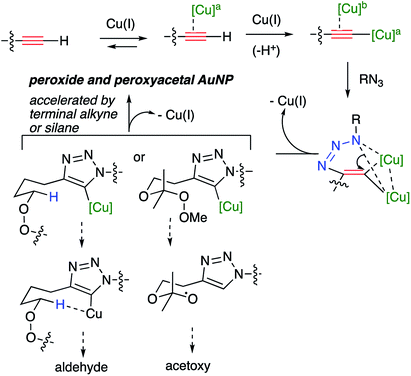 |
| Scheme 6 CuAAC mechanism and potential pathways for peroxide fragmentation. | |
The formation of an ester group (acetoxy) from fragmentations of the hydroperoxyacetals suggests the intermediacy of an alkoxy radical derived from cleavage of the peroxyacetal by an electron-donor; the likely reducing agent is the metalated triazole described above (Scheme 6).44 In contrast to the reactions of dialkyl peroxides, byproduct formation for the peroxyacetals is not suppressed by the presence of excess alkyne or added silane; it is suppressed by use of iodoalkynyl substrates or by increasing the peroxide/alkyne distance.
It is interesting to compare our results with reported CuAAC reactions of alkynyl derivatives of artemisinin-derived propargyl acetals and amides (Fig. 4); the approximate distance between the peroxide and the reaction site is similar in both systems.13 The heterolytic fragmentation we observed with dialkyl peroxides is not available in the artemisinin derivatives due to the lack of adjacent C–H groups. However, the fact that radical fragmentations are not observed in the artemisinin derivatives may indicate that the SET fragmentation requires close approach of the organocopper intermediates and the peroxyacetal, something that is possible in A and B and not possible in C or D.
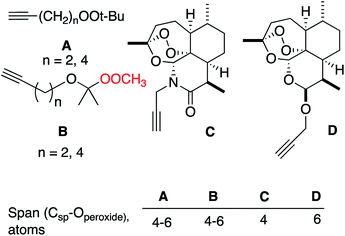 |
| Fig. 4 Comparison with artemisinin-derived “click” substrates. | |
CuAAC-based functionalization of AuNPs, which exploits the high affinity of thiols for gold has been widely applied to a variety of applications in both organic and aqueous media.11c,14b,c,32–37 At the outset of our studies, we were concerned by reports suggesting that CuAAC reactions on gold nanoparticles require longer reaction periods or use of microwave heating,45,46 and can be accompanied by nanoparticle aggregation and precipitation.45,47 Our results indicated that modular introduction of peroxides on nanoparticles via CuAAC suffers from no such limitations, and can be performed in less than eight hours and in the absence of microwave heating. Installation of dialkyl peroxides proceeds cleanly even in the absence of additives required in solution reactions, suggesting that the high local concentration of alkyne relative to the surface N3 groups suppresses aldehyde formation. Peroxyacetals, which are much more activated than dialkyl peroxides towards cleavage,6,43 also undergo successful click reactions on surfaces. However, in this case, the side reaction observed in solution phase chemistry continued to be observed in reactions on nanoparticles. This reaction, in contrast to some results on the extremely hindered peroxyacetal core of artemisinin, is suppressed by the use of a substrate containing a greater span between the peroxide and alkyne groups.
With the exception of functionalized SAMs displaying peroxides (via ozonolysis of alkene-terminated monolayers)8 or diacyl peroxides (via condensation of H2O2 with carboxylic acids),48,49 there are few examples of covalent introduction of peroxide on surfaces. Much of this is likely to relate to concerns about compatibility of the surface with the functionalization method; for example, ozone is known to attack the thiol/Au interface and is incompatible with many electron-rich groups.18b,50 Our work demonstrates that the CuAAC reaction can be used to install both dialkyl peroxides and the more reactive peroxyacetals on nanoparticle surfaces.
Conclusions
Our work, the first systematic investigation of the factors influencing the use of click reactions for installing organic peroxides, demonstrates that the copper-assisted click offers a practical approach for modular introduction of dialkyl peroxides and peroxyacetals. The best results are obtained with dialkyl peroxides; a side reaction involving heterolytic fragmentation of the peroxide can be easily circumvented by choice of substrate or additive. Although installation of more reactive peroxyacetals can be complicated by a radical fragmentation, this process can be minimized by use of a greater span between the peroxide and the reaction site.
Our work also provides first example of the use of click chemistry for modular introduction of organic peroxides on surfaces. Reactions are rapid, proceed to high conversion of nanoparticle surface functionality, and can be accomplished under mild conditions and without nanoparticle aggregation. Peroxide-functionalized surfaces could provide the basis for reactive or antimicrobial coatings,51 as well as a platform for investigating spatially-constrained interactions or reactions of peroxides or derived reactive oxygen species.52 Along these lines, the peroxyacetals investigated here, in addition to offering a source of alkoxy radicals, are known to be easily hydrolyzed to generate free hydroperoxides,16 while the simple t-alkyl peroxides, although nearly inert to simple reducing agents and Fe(II), are known to be activated photochemically52,53 or by iron/thiol complexes.43
Experimental procedures
General methods
All reagents and solvents were used as purchased except for CH2Cl2 (distilled from CaH2), DMF (vacuum distilled from CaH2), and THF (distilled from Na/Ph2CO). All nonaqueous reactions were conducted under an atmosphere of N2 in flame-dried glassware. Thin layer chromatography (TLC) was performed on 0.25 mm hard-layer silica plates. Developed plates were visualized by 254 nm UV lamp and/or by staining: 2.5% ammonium molybdate and 0.5% ceric sulfate in 10% aqueous sulfuric acid (general stain, after heating); 1% aq. potassium permanganate (alkynes); 1% N,N′-dimethyl-p-phenylenediamine in 1
:
20
:
100 acetic acid/water/methanol (specific for peroxides; dialkyl peroxides and peroxyacetals can be visualized as a reddish or reddish-green spot upon heating);26 or vanillin and sulfuric acid (3% each) in ethanol (general stain, after heating). Unless otherwise described, chromatography refers to silica flash chromatography.
NMR spectra were acquired in CDCl3 at 400 (1H) or 100 MHz (13C) unless otherwise noted. Chemical shifts are reported relative to residual chloroform (7.26 ppm, 1H; 77.36 ppm, 13C). 1H spectra are reported as chemical shift (multiplicity, J couplings in Hz, number of protons). IR spectra were recorded as neat films on a ZrSe crystal; selected absorbances are reported in cm−1. High resolution mass spectra (HRMS) were obtained at the Nebraska Center for Mass Spectrometry at UNL. XPS and TEM spectra were acquired in the Nebraska Center for Nanoscience and Materials on a Thermo Scientific K-Alpha X-ray photoelectron spectrometer using a monochromated Al Kα (1486.6 eV) X-ray source and a dual-beam flood source using a FEI Tecnai Osiris (scanning) transmission electron microscope.
CAUTION: Although we experienced no exotherms or explosions during the reported investigations, any work with peroxides of moderate or high active oxygen content, in particular any steps involving heating and/or concentration, should be conducted following standard precautions (adjusting scale to perceived hazard; concentration, and when necessary, reaction behind shields; some analysis of the thermal sensitivity of new products). Readers are directed towards a web-published overview of peroxide safety.10d
Synthesis of peroxyalkynes
6-(tert-Butylperoxy)-1-hexyne (1) was prepared by an adaptation of published procedures.54 To a solution of 6-chloro-1-hexyne (4.66 g, 40.0 mmol) in acetone (150 mL) was added sodium iodide (32.98 g, 220.0 mmol, 5.5 equiv.). The reaction was stirred under reflux for 18 hours. The cooled reaction was then diluted with water (50 mL) and extracted with hexanes (50 mL × 3). The combined organic layers were dried with Na2SO4 and the residue concentrated under reduced pressure to yield 7.5307 g (91%) of 6-iodo-1-hexyne as a yellow oil which was used without purification. Spectral details matched those previously reported.15,55 Rf = 0.42 (10% EA/Hex); 1H δ 3.20 (t, J = 8.0, 2H), 2.22 (td, J = 3.5, J = 9.3, 2H), 1.92 (t, J = 9.3, 1H), 1.63 (p, J = 6.4, 2H); 13C δ 83.7, 69.0, 32.3, 29.2, 17.5, 6.2.
To a solution of CsOH monohydrate (4.50 g, 30.0 mmol, 1.2 equiv.) in DMF (100 mL) at 0 °C was added dropwise TBHP as an ∼5.5 M solution in decane (6.81 mL, 37.5 mmol, 1.5 equiv.). The mixture was stirred for 30 min, whereupon 6-iodo-1-hexyne (5.20 g, 25.0 mmol) was added. The reaction was allowed to slowly warm to room temperature. After 5 h, the reaction was quenched with water (30 mL) and extracted with hexanes (30 mL × 3). The combined organic layers were dried with Na2SO4, concentrated under reduced pressure and the residue purified by column chromatography (5% EA/Hex) to yield 3.981 g (94%) of 6-(tert-butylperoxy)-1-hexyne as a colorless oil. Spectral details matched previous reports:54a Rf = 0.56 (10% EA/Hex) 1H δ 3.96 (t, J = 6.4, 2H), 2.22 (td, J = 7.0, J = 2.6, 2H), 1.94 (t, J = 2.6, 1H), 1.72 (m, 2H), 1.61 (m, 2H), 1.24 (s, 9H); 13C δ 84.3, 80.2, 74.5, 68.6, 27.2, 26.5, 25.4, 18.4; IR: 3314, 2924, 2119, 1362, 1198, 628; HRMS (ESI+, TOF) calcd for C10H18NaO2 [M + Na]+: 193.1204; found: 193.1205.
8-(tert-Butylperoxy)-1-octyne (2) was prepared by a similar procedure as described above. Reaction of 8-chloro-1-octyne (3.3914 g, 23.45 mmol) and sodium iodide (19.366 g, 129.2 mmol, 5.5 equiv.) furnish 5.0135 g (91%) of 8-iodo-1-octyne as a yellow oil which was used without further purification. Spectral details matched those previously reported:56 Rf: 0.43 (10% EA/Hex); 1H δ 3.19 (t, J = 7.0, 2H), 2.19 (td, J = 6.6, J = 2.2, 2H), 1.94 (t, J = 2.6, 1H), 1.83 (p, J = 6.7, 2H), 1.53 (m, 2H), 1.41–1.43 (m, 4H); 13C δ 84.5, 68.4, 33.5, 30.1, 28.3, 27.7, 18.4, 7.1.
By the same method as employed for synthesis of 1, reaction of the iodooctyne (3.8046 g, 16.50 mmol) with CsOH monohydrate (3.310 g, 22.1 mmol, 1.3 equiv.) and TBHP as an ∼5.5 M solution in decane (4.50 mL, 24.75 mmol, 1.5 equiv., ∼5.5 M solution in decane) in DMF, furnished 3.0118 g (92%) of 8-(tert-butylperoxy)-1-octyne (2) as a colorless oil: Rf: 0.55 (10% EA/Hex); 1H δ 3.93 (t, J = 6.6, 2H), 2.18 (td, J = 6.9, J = 2.5, 2H), 1.93 (t, J = 2.6, 1H), 1.50–1.64 (m, 4H), 1.35–1.46 (m, 4H), 1.24 (s, 9H); 13C δ 84.7, 80.2, 75.1, 68.3, 28.7, 28.5, 27.9, 26.4, 25.8, 18.4 IR: 3312, 2945 (s), 2956, 1456, 1360 (s), 1198 (s); HRMS (ESI+, TOF) calcd for C12H22NaO2 [M + Na]+: 221.1517; found: 221.1510.
1-Iodo-6-tert-butylperoxy hexyne (3) was prepared using an adaptation of a published procedure.17 To a solution of 6-(tert-butylperoxy)-1-hexyne (850 mg, 4.99 mmol) in MeOH (20 mL) was added KI (1.02 g, 6.13 mmol, 1.2 equiv.), followed by TBHP as an ∼5.5 M solution in decane (1.36 mL, 7.48 mmol, 1.2 equiv.). The reaction was stirred at room temperature for 18 hours. The reaction was quenched with saturated aqueous Na2S2O3 (10 mL), washed with brine (10 mL), and extracted with EA (15 mL × 3). The combined organic layers were dried with Na2SO4, and the residue concentrated under reduced pressure to yield 1.1083 g (75%) of iodoalkynyl peroxide 3 as a light yellow oil: Rf = 0.55 (20% EA/Hex); 1H δ 3.95 (t, J = 6.3, 2H), 2.40 (t, J = 6.8, 2H), 1.70 (p, J = 6.1, 2H), 1.59 (p, J = 7.0, 2H), 1.24 (s, 9H) 13C δ 94.4, 80.3, 74.4, 27.2, 26.5, 25.4, 20.8, −6.8; IR 2925, 1362, 1196, 877; HRMS (ESI+, TOF) calcd for C10H17INaO2 [M + Na]+: 319.0171; found: 319.0174.
1-Iodo-8-tert-butylperoxy octyne (4). By a similar procedure as employed for synthesis of iodohexynyl peroxide 3, reaction of 8-(tert-butylperoxy)-1-octyne (714.9 mg, 3.60 mmol), KI (685.5 mg, 4.13 mmol, 1.2 equiv.), and TBHP (1.0 mL, 5.5 mmol, 1.5 equiv.) furnished, after column chromatography (10% EA/Hex), 901.1 mg (77%) of iodooctynyl peroxide 4 as a light yellow oil: Rf: 0.57 (20% EA/Hex); 1H δ 3.95 (t, J = 6.7, 2H), 2.38 (t, J = 7.0, 2H), 1.62 (p, J = 7.1, 2H), 1.54 (p, J = 7.0, 2H), 1.26–1.36 (m, 4H), 1.26 (s, 9H); 13C δ 94.8, 80.2, 75.0, 28.7, 28.5, 27.9, 26.5, 25.8, 20.9, −7.4; IR: 2977, 2937, 2860, 1362, 1197; HRMS (ESI+, TOF) calcd for C12H21INaO2 [M + Na]+: 347.0484; found: 347.0485.
Synthesis of alkynyl peroxyacetals
3-((2-Hydroperoxypropan-2-yl)oxy)-1-propyne(5) and 3-((2-((2-hydroperoxypropan-2-yl)peroxy)propan-2-yl)oxy)-1-propyne (5a) were prepared using a procedure reported for unsaturated alcohols.19 To a solution of propargyl alcohol (0.97 mL, 16.9 mmol, 3 equiv.) in CH2Cl2 (50 mL) was added 2,3-dimethyl-2-butene (0.65 mL, 5.47 mmol). This mixture was cooled to −78 °C whereupon a gaseous stream of O3/O2 was admitted (pipette, ∼0.5–1 mmol O3/minute). When the reaction was complete (TLC) introduction of O3/O2 was halted and N2 was briefly bubbled through the reaction mixture. The solution was carefully concentrated under reduced pressure (CAUTION) and the residue purified by column chromatography (5% ether/pentane) to yield 210.8 mg (20%) of hydroperoxyperoxide 5a as a colorless oil, followed by traces of volatile hydroperoxyacetal 5. (CAUTION: the nearly 16% active oxygen content of hydroperoxyperoxide 5a suggests a moderate to high potential for exothermic decomposition.) As a result, this preparation was confined to <20 mmol scale and products were handled carefully (see general Discussion on safety, above).10d
5a: Rf = 0.46 (20% EA/Hex); 1H δ 9.61 (s, 1H), 4.31 (t, J = 2.4, 2H), 2.43 (t, J = 2.3, 1H), 1.51 (s, 6H), 1.42 (s, 6H); 13C δ 109.4, 106.8, 80.4, 74.3, 51.2, 23.5, 20.9; IR: 3362, 3286, 2999, 2946, 1369, 1176, 1034, 826, 620; HRMS (ESI+, TOF) calcd for C9H16NaO5 [M + Na]+: 227.0895; found: 227.0896.
5: (trace byproduct; incompletely characterized as a colorless oil): Rf = 0.43 (20% EA/Hex); 1H δ 9.88 (s, 1H), 4.20 (t, J = 2.4, 2H), 2.45 (t, J = 2.4, 1H), 1.41 (s, 6H); 13C δ 105.9, 81.3, 73.7, 49.9, 22.7.
4-((2-Hydroperoxypropan-2-yl)oxy)-1-butyne (6) and 4-((2-((2-hydroperoxypropan-2-yl)peroxy)propan-2-yl)oxy)-1-butyne (6a) were prepared using the same procedure described above. To a solution of 3-butyn-1-ol (1.97 mL, 26.0 mmol, 2 equiv.) in CH2Cl2 (125 mL) was added 2,3-dimethyl-2-butene (1.56 mL, 13.1 mmol). This mixture was cooled to −78 °C whereupon a gaseous stream of O3/O2 was admitted (approximately 1 mmol O3/minute). When the reaction as judged complete, by TLC, introduction of O3/O2 was halted and N2 was briefly bubbled through the reaction mixture. The solution was carefully concentrated under reduced pressure and the residue (CAUTION) purified by column chromatography (5% ether/pentane) to yield 455.6 mg (24%) of 6 as an colorless oil, accompanied by 141.5 mg (5%) of 6a. CAUTION: The products shown here are hydroperoxides or peroxy hydroperoxides with active oxygen contents suggesting a moderate to high potential for exothermic decomposition. These reactions were deliberately run on a modest scale and products should be handled carefully.
6a: Rf = 0.46 (20% EA/Hex); 1H δ 9.75 (s, 1H), 3.80 (t, J = 9.6, 2H), 2.59 (td, J = 6.1, J = 3.6, 2H), 2.01 (t, J = 3.6, 1H), 1.53 (s, 6H), 1.47 (s, 6H); 13C δ 109.4, 106.8, 80.4, 74.3, 51.2, 23.5, 20.9; IR: 3293, 2997, 2946, 1367, 1196, 1177, 1141, 1045, 834, 636; HRMS (ESI+, TOF) calcd for C9H16NaO5 [M + Na]+: 241.1052; found: 241.1052.
6: Rf = 0.53 (20% EA/Hex); 1H δ 8.46 (s, 1H), 3.65 (t, J = 5.4, 2H), 2.50 (td, J = 6.0, J = 2.6, 2H), 2.09 (t, J = 2.6, 1H), 1.49 (s, 6H); 13C δ 105.7, 83.7, 69.8, 60.0, 22.6, 20.5; IR: 3427, 3287, 2995, 2947, 1367, 1201, 1158, 1051, 816, 639; HRMS (ESI+, TOF) calcd for C7H12NaO3 [M + Na]+: 167.0684; found: 167.0681.
6-((2-Hydroperoxypropan-2-yl)oxy)-1-hexyne (7). By a similar procedure as described above, a mixture of 5-hexyn-1-ol (0.88 mL, 7.98 mmol, 3 equiv.) in CH2Cl2 (30 mL) and 2,3-dimethyl-2-butene (0.32 mL, 2.69 mmol) were reacted with O3/O2 to generate, after careful concentration and chromatography 328.6 mg (71%) of hydroperoxyacetal 7 as a colorless oil: Rf = 0.41 (20% EA/Hex); 1H δ 7.89 (s, 1H), 3.51 (t, J = 6.5, 2H), 2.22 (td, J = 6.7, J = 2.6, 2H), 1.95 (t, J = 2.6, 1H), 1.73–1.57 (m, 4H), 1.39 (s, 6H); 13C δ 105.3, 84.5, 68.7, 61.2, 29.1, 25.3, 22.8, 18.3; IR: 3296, 2993, 2943, 2872, 2117, 1740, 1366, 1199, 1151, 1061, 866, 629; HRMS (ESI+, TOF) calcd for C9H16NaO3 [M + Na]+: 195.0997; found: 195.0995.4-((2-Methylperoxypropan-2-yl)oxy)-1-butyne (8) was prepared using an adaptation of a reported procedure.21,57 To a solution of hydroperoxyacetal 6 (359 mg, 2.49 mmol) in THF (10 mL) was added potassium tert-butoxide (350 mg, 3.12 mmol, 1.2 equiv.), followed by methyl iodide (0.19 mL, 3.05 mmol, 1.2 equiv.). After the reaction had stirred at room temperature for 5 minutes, it was quenched with water (10 mL) and the resulting mixture extracted with ether (15 mL × 3). The combined organic layers were dried with Na2SO4 and concentrated under reduced pressure. The residue was purified by column chromatography (10% ether/pentane) to yield 262.2 mg (66%) of peroxyacetal 8 as a colorless oil: Rf = 0.54 (20% EA/Hex); 1H δ 3.85 (s, 3H), 3.66 (t, J = 9.8, 2H), 2.47 (td, J = 9.8, J = 3.6, 2H), 1.97 (t, J = 3.6, 1H), 1.40 (s, 6H); 13C δ 104.9, 81.5, 69.4, 63.4, 60.2, 23.2, 20.3; IR: 2992, 2943, 2894, 1379, 1200, 1152, 1042, 864; HRMS (ESI+, TOF) calcd for C8H14NaO3 [M + Na]+: 181.0814; found: 181.0841.
6-((2-Methylperoxypropan-2-yl)oxy)-1-hexyne (9). By a similar procedure as described for above, a solution of hydroperoxyacetal 7 (146 mg, 0.85 mmol) in THF (5 mL) was reacted with potassium tert-butoxide (114 mg, 1.02 mmol, 1.2 equiv.) and methyl iodide (0.10 mL, 1 mmol, 1.2 equiv.), to furnish, after purification, 142.5 mg (90%) of peroxyacetal 9 as an colorless oil: Rf = 0.45 (20% EA/Hex); 1H δ 3.84 (s, 3H), 3.53 (t, J = 8.3, 2H), 2.23 (td, J = 9.3, J = 3.4, 2H), 1.93 (t, J = 3.1, 1H), 1.71–1.59 (m, 4H), 1.38 (s, 6H); 13C δ 104.7, 84.6, 68.4, 63.3, 61.0, 29.1, 25.4, 23.5, 23.2, 18.3; IR: 3294, 2943, 2117, 1365, 1205, 1153, 1067, 1015, 869, 629; HRMS (ESI+, TOF) calcd for C10H18NaO3 [M + Na]+: 209.1154; found: 209.1153.
1-Iodo-4-((2-methylperoxypropan-2-yl)oxy)-1-butyne (10). By a similar procedure as employed for synthesis of iodoalkynyl peroxide 3, reaction of peroxyacetal 8 (141 mg, 0.89 mmol) in MeOH (3 mL) with KI (183 mg, 1.10 mmol, 1.2 equiv.) followed by TBHP as an ∼5.5 M solution in decane (0.25 mL, 1.38 mmol, 1.2 equiv.) furnished, after concentration under reduced pressure. 206.7 mg (81%) of iodoalkynyl peroxyacetal 10 as a light yellow oil: Rf = 0.54 (10% EA/Hex); 1H δ 3.85 (s, 3H), 3.64 (t, J = 7.3, 2H), 2.64 (t, J = 7.4, 2H), 1.39 (s, 6H); 13C δ 104.9, 91.5, 63.4, 60.2, 23.2, 22.6, −5.4; IR: 2992, 2940, 2891, 1366, 1199, 1152, 1073, 1013, 862; HRMS (ESI+, TOF) calcd for C8H13INaO2 [M + Na]+: 306.9807; found: 306.9811.
1-Iodo-6-((2-methylperoxypropan-2-yl)oxy)-1-hexyne (11). By a similar procedure as employed for synthesis of 3, reaction of peroxyacetal 9 (242 mg, 1.30 mmol) in MeOH (10 mL) with KI (267 mg, 1.61 mmol, 1.2 equiv.) and TBHP as an ∼5.5 M solution in decane (0.36 mL, 1.98 mmol, 1.5 equiv.) furnished iodoalkynyl peroxyacetal 11 (400.8 mg, 98%) of as a light yellow oil: Rf = 0.49 (10% EA/Hex); 1H δ 3.85 (s, 3H), 3.52 (t, J = 6.5, 2H), 2.40 (t, J = 6.8, 2H), 1.69–1.54 (m, 4H), 1.38 (s, 6H); 13C δ 104.7, 94.7, 63.3, 61.0, 29.2, 25.4, 23.2, 20.8, −7.1; IR: 3299, 2940, 1466, 1378, 1365, 1204, 1152, 1013, 858, 632; HRMS (ESI+, TOF) calcd for C10H17INaO2 [M + Na]+: 335.0120; found: 335.0126.
Synthesis of peroxy azides
1-Azido-(4-(tert-butylperoxy))butane (12). To a solution of 1-bromo-4-chlorobutane (2.57 g, 15.0 mmol) in DMF (40 mL) was added sodium azide (1.96 mg, 30.1 mmol, 2 equiv.). The reaction was stirred at room temperature for 24 hours. The reaction was diluted with (20 mL) water and extracted with hexanes (15 mL × 3). The combined organic layers were dried with Na2SO4 and the residue concentrated under reduced pressure to yield 1.8975 g (95%) of 1-azido-4-chlorobutane as a colorless oil which was used without further purification: Rf = 0.49 (10% EA/Hex). Spectral details matched those previously reported.22 Rf = 0.49 (10% EA/Hex).A solution of the 1-azido-4-chlorobutane (2.40 g, 18.0 mmol) and sodium iodide (15.0 g, 100 mmol, 5.5 equiv.) was refluxed in acetone (150 mL) for 18 hours. The reaction was then diluted with water (60 mL) and extracted with hexanes (50 mL × 3). The combined organic layers were dried with Na2SO4 and the residue concentrated under reduced pressure to yield 3.6943 g (91%) of 1-azido-4-iodobutane as an orange oil which was used without further purification. Spectral details matched those previously reported.22 Rf = 0.5 (10% EA/Hex); 1H δ 3.32 (t, J = 6.6, 2H), 3.20 (t, J = 6.7, 2H), 1.91 (p, J = 7.4, 2H), 1.71 (p, J = 7.4, 2H); 13C δ 50.5, 30.6, 30.0, 7.2.
By a similar procedure as applied for synthesis of peroxide 1, reaction of CsOH monohydrate (127 mg, 0.85 mmol, 1.2 equiv.) in DMF (12 mL) at 0 °C, TBHP as an ∼5.5 M solution in decane (0.19 mL, 1.05 mmol, 1.5 equiv., ∼5.5 M solution in decane), and 1-azido-4-iodobutane (158 mg, 0.70 mmol) furnished, after purification by chromatography (5% EA/Hex), 80.9 mg (62%) of the azidobutyl t-butyl peroxide (12) as a colorless oil (CAUTION: low molecular weight azide/peroxide): Rf = 0.51 (10% EA/Hex); 1H δ 3.96 (m, 2H), 3.30 (t, J = 6.4, 2H), 1.71–1.66 (m, 4H), 1.24 (s, 9H); 13C δ 80.3, 74.7, 51.4, 26.5, 26.0, 25.4; IR: 2977, 2931, 2093, 1456, 1362, 1245, 1196, 881; HRMS (ESI+, TOF) calcd for C8H17N3NaO2 [M + Na]+: 210.1218; found: 210.1213.
((6-Azidohexan-2-yl)peroxy)triethylsilane (13). Into a RBF containing CH2Cl2 (300 mL), were added sequentially iodine (7.61 g, 30.0 mmol), PPh3 (7.87 g, 30.0 mmol) and imidazole (2.04 g, 30.0 mmol). The mixture was stirred for 15 minutes at 0 °C, followed by the addition of 5-hexenol (2.50 g, 25.0 mmol). Reaction was stirred at room temperature and filtered through silica with hexanes (600 mL). The filtrate was concentrated under reduced pressure to yield 3.8557 g (75%) of 6-iodohexene as a light orange oil which was used without further purification. Spectral details matched those previously reported.58 Rf = 0.58 (10% EA/Hex); 1H δ 5.02 (dq, J = 17.0, J = 1.8, 1H), 4.96 (ddt, J = 10.0, 1.8, 0.9, 1H), 5.78 (dddd, J = 17.2, J = 10.5, J = 6.7, J = 6.7, 1H), 3.19 (t, J = 7.0, 2H), 2.08 (q, J = 7.0, 2H), 1.84 (p, J = 7.5, 2H), 1.50 (p, J = 7.5, 2H); 13C δ 138.2, 115.1, 33.0, 32.7, 29.8, 7.0.To a solution of the iodohexene (1.20 g, 5.71 mmol) in DMF (20 mL) was added sodium azide (1.11 g, 17.1 mmol, 3 equiv.). The reaction was stirred at room temperature for 18 hours and then diluted with water (10 mL). The combined hexane extracts (3 × 15 mL) were dried with Na2SO4 and the residue concentrated under reduced pressure to yield 688.5 mg (98%) of 6-azido-hexene as a colorless oil which was used without further purification. Spectral details matched those previously reported:59 Rf = 0.59 (10% EA/Hex); 1H δ 5.01 (dq, J = 17.2, J = 1.6, 1H), 4.97 (d, J = 10.2, 1H), 5.79 (dddd, J = 17.0, J = 10.5, J = 6.7, J = 6.7, 1H) 3.27 (t, J = 6.8, 2H), 2.09 (q, J = 7.1, 2H), 1.62 (p, J = 7.5, 2H), 1.48 (p, J = 7.6, 2H); 13C δ 138.3a 115.1, 51.5, 33.3, 28.4, 26.0.
To a solution of the azidohexene (113 mg, 0.90 mmol) in EtOH (10 mL) was added triethylsilane (0.29 mL, 1.82 mmol, 2 equiv.), followed by Co(acac)2 (25.7 mg, 0.10 mmol, 0.1 equiv.). The reaction was stirred at room temperature under O2 for 18 hours.23 The solution was concentrated under reduced pressure and the residue purified by column chromatography (5% EA/Hex) to yield 114.0 mg (46%) of azido peroxide 13 as a colorless oil: Rf = 0.5 (10% EA/Hex); 1H δ 4.01 (s, J = 5.8, 1H), 3.27 (t, J = 6.9, 2H), 1.61–1.40 (m, 6H), 1.20 (d, J = 6.2, 3H), 0.99 (t, J = 7.9, 9H), 0.69 (q, J = 8.0, 6H); 13C δ 81.2, 51.5, 34.0, 29.1, 22.8, 18.5, 6.9, 3.9; IR; 2938, 2877, 2093, 1459, 1242, 1006, 796, 727; HRMS (ESI+, TOF) calcd for C12H27N3NaO2Si [M + Na]+: 296.1770; found: 296.1772.
Hexyne (14) was used as received.
Hex-5-yn-1-yl benzene (15) (100848-88-2). Alkyne 15 was prepared using a variant of a reported procedure.60 To a solution of ethynyl trimethylsilane (998.3 mg, 3.84 mmol) in THF (10 mL) was added n-BuLi (2.7 mL, 4.32 mmol, 1.1 equiv.) at −78 °C, followed by 4-(iodobutyl)benzene (386.2 mg, 3.93 mmol, 1.02 equiv.). The reaction was allowed to warm to room temperature and stirred for 4 hours. The reaction was quenched with water (30 mL) and the combined ethyl acetate extracts (3 × 30 mL) were dried with Na2SO4. Concentration under reduced pressure yielded 825.2 mg (85%) of trimethyl(6-phenylhex-1-yn-1-yl)silane (2253948-27-3) as a colorless oil which was used directly in the following reaction. Rf = 0.60 (10% EA/Hex). Spectra details matched those in a literature report.61To a solution of the crude alkynyl silane (743.2 mg, 3.09 mmol) in methanol (25 mL) was added potassium hydroxide (304.0 mg, 5.42 mmol, 1.75 equiv.). The reaction was stirred at room temperature for 2 hours.62 The reaction was quenched with water (40 mL) and extracted with hexanes (30 mL × 3). The combined organic layers were dried with Na2SO4, concentrated under reduced pressure and the residue purified by column chromatography (5% EA/Hex) to yield 499.0 mg (89%) of the hex-5-yn-1-yl benzene as a colorless oil. Spectral details matched those previously reported:61,63 Rf = 0.65 (10% EA/Hex); 1H δ 7.31 (m, 2H), 7.24–7.21 (m, 3H), 2.67 (t, J = 7.9, 2H), 2.25 (td, J = 2.6, J = 7.1, 2H), 1.98 (t, J = 2.6, 1H), 1.78 (p, J = 7.8, 2H), 1.61 (p, 7.5, 2H); 13C δ 142.4, 128.5, 128.4, 125.9, 84.5, 68.4, 35.5, 30.5, 28.1, 18.4; IR: 3305, 2927, 2854, 1495, 1453, 744, 696.
3-Azidopropylbenzene (16). Iodine (11.74 g, 46.3 mmol), PPh3 (11.89 g, 45.3 mmol) and imidazole (3.16 g, 46.4 mmol) were sequentially added to a solution of CH2Cl2 (300 mL). The mixture was stirred for 15 minutes at 0 °C, whereupon 3-phenyl-1-propanol (5.07 g, 37.2 mmol) was added. The reaction was stirred at room temperature for 5 hours and filtered through a ∼3′′ pad of silica with hexanes (600 mL). The filtrate was concentrated under reduced pressure to yield 9.05 g (98%) of 3-iodopropylbenzene as a light orange oil which was used without further purification. Spectral details matched those previously reported.64 Rf: 0.50 (10% EA/Hex); 1H δ 7.33 (t, J = 7.4, 2H), 7.26 (t, J = 8.1, 1H), 7.24 (d, J = 6.6, 2H), 3.21 (t, J = 6.8, 2H), 2.77 (t, J = 7.3, 2H), 2.17 (p, J = 7.2, 2H); 13C δ 140.5, 128.7, 128.6, 126.3, 36.4, 35.0, 6.5.To a solution of the iodopropyl benzene (1.10 g, 4.48 mmol) in DMF (20 mL) was added sodium azide (875.6 g, 13.4 mmol, 3 equiv.). The reaction was stirred at room temperature for 18 hours. The reaction was diluted with water (10 mL) and extracted with CH2Cl2 (15 mL × 3). The combined organic layers were dried with Na2SO4 and the residue concentrated under reduced pressure to yield 694.1 mg (96%) of 3-azidopropylbenzene as a colorless oil. Spectral details matched those previously reported.65 Rf: 0.52 (10% EA/Hex); 1H δ 7.34 (t, J = 7.4, 2H), 7.26 (t, J = 7.4, 1H), 7.22 (d, J = 7.3, 2H), 3.32 (t, J = 6.8, 2H), 2.75 (t, J = 7.5, 2H), 1.95 (p, J = 7.2, 2H); 13C δ 141.0, 128.6, 128.6, 126.3, 50.8, 32.9, 30.6.
4-Azidobutylbenzene (17). Using a similar procedure as described above, reaction of a solution of iodine (7.18 g, 28.3 mmol), PPh3 (7.40 g, 28.2 mmol), imidazole (1.91 g, 28.1 mmol) and 4-phenyl-1-butanol (3.47 g, 23.1 mmol) in CH2Cl2 (300 mL) furnished 4.6282 g (77%) of 4-iodobutylbenzene as a light orange oil. Spectral details matched those previously reported.66 Rf = 0.51 (10% EA/Hex); 1H δ 7.32 (dt, J = 5.4, J = 1.5, 2H), 7.24 (dt, J = 8.4, 1.2, 1H), 7.21 (d, J = 6.8, 2H), 3.23 (t, J = 6.8, 2H), 2.67 (t, J = 7.6, 2H), 1.89 (m, 2H), 1.77 (m, 2H); 13C δ 141.9, 128.5, 126.0, 34.9, 33.1, 32.3, 6.9.Using a similar procedure as described above, reaction of the 4-iodobutylbenzene (1.25 g, 4.81 mmol) with sodium azide (969 mg, 14.9 mmol, 3 equiv.) in DMF (20 mL) furnished 811.7 mg (97%) of 4-azidobutylbenzene (17) as a colorless oil. Spectral details matched those previously reported.66 Rf = 0.53 (10% EA/Hex); 1H δ 7.32 (t, J = 7.3, 2H), 7.22 (t, J = 7.3, 1H), 7.21 (d, J = 7.3, 2H), 3.31 (t, J = 6.5, 2H), 2.68 (t, J = 7.6, 2H), 1.75 (m, 2H), 1.67 (m, 2H); 13C δ 142.0, 128.5, 128.5, 126.0, 51.5, 35.5, 28.6.
6-Azidohexane-1-thiol (18). This molecule, which is commercially available, has been previously described without characterization.67Synthesis of S-(6-hydroxyhexyl)ethanethioate was adapted from a reported procedure.67 To a solution of 6-bromohexanol (3.07 g, 17.0 mmol) in acetone (175 mL) was added potassium thioacetate (3.92 g, 34.3 mmol, 2 equiv.). The reaction was stirred at 40 °C for 18 hours. The reaction was then quenched with 1 M NaHCO3 (50 mL) and the mixture extracted with ether (50 mL × 3). The combined organic layers were dried with Na2SO4, concentrated under reduced pressure, and the residue was purified by column chromatography (5–20% ether/pentane) to yield 1.89 g (63%) of the thioester as a red oil. Spectral details matched those previously reported.68 Rf = 0.30 (30% EA/Hex); 1H δ 3.62 (t, J = 6.4, 2H), 2.86 (t, J = 6.8, 2H), 2.31 (s, 3H), 1.53–1.62 (m, 4H), 1.35–1.43 (m, 4H); 13C δ 196.2, 62.9, 32.7, 30.8, 29.6, 29.1, 28.6, 25.3; IR: 3292, 2944, 1380, 1205, 1157, 1064, 831, 630.
The following reagents were sequentially added to CH2Cl2 (300 mL): iodine (2.86 g, 11.3 mmol, 1.2 equiv.), PPh3 (2.96 g, 11.3 mmol, 1.2 equiv.) and imidazole (769.3 mg, 11.3 mmol, 1.2 equiv.). The mixture was stirred for 15 minutes at 0 °C, whereupon S-(6-hydroxyhexyl)ethanethioate (1.66 g, 9.42 mmol) was added. The reaction was stirred at room temperature for 5 hours and filtered through a 7.5 cm pad of silica with hexanes (600 mL). The filtrate was concentrated under reduced pressure to yield 1.98 g (73%) of S-(6-iodohexyl)ethanethioate as a brown oil which was used directly for the next reaction: Rf = 0.40 (5% EA/Hex); 1H δ 3.20 (t, J = 7.0, 2H), 2.89 (t, J = 7.3, 2H), 2.35 (s, 3H), 1.84 (p, J = 6.8, 2H), 1.61 (p, J = 6.9, 2H), 1.37–1.47 (m, 4H); 13C δ 196.1, 33.4, 30.8, 30.1, 29.4, 29.1, 27.8, 7.0; IR: 2929, 1686, 1132, 954, 623; HRMS (ESI+, TOF) calcd for C8H15IOS [M]+: 285.9888; found: 285.9898.
To a solution of S-(6-azidohexyl)ethanethioate (1.16 g, 4.05 mmol) in DMF (20 mL) was added sodium azide (1.45 g, 22.3 mmol, 5.5 equiv.). The reaction was stirred at room temperature for 18 hours. The reaction was diluted with water (10 mL) and extracted with CH2Cl2 (15 mL × 3). The combined organic layers were dried with Na2SO4 and the residue concentrated under reduced pressure to yield 762.6 mg (94%) of azido/ethioester as an orange oil which was used without further purification. Spectral details matched those previously reported.69 Rf = 0.32 (5% EA/Hex); 1H δ 3.25 (t, J = 7.1, 2H), 2.86 (t, J = 7.1, 2H), 2.32 (s, 3H), 1.54–1.61 (m, 4H), 1.37–1.40 (m, 4H); 13C δ 196.0, 51.5, 30.8, 29.5, 29.0, 28.8, 28.4, 26.3; IR: 2933, 2090, 1688, 1256, 1132, 951, 624.
The deblocking of the thioacetate employed a procedure reported for a nine-carbon homolog.70 To a solution of S-(6-azidohexyl)ethanethioate (762.6 g, 3.79 mmol) in MeOH (70 mL) was added conc. aq. HCl (4.0 mL). The reaction was stirred at reflux for 3 hours and then allowed to cool. The reaction was diluted with water (20 mL) and extracted with diethyl ether (25 mL × 3). The combined organic layers were dried with Na2SO4 and the residue concentrated under reduced pressure to yield 559.8 mg (93%) of 6-azidohexane-1-thiol as a strong-smelling orange oil. Rf = 0.35 (5% EA/Hex); 1H δ 3.27 (t, J = 6.6, 2H), 2.53 (q, J = 7.2, 2H), 1.57–1.64 (m, 4H), 1.37–1.43 (m, 4H); IR: 3930, 3857, 2400 (w); 2086 (s); 1454, 1253; 13C δ 51.5, 33.9, 28.9, 28.0, 26.3, 24.6 IR: 2930, 2087, 1254; HRMS (ESI+, TOF) calcd for C6H13N3S [M]+: 159.0830; found: 159.0867, 316.1512 (disulfide).
General procedure for click reactions
Click reactions were conducted based upon adaptations of a reported procedure.25b
General procedure for click reactions with CuI. To a solution of alkyne (1 equiv.) and azide (1 equiv.) in THF was added CuI (0.2 equiv.), followed by triethylamine (3 equiv.). The reaction was stirred at room temperature for 3 hours, then diluted with water and extracted with ethyl acetate (×3). The combined organic layers were dried with Na2SO4, concentrated under reduced pressure and the residue purified by column chromatography.
General procedure for click reactions with CuSO4. To a solution of alkyne (1 equiv.) and azide 16 (1 equiv.) in CH2Cl2 was added an aqueous solution containing CuSO4 (0.2 equiv.) and sodium ascorbate (0.2 equiv.). After stirring at rt for 3 h, the reaction was diluted with water and extracted with ethyl acetate (×3). The combined organic layers were dried with Na2SO4, concentrated under reduced pressure and the residue purified by column chromatography.
4-(4-(tert-Butylperoxy)butyl)-1-(3-phenylpropyl)-1H-1,2,3-triazole (19). To a solution of peroxyhexyne 1 (203 mg, 1.19 mmol) and azide 16 (193 mg, 1.20 mmol) in THF (5 mL) was added CuI (47.6 mg, 0.25 mmol, 0.2 equiv.),25b followed by triethylamine (0.50 mL, 3.6 mmol, 3 equiv.) and triethylsilane (0.21 mL, 1.3 mmol, 1.1 equiv.). The reaction was stirred at room temperature for 24 hours. The reaction was diluted with water (10 mL) and extracted with EA (15 mL × 3). The combined organic layers were dried with Na2SO4, concentrated under reduced pressure and the residue purified by column chromatography (10% EA/Hex) to yield 156.2 mg (38%) of triazole 19 as a yellow oil: Rf = 0.28 (40% EA/Hex); 1H δ 7.29 (t, J = 7.4, 2H), 7.26 (s, 1H), 7.23 (t, J = 7.4, 1H), 7.19 (d, J = 7.1, 2H), 4.33 (t, J = 7.1, 2H), 3.99 (t, J = 6.3, 2H), 2.77 (t, J = 7.4, 2H), 2.67 (t, J = 7.5, 2H), 2.25 (p, J = 7.3, 2H), 1.78 (m, 2H), 1.69 (m, 2H), 1.25 (s, 9H); 13C δ 148.0, 140.4, 128.7, 128.5, 126.4, 120.7, 80.2, 74.8, 49.5, 32.7, 31.8, 27.6, 26.5, 26.2, 25.6; IR: 2976, 2932, 2866, 1497, 1454, 1362, 1196, 1046, 878, 746, 670, 493; HRMS (ESI+, TOF) calcd for C19H29N3NaO2 [M + Na]+: 354.2157; found: 354.2155.
4-(4-(tert-Butylperoxy)butyl)-1-(4-phenylbutyl)-1H-1,2,3-triazole (20) and 4-(4-(tert-butylperoxy)butyl)-5-iodo-1-(4-phenylbutyl)-1H-1,2,3-triazole (20i). To a solution of 6-(tert-butylperoxy)-1-hexyne (238 mg, 1.40 mmol) and 4-azidobutylbenzene (209 mg, 1.19 mmol) in THF (5 mL) was added CuI (47.6 mg, 0.25 mmol, 0.2 equiv.), followed by triethylamine (0.60 mL, 4.29 mmol, 3 equiv.).46 The reaction was stirred at room temperature for 1 hour. The reaction was diluted with water (10 mL) and extracted with EA (15 mL × 3). The combined organic layers were dried with Na2SO4, concentrated under reduced pressure and the residue purified by column chromatography (10% EA/Hex) to yield 154.4 mg (37%) of triazole 20 as a yellow oil. Reactions conducted in the presence of CuI often contained traces of the corresponding 5-iodotriazole (20i).20: Rf = 0.27 (40% EA/Hex); 1H δ 7.29 (t, J = 7.4, 2H), 7.24 (s, 1H), 7.21 (t, J = 7.4, 1H), 7.16 (d, J = 7.2, 2H), 4.33 (t, J = 7.2, 2H), 3.98 (t, J = 6.1, 2H), 2.75 (t, J = 7.2, 2H), 2.66 (t, J = 7.5, 2H), 1.93 (p, J = 7.5, 2H), 1.80–1.62 (m, 6H), 1.25 (s, 9H); 13C δ 150.0, 141.6, 128.5, 128.5, 126.1, 120.6, 80.2, 74.8, 50.1, 35.3, 29.9, 28.3, 27.6, 26.4, 26.2, 25.6 ppm; IR: 3026, 2934, 1454, 1362, 1196, 1044, 747, 699; HRMS (ESI+, TOF) calcd for C20H31N3NaO2 [M + Na]+: 368.2314; found: 368.2312.
20i: Rf = 0.31 (40% EA/Hex); 1H δ 7.21 (t, J = 7.2, 2H), 7.18 (m, 3H), 4.37 (t, J = 7.2, 2H), 3.99 (t, J = 6.4, 2H), 2.70 (t, J = 7.2, 2H), 2.68 (t, J ∼ 7, 2H), 1.95 (p, J = 7.2, 2H), 1.80 (m, 2H), 1.76 (m, 4H), 1.25 (s, 9H); 13C δ 151.60, 141.7, 128.58, 128.55, 126.1, 120.6, 80.3, 78.1, 74.8, 50.6, 35.3, 29.5, 28.2, 27.6, 26.4, 26.1, 25.8 ppm; IR: 2976, 2936, 1454, 1362, 1197, 1044, 743, 699; HRMS (ESI+, TOF) calcd for C20H30IN3NaO2 [M + Na]+: 494.1280; found: 494.1276.
4-(1-(4-Phenylbutyl)-1H-1,2,3-triazol-4-yl)butanal (21). This colorless oil, which coelutes with the parent peroxide, was a minor byproduct in many of the click reactions but became a major byproduct for reactions conducted for long periods or when the peroxy triazole products were resubjected to reaction conditions: Rf = 0.27 (40% EA/Hex); 1H δ 9.79 (t, J = 0.7, 1H), 7.32–7.16 (m, 6H), 4.34 (t, J = 7.2, 2H), 2.78 (t, J = 7.4, 2H), 2.68 (t, J = 7.5, 2H), 2.55 (td, J = 1.4, J = 7.3, 2H), 2.04 (p, J = 7.3, 2H), 1.94 (p, J = 7.6, 2H), 1.67 (m, 2H); 13C (176 MHz) δ 202.2, 147.2, 141.6, 128.6, 128.5, 126.2, 120.8, 50.2, 43.3, 35.3, 32.1, 28.3, 27.2, 21.9; IR: 3135, 3025, 2925, 2723, 1722, 1454, 1275, 748, 701; HRMS (ESI+, TOF) calcd for C20H31N3NaO2 [M + Na]+: 294.1582; found: 294.1588.
Stability of peroxyalkyne towards CuI
To 6-(tert-butylperoxy)-1-hexyne 1 (170 mg, 1.0 mmol) in THF (5 mL) was added CuI (38 mg, 0.20 mmol, 0.2 equiv.). The reaction was stirred at room temperature for 24 hours. The reaction was quenched with water and extracted with ethyl acetate (15 mL × 3). The combined organic layers were dried with Na2SO4, concentrated under reduced pressure to yield 153.4 mg (90%) of recovered starting material as a colorless oil: Rf = 0.55 (10% EA/Hex).
Reactions in the presence of TBTA
CuSO4. To 6-(tert-butylperoxy)-1-hexyne 1 (272 mg, 1.6 mmol) and 4-phenyl-butyl-azide 17 (228 mg, 1.3 mmol) in 1
:
1 DMSO/water (10 mL) was added CuSO4 (25.5 mg, 0.16 mmol, 0.1 equiv.), sodium ascorbate (47.5 mg, 0.24 mmol, 0.15 equiv.), and TBTA (8.4 mg, 0.016 mmol, 0.01 equiv.). The reaction was stirred at room temperature for 1.5 hours. The reaction was quenched with water and extracted with ethyl acetate (15 mL × 3). The combined organic layers were dried with Na2SO4, concentrated under reduced pressure and purified by column chromatography (10% EA/Hex) to yield 89.9 mg (20%) of triazole 20 as a yellow oil: Rf = 0.28 (40% EA/Hex).
CuI. To 6-(tert-butylperoxy)-1-hexyne 1 (238 mg, 1.4 mmol) and azide 17 (245 mg, 1.4 mmol) in DMSO
:
water (2.5 mL
:
2.5 mL) was added CuI (57 mg, 0.30 mmol, 0.2 equiv.) and triethylamine (0.6 mL, 4.2 mmol, 3 equiv.), followed by TBTA (7.4 mg, 0.014 mmol, 0.01 equiv.). The reaction was stirred at room temperature for 3 hours. The reaction was quenched with water and extracted with ethyl acetate (15 mL × 3). The combined organic layers were dried with Na2SO4, concentrated under reduced pressure and purified by column chromatography (10% EA/Hex) to yield 166.3 mg (35%) of triazole 20 as a yellow oil: Rf = 0.28 (40% EA/Hex).
Reaction of iodoalkyne
4-(4-(tert-Butylperoxy)butyl)-5-iodo-1-(3-phenylpropyl)-1H-1,2,3-triazole (19i). To a solution of 1-iodo-6-tert-butylperoxy hexyne 3 (118 mg, 0.40 mmol) and 3-azidopropylbenzene 16 (64.5 mg, 0.41 mmol) in THF (3.5 mL) was added CuI (7.6 mg, 0.04 mmol, 0.1 equiv.), followed by triethylamine (0.12 mL, 0.86 mmol, 2 equiv.).12 The reaction was stirred at room temperature for 24 hours. The reaction was diluted with water (10 mL) and extracted with EA (15 mL × 3). The combined organic layers were dried with Na2SO4, concentrated under reduced pressure and the residue purified by column chromatography (10% EA/Hex) to yield 116.4 mg (64%) of iodotetrazole 19i as a yellow oil: Rf = 0.36 (20% EA/Hex); 1H δ 7.32 (t, J = 7.5, 2H), 7.24 (t, J = 7.5, 1H), 7.22 (d, J = 7.6, 2H), 4.37 (t, J = 7.3, 2H), 3.99 (t, J = 6.4, 2H), 2.70 (td, J = 7.5, J = 3.4, 4H), 2.25 (p, J = 7.4, 2H), 1.80 (p, J = 7.6, 2H), 1.68 (p, J = 6.3, 2H), 1.25 (s, 9H); 13C δ 151.6, 140.4, 128.7, 128.6, 126.4, 80.2, 78.1, 74.7, 50.1, 32.6, 31.3, 27.6, 26.5, 26.0, 25.8; IR: 3031, 2936, 2098, 1461, 1361, 1196 1022, 880, 752, 699, 509; HRMS (ESI+, TOF) calcd for C19H28IN3NaO2 [M + Na]+: 480.1124; found: 480.1126.
Competition of iodoalkynyl peroxide with simple alkyne
To a solution of 6-(tert-butylperoxy)-1-iodohexyne 3 (0.5 mmol), 5-hexyn-1-ol (0.5 mmol) and 3-phenylpropyl azide 16 (0.5 mmol) in THF (5 mL) was added CuI (0.050 mmol, 0.1 equiv.), followed by triethylamine (1.0 mmol, 2 equiv.). The reaction was stirred at room temperature for 18 hours. The reaction was quenched with water and extracted with ethyl acetate (15 mL × 3). The combined organic layers were dried with Na2SO4, concentrated under reduced pressure and purified by column chromatography (10% EA/Hex) to yield 64.5 mg (29%) of 4-(4-(tert-butylperoxy)butyl)-5-iodo-1-(3-phenylpropyl)-1H-1,2,3-triazole (19i) as a yellow oil.
4-(6-(tert-Butylperoxy)hexyl)-1-(3-phenylpropyl)-1H-1,2,3-triazole (6/3) (22) and 4-(6-(tert-butylperoxy)hexyl)-5-iodo-1-(3-phenylpropyl)-1H-1,2,3-triazole and 6-(1-(3-phenylpropyl)-1H-1,2,3-triazol-4-yl)hexanal (24). To a solution of 8-(tert-butylperoxy)-1-octyne 2 (304.1 mg, 1.53 mmol) and 3-azidopropylbenzene 16 (268.4 mg, 1.66 mmol, 1.1 equiv.) in THF (6 mL) was added CuI (64.8 mg, 0.25 mmol, 0.2 equiv.), followed by triethylamine (0.65 mL, 4.66 mmol, 3 equiv.).3 The reaction was stirred at room temperature for 3 hours. The reaction was diluted with water (10 mL) and extracted with EA (15 mL × 3). The combined organic layers were dried with Na2SO4, concentrated under reduced pressure and the residue purified by column chromatography (10% EA/Hex) to yield 374.3 mg (68%) of peroxy triazole 22, 45.9 mg (6%) of iodotriazole 22i and 47.1 mg (11%) of aldehyde 24.22: Rf: 0.28 (40% EA/Hex); 1H δ 7.31 (t, J = 7.5, 2H), 7.24 (s, 1H), 7.22 (t, J = 7.6, 1H), 7.19 (d, J = 7.4, 2H), 4.33 (t, J = 7.1, 2H), 3.94 (t, J = 6.5, 2H), 2.73 (t, J = 7.6, 2H), 2.66 (t, J = 7.5, 2H), 2.25 (p, J = 7.3, 2H), 1.69 (p, J = 7.4, 2H), 1.61 (p, J = 6.8, 2H), 1.36–1.45 (m, 4H), 1.25 (s, 9H); 13C δ 148.4, 140.4, 128.7, 128.5, 126.4, 120.6, 80.2, 75.1, 49.4, 32.7, 31.8, 29.5, 29.2, 27.9, 26.5, 26.1, 25.7; IR: 2976, 2933, 2859, 1454, 1361, 1197, 1047; HRMS (ESI+, TOF) calcd for C21H33N3NaO2 [M + Na]+: 382.2470; found: 382.2467.
22i: Rf: 0.32 (40% EA/Hex); 1H δ 7.31 (t, J = 7.3, 2H), 7.21–7.24 (m, 3H), 4.36 (t, J = 7.2, 2H), 3.94 (t, J = 6.6, 2H), 2.68 (app p, J = 8.1, J = 7.6, 4H), 2.25 (p, J = 7.2, 2H), 1.71 (p, J = 7.1, 2H), 1.61 (p, J = 6.6, 2H), 1.40–1.41 (m, 4H), 1.25 (s, 9H); 13C δ 151.9, 140.4, 128.7, 128.6, 126.4, 80.1, 78.0, 75.1, 50.1, 32.6, 31.3, 29.1, 29.0, 27.9, 26.5, 26.1, 26.1; IR: 2974, 2934, 2861, 1453, 1362; HRMS (ESI+, TOF) calcd for C21H32IN3NaO2 [M + Na]+: 508.1437; found: 508.1423.
24: Rf: 0.27 (40% EA/Hex); 1H δ 9.76 (t, J = 1.69, 1H), 7.30 (t, J = 7.4, 2H), 7.25 (s, 1H), 7.22 (t, J = 7.2, 1H), 7.18 (d, J = 7.3, 2H), 4.32 (t, J = 7.2, 2H), 2.73 (t, J = 7.6, 2H), 2.65 (t, J = 7.6, 2H), 2.44 (td, J = 1.7, J = 7.3, 2H), 2.24 (p, J = 7.6, 2H), 1.69 (m, 2H), 1.58 (p, J = 6.8, 2H) 1.42 (m, 2H); 13C (176 MHz) δ 202.7, 148.4, 140.4, 128.7, 128.5, 126.4, 120.6, 62.9, 49.5, 43.9, 32.7, 31.8, 25.5, 21.9, 14.3; IR: 2928, 2856, 1720, 1453, 1212, 1047, 1029 cm−1; HRMS (ESI+, TOF) calcd for C17H23N3NaO [M + Na]+: 308.1739; found: 308.1734.
4-(6-(tert-Butylperoxy)hexyl)-1-(3-phenylpropyl)-1H-1,2,3-triazole (22) via CuSO4. To a solution of 8-(tert-butylperoxy)-1-octyne 2 (301.6 mg, 1.52 mmol) and 3-azidopropylbenzene 16 (260.5 mg, 1.62 mmol, 1.1 equiv.) in CH2Cl2 (4 mL) was added 2 mL of an aqueous solution containing CuSO4 (50.7 mg, 0.32 mmol, 0.2 equiv.) and sodium ascorbate (65.4 mg, 0.33 mmol, 0.2 equiv.).12,29 After stirring at rt for 3 h, the reaction was diluted with water (10 mL) and extracted with EA (15 mL × 3). The combined organic layers were dried with Na2SO4, concentrated under reduced pressure and the residue purified by column chromatography (10% EA/Hex) to yield 223.9 mg (41%) of 4-(6-(tert-butylperoxy)hexyl)-1-(3-phenylpropyl)-1H-1,2,3-triazole (22) accompanied by 139.0 mg (37%) of aldehyde 24.
4-(6-(tert-Butylperoxy)hexyl)-1-(3-phenylpropyl)-1H-1,2,3-triazole (22) in presence of silane. To a solution of 8-(tert-butylperoxy)-1-octyne 2 (263.8 mg, 1.33 mmol) and 3-azidopropylbenzene 16 (261.0 mg, 1.34 mmol, 1.0 equiv.) in THF (5 mL) was added CuI (53.1 mg, 0.28 mmol, 0.2 equiv.),12 followed by triethylamine (0.60 mL, 4.3 mmol, 3 equiv.) and triethylsilane (0.45 mL, 2.50 mmol, 1.9 equiv.). The reaction was stirred at room temperature for 24 hours. The reaction was diluted with water (10 mL) and extracted with EA (15 mL × 3). The combined organic layers were dried with Na2SO4, concentrated under reduced pressure and the residue purified by column chromatography (10% EA/Hex) to yield 239.0 mg (50%) of 4-(6-(tert-butylperoxy)hexyl)-1-(3-phenylpropyl)-1H-1,2,3-triazole as a yellow oil (spectra described previously).
4-(6-(tert-Butylperoxy)hexyl)-5-iodo-1-(3-phenylpropyl)-1H-1,2,3-triazole (22i) from iodoalkyne 3. To a solution of 1-iodo-8-tert-butylperoxy octyne 4 (297.2 mg, 0.917 mmol) and 3-azidopropylbenzene 16 (159.0 mg, 0.99 mmol, 1.1 equiv.) in THF (6 mL) was added CuI (28.3 mg, 0.15 mmol, 0.2 equiv.), followed by triethylamine (0.30 mL, 2.15 mmol, 2 equiv.).27c The reaction was stirred at room temperature for 24 hours. The reaction was diluted with water (10 mL) and extracted with EA (15 mL × 3). The combined organic layers were dried with Na2SO4, concentrated under reduced pressure and the residue purified by column chromatography (10% EA/Hex) to yield 338.4 mg (76%) of iodotriazole 22i as a yellow oil (described previously).
4-(6-(tert-Butylperoxy)hexyl)-1-(4-phenylbutyl)-1H-1,2,3-triazole (23), 4-(6-(tert-butylperoxy)hexyl)-5-iodo-1-(4-phenylbutyl)-1H-1,2,3-triazole (23i) and 6-(1-(4-phenylbutyl)-1H-1,2,3-triazol-4-yl)hexanal (25). To a solution of 8-(tert-butylperoxy)-1-octyne 2 (305.4 mg, 1.54 mmol) and 4-azidobutylbenzene 17 (277.0 mg, 1.58 mmol, 1.0 equiv.) in THF (6 mL) was added CuI (64.2 mg, 0.34 mmol, 0.2 equiv.), followed by triethylamine (0.65 mL, 4.66 mmol, 3 equiv.). The reaction was stirred at room temperature for 24 hours. The reaction was diluted with water (10 mL) and extracted with EA (15 mL × 3). The combined organic layers were dried with Na2SO4, concentrated under reduced pressure and the residue purified by column chromatography (10% EA/Hex) to yield 315.7 mg (55%) of 29.8 mg (4%) of iodotriazole 23i, 315.7 mg (55%) of triazole 23 and 134.6 mg (45%) of aldehyde 25.23: Rf: 0.28 (40% EA/Hex): 1H δ 7.30 (t, J = 7.3, 2H), 7.22 (s, 1H), 7.21 (t, J = 7.5, 1H), 7.16 (d, J = 7.4, 2H), 4.33 (t, J = 7.2, 2H), 3.94 (t, J = 6.6, 2H), 2.72 (t, J = 7.7, 2H), 2.67 (t, J = 7.5, 2H), 1.94 (p, J = 7.6, 2H), 1.58–1.72 (m, 6H), 1.40–1.41 (m, 4H), 1.26 (s, 9H); 13C δ 148.4, 141.6, 128.5, 128.5, 126.1, 120.5, 80.2, 75.1, 50.1, 35.3, 29.9, 29.5, 29.2, 28.3, 27.9, 26.5, 26.1, 25.7; IR: 2977, 2920, 2860, 1454, 1362; HRMS (ESI+, TOF) calcd for C22H35N3NaO2 [M + Na]+: 396.2627; found: 396.2612.
23i:27 Rf: 0.32 (40% EA/Hex) 1H δ 7.30 (t, J = 6.8, 2H), 7.22 (t, J = 7.2, 1H), 7.18 (d, J = 7.0, 2H), 4.37 (t, J = 7.3, 2H), 3.95 (t, J = 6.3, 2H), 2.64–2.70 (m, 4H), 1.96 (p, J = 7.0, 2H), 1.68–1.73 (m, 4H), 1.62 (p, J = 6.5, 2H), 1.38–1.46 (m, 4H), 1.26 (s, 9H); 13C δ 151.9, 141.6, 128.5, 128.5, 126.1, 80.2, 77.9, 75.1, 50.6, 35.2, 29.8, 29.5, 29.1, 29.0, 28.1, 27.9, 26.5, 26.1; IR: 2088, 2920, 2858, 1454, 1361; HRMS (ESI+, TOF) calcd for C22H34IN3NaO2 [M + Na]+: 522.1593; found: 522.1581.
25: Rf: 0.27 (40% EA/Hex); 1H δ 9.77 (t, J = 1.7, 1H), 7.30 (t, J = 7.4, 2H), 7.22 (s, 1H), 7.21 (t, J = 7.4, 1H), 7.16 (d, J = 7.4, 2H), 4.33 (t, J = 7.2, 2H), 2.73 (t, J = 7.6, 2H), 2.67 (t, J = 7.6, 2H), 1.94 (p, J = 7.7, 2H), 1.63–1.73 (m, 4H), 1.59 (p, J = 6.6, 2H), 1.37–1.45 (m, 4H); 13C (176 MHz) δ 202.8, 148.4, 141.6, 128.5, 128.5, 126.1, 120.5, 62.9, 50.1, 43.9, 35.3, 32.7, 29.9, 28.3, 25.5, 21.9; IR: 2920, 2858, 1721, 1454; HRMS (ESI+, TOF) calcd for C18H25N3NaO [M + Na]+: 322.1895′; found: 322.1882.
4-(6-(tert-Butylperoxy)hexyl)-1-(4-phenylbutyl)-1H-1,2,3-triazole (23) and 6-(1-(4-phenylbutyl)-1H-1,2,3-triazol-4-yl)hexanal (25). To a solution of 8-(tert-butylperoxy)-1-octyne 2 (305.1 mg, 1.54 mmol) and 4-azidobutylbenzene 17 (272.3 mg, 1.55 mmol, 1.0 equiv.) in CH2Cl2 (4 mL) was added a solution of CuSO4 (59.2 mg, 0.37 mmol, 0.2 equiv.) and sodium ascorbate (75.5 mg, 0.38 mmol, 0.2 equiv.) in water (2 mL).12 The reaction was stirred at room temperature for 24 hours. The reaction was diluted with water (10 mL) and extracted with EA (15 mL × 3). The combined organic layers were dried with Na2SO4, concentrated under reduced pressure and the residue purified by column chromatography (10% EA/Hex) to yield 225.7 mg (39%) of peroxy triazole 23 as a yellow oil accompanied by 231.4 mg (50%) of aldehyde 25.
Click reaction of peroxyacetals
To a solution of 4-((2-methylperoxypropan-2-yl)oxy)-1-butyne 8 (166 mg, 1.05 mmol) and 4-azidobutylbenzene 17 (193 mg, 1.10 mmol) in THF (6 mL) was added CuI (38.1 mg, 0.20 mmol, 0.2 equiv.), followed by triethylamine (0.28 mL, 2.01 mmol, 3 equiv.). The reaction was stirred at room temperature for 1.5 hours. The reaction was diluted with water (10 mL) and extracted with EA (15 mL × 3). The combined organic layers were dried with Na2SO4, concentrated under reduced pressure and the residue purified by column chromatography (10% EA/Hex) to yield a light yellow oil as a nearly inseparable mixture of 4-(2-((2-(methylperoxy)propan-2-yl)oxy)ethyl)-1-(4-phenylbutyl)-1H-1,2,3-triazole and 4-(2-acetoxyethyl)-1-(4-phenylbutyl)-1H-1,2,3-triazole. The peroxyacetal, typically formed in less than 10% yield, was contaminated with variable amounts of the ester.
4-(2-((2-(Methylperoxy)propan-2-yl)oxy)ethyl)-1-(4-phenylbutyl)-1H-1,2,3-triazole. Rf = 0.34 (30% EA/Hex); 1H δ 7.32 (t, J = 7.1, 2H), 7.27 (s, 1H), 7.24 (t, J = 7.7, 1H), 7.23 (d, J = 7.5, 2H), 4.38 (t, J = 7.2, 2H), 3.86 (t, J = 7.6, 2H), 3.84 (s, 3H), 2.97 (t, J = 7.5, 2H), 2.71 (t, J = 7.3, 2H), 2.26 (p, J = 7.4, 2H), 1.28 (s, 6H); 13C δ 149.3, 140.4, 128.7, 128.6, 126.4, 104.9, 63.3, 60.7, 50.2, 32.6, 31.4, 29.8, 27.4, 23.2.
4-(4-((2-(Methylperoxy)propan-2-yl)oxy)butyl)1-(3-phenylpropyl)-1H-1,2,3-triazole (26). To a solution of 6-((2-methylperoxypropan-2-yl)oxy)-1-hexyne 10 (102 mg, 0.55 mmol) and 4-azidobutylbenzene 17 (98.1 mg, 0.56 mmol) in THF (5 mL) was added CuI (20.9 mg, 0.11 mmol, 0.2 equiv.), followed by triethylamine (0.23 mL, 1.7 mmol, 3 equiv.). The reaction was stirred at room temperature for 3 hours. The reaction was diluted with water (10 mL) and extracted with EA (15 mL × 3). The combined organic layers were dried with Na2SO4, concentrated under reduced pressure and the residue purified by column chromatography (10% EA/Hex) to yield 88.5 mg (45%) of the peroxyacetal/triazole 26 as a yellow oil: Rf = 0.28 (30% EA/Hex); 1H δ 7.32 (t, J = 7.3, 2H), 7.27 (s, 1H), 7.23 (t, J = 7.4, 1H), 7.19 (d, J = 7.2, 2H), 4.33 (t, J = 7.1, 2H), 3.85 (s, 3H), 3.56 (t, J = 6.5, 2H), 2.77 (t, J = 7.5, 2H), 2.67 (t, J = 7.5, 2H), 2.25 (p, J = 7.3, 2H), 1.82–1.56 (m, 4H), 1.40 (s, 6H); 13C δ 148.0, 140.4, 128.7, 128.6, 126.4, 120.7, 104.7, 63.3, 61.3, 49.5, 32.7, 31.8, 29.6, 26.2, 25.6, 23.2; IR: 3025, 2926, 2858, 2202, 1736, 1454, 1365, 1207, 1066, 748, 701; HRMS (ESI+, TOF) calcd for C19H29N3NaO30. [M + Na]+: 370.2107; found: 370.2103.
5-Iodo-4-(4-((2-(methylperoxy)propan-2-yl)oxy)butyl)-1-(3-phenylpropyl)-1H-1,2,3-triazole (27i). To a solution of 1-iodo-6-((2-methylperoxypropan-2-yl)oxy)-1-hexyne 11 (172 mg, 0.55 mmol) and 3-azidopropylbenzene 16 (96.7 mg, 0.60 mmol) in THF (5 mL) was added CuI (10.5 mg, 0.055 mmol, 0.1 equiv.), followed by triethylamine (0.15 mL, 1.08 mmol, 2 equiv.). The reaction was stirred at room temperature for 24 hours. The reaction was diluted with water (10 mL) and extracted with EA (15 mL × 3). The combined organic layers were dried with Na2SO4, concentrated under reduced pressure and the residue purified by column chromatography (10% EA/Hex) to yield 84.5 mg (33%) of peroxyacetal/iodotriazole 27i as a light yellow oil: Rf = 0.31 (30% EA/Hex); 1H δ 7.31 (t, J = 7.0, 2H), 7.23 (t, J = 7.0, 1H), 7.21 (d, J = 7.7), 4.36 (t, J = 7.2, 2H), 3.85 (s, 3H), 3.56 (t, J = 6.6, 2H), 2.70 (t, J = 7.6, 2H), 2.25 (p, J = 7.4, 2H), 1.84–1.62 (m, 4H), 1.40 (s, 6H); 13C δ 151.7, 140.4, 128.6, 128.5, 126.4, 104.7, 78.1, 63.3, 61.3, 50.1, 32.6, 31.3, 29.5, 26.0, 25.7, 23.2; IR: 3356, 3026, 2934, 2860, 1733, 1453, 1210, 1028, 745, 699; HRMS (ESI+, TOF) calcd for C15H20IN3NaO [(M − C4H8O2) + Na]+; elim. with loss of peroxyacetal]+: 408.0549; found: 408.0549.
Click reaction with azidoalkyl peroxides
4-Butyl-1-(4-(tert-butylperoxy)butyl)-1H-1,2,3-triazole (28). To a solution of 1-azido-(4-(tert-butylperoxy)butane 12 (142 mg, 0.76 mmol) and 1-hexyne 14 (65.7 mg, 0.80 mmol) in THF (3.5 mL) was added CuI (30.5 mg, 0.16 mmol, 0.2 equiv.), followed by triethylamine (0.32 mL, 2.30 mmol, 3 equiv.). After stirring for 3.5 hours, the reaction was diluted with water (10 mL). The combined EA extracts (15 mL × 3) were dried with Na2SO4, and concentrated under reduced pressure. The residue was purified by column chromatography (10% EA/Hex) to yield 69.5 mg (34%) of peroxy triazole 28 as a light yellow oil: Rf = 0.25 (40% EA/Hex); 1H δ 7.26 (s, 1H), 4.34 (t, J = 7.2, 2H), 3.95 (t, J = 6.2, 2H), 2.70 (t, J = 7.7, 2H), 1.98 (p, J = 7.5, 2H), 1.64 (p, J = 7.4, 2H), 1.62 (p, J = 7.4, 2H), 1.37 (tt, J = 14.9, J = 7.4, 2H), 1.22 (s, 9H), 0.92 (t, J = 7.3, 3H); 13C δ 148.5, 120.6, 80.4, 74.2, 50.0, 31.7, 27.5, 26.4, 25.5, 25.1, 22.4, 14.0; IR: 3133, 2931, 2097, 1458, 1362, 1196, 1046, 880; HRMS (ESI+, TOF) calcd for C14H27N3NaO2 [M + Na]+: 292.2001; found: 292.2003.
4-(4-(tert-Butylperoxy)butyl)-1-(4-phenylbutyl)-1H-1,2,3-triazole (29). By a similar procedure as for synthesis of 28 (above), reaction of a THF solution (3.5 mL) of 1-azido-(4-(tert-butylperoxy)butane 12 (140 mg, 0.75 mmol) and 5-hexynyl benzene 15 (125 mg, 0.79 mmol), CuI (30.5 mg, 0.16 mmol, 0.2 equiv.), and triethylamine (0.32 mL, 2.30 mmol, 3 equiv.) furnished 38.5 mg (15%) of peroxy triazole 29 as a light yellow oil: Rf = 0.23 (40% EA/Hex); 1H δ 7.31–7.17 (m, 6H), 4.39 (t, J = 7.3, 2H), 3.99 (t, J = 6.2, 2H), 2.77–2.64 (m, 4H), 2.02 (m, 2H), 1.83–1.61 (m, 6H), 1.26 (s, 9H); 13C δ 152.1, 142.5, 128.6, 128.5, 128.4, 125.8, 80.3, 74.0, 50.6, 35.7, 31.0, 28.7, 27.0, 26.5, 26.1, 25.1; IR: 3026, 2925, 2155, 2032, 1454, 1362, 1196, 699; HRMS (ESI+, TOF) calcd for C20H31N3NaO2 [M + Na]+: 368.2314; found: 368.2313.
4-Butyl-1-(4-(tert-butylperoxy)butyl)-1H-1,2,3-triazole (28, CuSO4 catalyst). To a solution of azidoperoxide 12 (0.80 mmol) and 1-hexyne 14 (0.9 mmol) in CH2Cl2 (3.5 mL) was added a solution of CuSO4 (0.16 mmol, 0.2 equiv.) and sodium ascorbate (0.16 mmol, 0.2 equiv.) in water (1.5 mL). After stirring for 24 h, the reaction was diluted with water. The combined ethyl acetate extracts (3 × 15 mL) were dried with Na2SO4, concentrated under reduced pressure and purified by column chromatography (10% EA/Hex) to yield 61.7 mg (29%) of peroxy triazole 28 as a light yellow oil: Rf = 0.25 (40% EA/Hex).
4-(4-(tert-Butylperoxy)butyl)-1-(4-phenylbutyl)-1H-1,2,3-triazole (29, CuSO4 catalyst). By a similar procedure as described above for synthesis of 28, reaction of azidoperoxybutane 12 and hexynyl benzene (15) with CuSO4 and sodium ascorbate in CH2Cl2 water furnished 7.1 mg (5%) of peroxy triazole 29.
Unsuccessful click reaction involving silylperoxy/azide 13
Reaction of peroxide 13 with alkynes 14 or 15 in the presence of 20% CuI/Et3N under conditions similar to those described earlier furnished a mixture of peroxide decomposition products. The same reaction, when conducted in the presence of 20% CuSO4/sodium ascorbate resulted in no detectable reaction (TLC) and extensive recovery of 13.
Preparation of functionalized nanoparticles
Synthesis of pentanethiolate functionalized gold nanoparticles was adapted from a reported procedure.38a An aqueous solution of hydrogen tetrachloroaurate (10 mL, 30 mmol, 30 mM) was vigorously stirred with a toluene solution of tetraoctylammonium bromide (27 mL, 50 mmol, 50 mM) until the tetrachloroaurate was transferred into the organic toluene layer. Pentanethiol (42 mg) was then added to the organic phase, followed by the addition of freshly prepared aqueous solution of sodium borohydride (8.33 mL, 3.3 mmol, 0.4 M). After the reaction had stirred for 3 hours at room temperature, the organic layer was separated and concentrated under reduced pressure to a volume of ∼1 mL. The concentrated layer was mixed with 125 mL of methanol and held at −18 C for 4 hours. The resulting solution was centrifuged. The nanoparticle-containing pellet was resuspended (methanol, 25 mL) and again centrifuged (3×) to yield (73.1 mg) a brown precipitate: 1H δ 1.32 (4H), 0.92 (3H);39 IR: 2956, 2921; elemental analysis: Au, 50.1; C, 43.9; S, 6.0%; XPS (binding energies): Au 4f7/2 (82.6 eV), Au 4f5/2 (86.4 eV), C 1s (284.3 eV), S 2p (161.2 eV). Solutions of the nanoparticles displayed a broad absorption in the following paragraph, a dodecanethiol-passivated set of nanoparticles prepared as a standard, and which did display the small 520 nm bump, otherwise exhibited identical properties to the C5 nanoparticles.71
Dodecanethiolate functionalized gold nanoparticles. Synthesis of dodecanethiolate functionalized gold nanoparticles were prepared following a reported procedure.38a An aqueous solution of hydrogen tetrachloroaurate (10 mL, 30 mmol, 30 mM) was vigorously stirred with a toluene solution of tetraoctylammonium bromide (27 mL, 50 mmol, 50 mM) until the tetrachloroaurate was transferred into the organic toluene layer. Dodecanethiol (57 mg) was then added to the organic phase, followed by the addition of freshly prepared aqueous solution of sodium borohydride (8.33 mL, 3.3 mmol, 0.4 M). The reaction was stirred for 3 hours at room temperature. After 3 hours of stirring at room temperature, the organic layer was separate, and concentrated under reduced pressure to 1 mL, and mixed with 125 mL of methanol to remove excess thiol and kept at −18 C for 4 hours. The solution was centrifuged and washed with methanol (25 mL × 3) to yield (73.1 mg) a black precipitate: 1H δ 1.27 (10H), 0.91 (3H); IR: 2956, 2920; UV-vis: 520.65 nm (diagnostic signal; see ESI†); elemental analysis: Au, 78.4; C, 17.7; S, 3.9%; XPS (binding energies): Au 4f7/2 (82.9 eV), Au 4f5/2 (86.6 eV), C 1s (283.8 eV), S 2p (161.0 eV).Azide functionalized gold nanoparticles (N3Au) were prepared via a modification of reported procedures.36 To a solution of C5SH-Au P (47.9 mg) in CH2Cl2 (5 mL) was added 6-azidohexane-1-thiol (142.5 mg). After stirring for 3 days at room temperature under nitrogen, the reaction solution was concentrated under reduced pressure and pelleted/resuspended (6 × 10 mL, methanol) to yield (62.3 mg) a brown precipitate: 1H δ 3.31 (2H), 1.45 (2H), 1.28 (4H), 0.87 (3H); IR: 2922, 2851, 2094; elemental analysis: Au, 54.0; C, 34.2; S, 8.5; N, 3.3%; XPS (binding energies): Au 4f7/2 (83.1 eV), Au 4f5/2 (86.9 eV), C 1s (283.9 eV), S 2p (162.0 eV), N 1s (398.3 eV).
Peroxide functionalized gold nanoparticles (PeroxideAu) were prepared via a modification of reported procedures.37 To a solution of 6-(tert-butylperoxy)-1-hexyne (182.9 mg, 1.07 mmol) and azidoalkylthiolate Au-NP (23.4 mg) in THF (5 mL) was added CuI (41.7 mg, 0.22 mmol, 0.2 equiv. per alkyne), followed by triethylamine (0.50 mL, 3.6 mmol, 3 equiv. per alkyne). The reaction was stirred at room temperature for 8 hours. The reaction was diluted with saturated aqueous solution of NH4Cl (10 mL) and the organic layer was washed with water (10 mL × 3). The organic layer was dried with Na2SO4, concentrated under reduced pressure and washed with methanol (10 mL × 6) to remove excess alkyne to yield (29.8 mg) a light brown precipitate: 1H δ 3.99 (2H), 2.47 (2H), 1.60–1.75 (8H), 1.26–1.30 (11H), 0.88 (1.5 H) IR: 2975, 2926, 2865, 1723, 1559, 1463, 1362, 1022, 803; elemental analysis: Au, 26.4; C, 49.9; S, 4.7; N, 5.1; O, 13.9%; XPS (binding energies): Au 4f7/2 (84.0 eV), Au 4f5/2 (87.8 eV), C 1s (284.3 eV), S 2p (162.9 eV), N 1s (399.6 eV), O 1s (531.5 eV).
Peroxyacetal functionalized gold nanoparticles (PeroxyacetalAu). To a solution of 6-((2-peroxypropan-2-yl)oxy)-1-hexyne (197.9 mg, 1.06 mmol) and azidoalkylthiolate Au-NP (28.2 mg) in THF (5 mL) was added CuI (41.4 mg, 0.22 mmol, 0.2 equiv. per alkyne), followed by triethylamine (0.50 mL, 3.6 mmol, 3 equiv. per alkyne). The reaction was stirred at room temperature for 8 hours. The reaction was diluted with saturated aqueous solution of NH4Cl (10 mL) and the organic layer was washed with water (10 mL × 3). The organic layer was dried with Na2SO4, concentrated under reduced pressure and washed with methanol (10 mL × 6) to remove excess alkyne to yield (14.1 mg) a yellow precipitate: 1H δ 3.86 (2H), 2.05 (2H), 1.67 (2H), 1.57 (6H), 1.39 (5 H), 1.28 (4H), 1.12 (4H), 0.91 (5H); IR: 3411, 2921, 2851, 1738, 1240, 1065; elemental analysis: Au, 20.0; C, 52.6; S, 4.3; N, 4.6; O, 18.5%; XPS (binding energies): Au 4f7/2 (83.9 eV), Au 4f5/2 (87.6 eV), C 1s (284.0 eV), S 2p (163.2 eV), N 1s (399.3 eV), O 1s (531.5 eV).
Abbreviations
Hexane(s) | Hex |
EA | Ethyl acetate |
THF | Tetrahydrofuran |
DMF | N,N-Dimethylformamide |
DMSO | Dimethyl sulfoxide |
EtOH | Ethanol |
MeOH | Methanol |
TEA | Triethylamine |
Na ascorb. | Sodium ascorbate |
TBHP | tert-Butyl hydroperoxide |
TBTA | Tris[(1-benzyl-1H-1,2,3-triazol-4-yl)methyl]amine |
Co(acac)2 | Cobalt(II) acetylacetonate |
Conflicts of interest
There are no conflicts of interest to declare.
Acknowledgements
Funding was provided by NSF (CHE 1464914) and by Dr In Quen Lee. Materials characterization was performed in part in the Nebraska Nanoscale Facility: National Nanotechnology Coordinated Infrastructure and the Nebraska Center for Materials and Nanoscience, facilities supported by NSF (ECCS 1542182) and the Nebraska Research Initiative. We thank Prof. Martha Morton, Ms Nataliia Vorobyova, Dr Moriah Locklear, and Mr Boone Evans for technical assistance and/or insightful suggestions.
References
-
(a) I. A. Yaremenko, V. A. Vil, D. V. Demchuk and A. O. Terent'ev, Rearrangements of organic peroxides and related processes, Beilstein J. Org. Chem., 2016, 12, 1647–1748 Search PubMed;
(b) P. Dussault, Reactions of Hydroperoxides and Peroxides, in Active Oxygen In Chemistry, Chapman & Hall, London, 1995, pp. 141–203 Search PubMed;
(c) The Chemistry of Peroxides, ed. J. F. Liebman and A. Greer, Wiley, 2014, vol. 3, p. 1120, ISBN: 978-1-118-41271-8 Search PubMed.
- For example, see: A. Banerjee and H. Yamamoto, Direct N–O bond formation via oxidation of amines with benzoyl peroxide, Chem. Sci., 2019, 10, 2124–2129 Search PubMed.
- G. O. Wilson, J. W. Henderson, M. M. Caruso, B. J. Blaiszik, P. J. McIntire, N. R. Sottos, S. R. White and J. S. Moore, Evaluation of peroxide initiators for radical polymerization-based self-healing applications, J. Polym. Sci., Part A: Polym. Chem., 2010, 48, 2698–2708 Search PubMed.
- P. M. O'Neill, V. E. Barton and S. A. Ward, The molecular mechanism of action of artemisinin—the debate continues, Molecules, 2010, 15, 1705–1721 Search PubMed.
- P. H. Dussault, A. D. George and T. K. Trullinger, Peroxides as oxidative enzyme inhibitors: mechanism-based inhibition of a cysteine protease by an amino acid ozonide, Bioorg. Med. Chem. Lett., 1999, 9, 3255–3258 Search PubMed.
- S. Kyasa, R. N. Meier, R. A. Pardini, T. K. Truttmann, K. T. Kuwata and P. H. Dussault, Synthesis of Ethers via Reaction of Carbanions and Monoperoxyacetals, J. Org. Chem., 2015, 80, 12100–12114 Search PubMed.
-
(a) N. A. Porter, A perspective on free radical autoxidation: the physical organic chemistry of polyunsaturated fatty acid and sterol peroxidation, J. Org. Chem., 2013, 78, 3511–3524 Search PubMed;
(b) A. Bodin, M. Linnerborg, J. L. G. Nilsson and A.-T. Karlberg, Structure Elucidation, Synthesis, and Contact Allergenic Activity of a Major Hydroperoxide Formed at Autoxidation of the Ethoxylated Surfactant C12E5, Chem. Res. Toxicol., 2003, 16, 575–582 Search PubMed.
-
(a) T. M. McIntire, O. Ryder and B. Finlayson-Pitts, Secondary ozonide formation from the ozone oxidation of unsaturated self-assembled monolayers on zinc selenide attenuated total reflectance crystals, J. Phys. Chem. C, 2009, 113, 11060–11065 Search PubMed;
(b) A. Razgon, R. G. Bergman and C. N. Sukenik, Ozonolysis-based route to the in situ formation of aldehyde-bearing self-assembled monolayer surfaces, Langmuir, 2008, 24, 2545–2552 Search PubMed.
-
(a) H. X. Jin, H. H. Liu, Q. Zhang and Y. Wu, On the susceptibility of organic peroxy bonds to hydride reduction, J. Org. Chem., 2005, 70, 4240–4247 Search PubMed;
(b) I. Opsenica, D. Opsenica, K. S. Smith, W. K. Milhous and B. A. Šolaja, Chemical stability of the peroxide bond enables diversified synthesis of potent tetraoxane antimalarials, J. Med. Chem., 2008, 51, 2261–2266 Search PubMed;
(c) P. Dussault, The peroxide changes everything: new methodology for the synthesis of peroxide-containing natural products, Synlett, 1995, 997–1003 Search PubMed;
(d) H. O'Dowd, P. Ploypradith, S. Xie, T. A. Shapiro and G. H. Posner, Antimalarial artemisinin analogs. Synthesis via chemoselective C-C bond formation and preliminary biological evaluation, Tetrahedron, 1999, 55, 3625–3636 Search PubMed;
(e) P. H. Dussault and C. T. Eary, Palladium-Mediated Carbon– Carbon Bond Forming Reactions as a New Method for the Synthesis of Peroxides and Hydroperoxides, J. Am. Chem. Soc., 1998, 120, 7133–7134 Search PubMed.
-
(a) J. Zabicky, Analytical and Safety Aspects of Organic Peroxides and Related Functional Groups, in The Chemistry of the Peroxide Group, ed. Z. Rappoport, John Wiley & Sons, Chichester, 2006, vol. 2, pt 2, pp. 597–773; ISBN: 978-0-470-86274-2 Search PubMed;
(b) D. E. Clark, Peroxides and Peroxide-forming Compounds, J. Chem. Health Saf., 2001, 8, 12–21 Search PubMed;
(c) G. D. Kozak, A. N. Tsvigunov and N. I. Akinin, Similarities and Differences in Explosion Hazards of Organic Peroxides
and High Explosives: an Experimental Study, Cent. Eur. J. Energ. Mater., 2011, 8, 249–260 Search PubMed;
(d) P. H. Dussault, Working with Organic Peroxides in the Academic Lab, Department of Chemistry, University of Nebraska-Lincoln; Digital Commons@University of Nebraska Lincoln; DOI:10.13014/K2GT5KCJ; accessed July 2020.
-
(a) C. S. McKay and M. G. Finn, Click chemistry in complex mixtures: bioorthogonal bioconjugation, Chem. Biol., 2014, 21, 1075–1101 Search PubMed;
(b) W. H. Binder and R. Sachsenhofer, ‘Click’ chemistry in polymer and materials science, Macromol. Rapid Commun., 2007, 28, 15–54 Search PubMed;
(c) J. E. Moses and A. D. Moorhouse, The growing applications of click chemistry, Chem. Soc. Rev., 2007, 36, 1249–1262 Search PubMed;
(d) H. C. Kolb and K. B. Sharpless, The growing impact of click chemistry on drug discovery, Drug Discovery Today, 2003, 8, 1128–1137 Search PubMed;
(e) R. Chelmowski, D. Kafer, S. D. Köster, T. Klasen, T. Winkler, A. Terfort, N. Metzler-Nolte and C. Wöll, Postformation modification of SAMs: using click chemistry to functionalize organic surfaces, Langmuir, 2009, 25, 11480–11485 Search PubMed.
-
(a) M. Meldal and C. W. Tornøe, Cu-catalyzed azide– alkyne cycloaddition, Chem. Rev., 2008, 108, 2952–3015 Search PubMed;
(b) J. E. Hein and V. V. Fokin, Copper-catalyzed azide-alkyne cycloaddition and beyond: new reactivity of copper acteylides, Chem. Soc. Rev., 2011, 39, 1302–1315 Search PubMed;
(c) L. Zhu, C. J. Brassard, X. Zhang, P. M. Guha and R. J. Clark, On the Mechanism of Copper(I)-Catalyzed Azide–Alkyne Cycloaddition, Chem. Rec., 2016, 16, 1501–1517 Search PubMed.
-
(a) S. Jana, S. Iram, J. Thomas, S. Liekens and W. Dehaen, Synthesis and anticancer activity of novel aza-artemisinin derivatives, Bioorg. Med. Chem., 2017, 25, 3671–3676 Search PubMed;
(b) H. M. Ismail, V. E. Barton, M. Panchana, S. Charoensutthivarakul, G. A. Biagini, S. A. Ward and P. M. O'Neill, A Click Chemistry-Based Proteomic Approach Reveals that 1,2,4-Trioxolane and Artemisinin Antimalarials Share a Common Protein Alkylation Profile, Angew. Chem., Int. Ed., 2016, 55, 6401–6405 Search PubMed;
(c) W. Li, Y. Zhou, G. Tang and Y. Xiao, Characterization of the Artemisinin Binding Site for Translationally Controlled Tumor Protein (TCTP) by Bioorthogonal Click Chemistry, Bioconjugate Chem., 2016, 27, 2828–2833 Search PubMed;
(d) L. H. Binh, N. T. T. Van, V. T. Kien, N. T. T. My, L. Van Chinh, N. T. Nga, H. X. Tien, D. T. Thao and T. K. Vu, Synthesis and in vitro cytotoxic evaluation of new triazole derivatives based on artemisinin via click chemistry, Med. Chem. Res., 2016, 25, 738–750 Search PubMed.
-
(a) Y. Chen, J. Q. Ren, X. G. Zhang, D. Y. Wu, A. G. Shen and J. M. Hu, Alkyne-Modulated Surface-Enhanced Raman Scattering-Palette for Optical Interference-Free and Multiplex Cellular Imaging, Anal. Chem., 2016, 88, 6115–6119 Search PubMed;
(b) R. Kozlowski, A. Ragupathi and R. B. Dyer, Characterizing the Surface Coverage of Protein-Gold Nanoparticle Bioconjugates, Bioconjugate Chem., 2018, 29, 2691–2700 Search PubMed;
(c) S. Liu and M. Han, Synthesis, Functionalization, and Bioconjugation of Monodisperse, Silica-Coated Gold Nanoparticles: Robust Bioprobes, Adv. Funct. Mater., 2005, 15, 961–967 Search PubMed.
- X.-R. Jiang, P. Wang, C. L. Smith and B. T. Zhu, Synthesis of Novel Estrogen Receptor Antagonists Using Metal-Catalyzed Coupling Reactions and Characterization of Their Biological Activity, J. Med. Chem., 2013, 56, 2779–2790 Search PubMed.
- P. Dussault and A. Sahli, 2-Methoxy-2-propyl hydroperoxide: a convenient reagent for the synthesis of hydroperoxides and peracids, J. Org. Chem., 1992, 57, 1009–1012 Search PubMed.
- K. Rajender Reddy, M. Venkateshwar, C. Uma Maheswari and P. Santhosh Kumar, Mild and efficient oxy-iodination of alkynes and phenols with potassium iodide and tert-butyl hydroperoxide, Tetrahedron Lett., 2010, 51, 2170–2173 Search PubMed.
-
(a) Y. Yamamoto, E. Niki and Y. Kamiya, Ozonization of Organic Compounds. Relative Reactivity of Protic Solvents Toward Carbonyl Oxide, Bull. Chem. Soc. Jpn., 1982, 55, 2405–2406 Search PubMed;
(b) T. Fisher and P. H. Dussault, Alkene Ozonolysis, Tetrahedron, 2017, 73, 4233–4258 Search PubMed.
- P. H. Dussault and D. R. Davies, Synthesis of 1,2-dioxanes, 1,2,4-trioxanes, and 1,2,4-trioxepanes via cyclizations of unsaturated hydroperoxyacetals, Tetrahedron Lett., 1996, 37, 463–466 Search PubMed.
- The pKa of propargyl alcohol is 13.2. Trifluoroethanol (pKa ∼ 12.4) has been employed as a nonnucleophilic polar solvent for ozonolysis: K. J. McCullough, H. Tokuhara, A. Masuyama and M. Nojima, New approaches to the synthesis of spiro-peroxylactones, Org. Biomol. Chem., 2003, 1, 1522–1527 Search PubMed.
- Y. Hamada, H. Tokuhara, A. Masuyama, M. Nojima, H. S. Kim, K. Ono, N. Ogura and Y. Wataya, Synthesis and notable antimalarial activity of acyclic peroxides, 1-(alkyldioxy)-1-(methyldioxy) cyclododecanes, J. Med. Chem., 2002, 45, 1374–1378 Search PubMed.
- L. Yao, B. T. Smith and J. Aubé, Base-Promoted Reactions of Bridged Ketones and 1,3- and 1,4-Haloalkyl Azides: Competitive Alkylation vs. Azidation Reactions of Ketone Enolates, J. Org. Chem., 2004, 69, 1720–1722 Search PubMed.
-
(a) S. Isayama and T. Mukaiyama, A New Method for Preparation of Alcohols from Olefins with Molecular Oxygen and Phenylsilane by the use of Bis(acetylacetonato)cobalt(II), Chem. Lett., 1989, 18, 1071–1074 Search PubMed;
(b) T. Tokuyasu, S. Kunikawa, A. Masuyama and M. Nojima, Co(III)-alkyl complex- and Co(III)-alkylperoxo complex-catalyzed triethylsilylperoxidation of alkenes with molecular oxygen and triethylsilane, Org. Lett., 2002, 4, 3595–3598 Search PubMed.
-
(a) Organic azides: syntheses and applications, ed. S. Bräse and K. Banert, John Wiley & Sons, 2010 Search PubMed;
(b) T. M. Klapötke, T. Rotariu, B. Stiasny, J. Stierstorfer, S. Wiegmann and T. Zecheru, Azido (tert-butylperoxy) methyl Compounds–An Exceptional Class of Energetic Materials, Eur. J. Org. Chem., 2016, 4382–4386 Search PubMed.
-
(a) F. Himo, T. Lovell, R. Hilgraf, V. V. Rostovtsev, L. Noodlesman, K. B. Sharpless and V. V. Fokin, Copper(I)-Catalyzed Synthesis of Azoles. DFT Study Predicts Unprecedented Reactivity and Intermediates, J. Am. Chem. Soc., 2005, 127, 210–216 Search PubMed;
(b) P. Wu and V. V. Fokin, Catalytic Azide-Alkyne Cycloaddition: Reactivity and Applications, Aldrichimica Acta, 2007, 40, 7–17 Search PubMed.
- L. L. Smith and F. L. Hill, Detection of sterol hydroperoxides on thin-layer chromatoplates by means of the Würster dyes, J. Chromatogr., 1972, 66, 101–109 Search PubMed.
-
(a) D. N. Barsoum, N. Okashah, X. Zhang and L. Zhu, Mechanism of Copper(I)-Catalyzed 5-Iodo-1,2,3-triazole Formation from Azide and Terminal Alkyne, J. Org. Chem., 2015, 80, 9542–9551 Search PubMed;
(b) D. N. Barsoum, C. J. Brassard, J. H. A. Deeb, N. Okashah, K. Sreenath, J. T. Simmons and L. Zhu, Synthesis of 5-Iodo-1,2,3-triazoles from organic azides and terminal alkynes: Ligand Acceleration Effect, Substrate Scope, and Mechanistic Insights, Synthesis, 2013, 45, 2372–2386 Search PubMed;
(c) J. E. Hein, J. C. Tripp, L. B. Krasnova, K. B. Sharpless and V. V. Fokin, Copper (I)-catalyzed cycloaddition of organic azides and 1-iodoalkynes, Angew. Chem., Int. Ed., 2009, 48, 8018–8021 Search PubMed.
- P. S. Donnelly, S. D. Zanatta, S. C. Zammit, J. M. White and S. J. Williams, “Click” cycloaddition catalysts: copper(i) and copper(ii) tris(triazolylmethyl)amine complexes, Chem. Commun., 2008, 2459 Search PubMed.
-
(a) B. T. Worrell, J. A. Malik and V. V. Fokin, Direct Evidence of a Dinuclear Copper Intermediate in Cu(I)-Catalyzed Azide-Alkyne Cycloadditions, Science, 2013, 80(340), 457–460 Search PubMed;
(b) R. Berg and B. F. Straub, Advancements in the mechanistic understanding of the copper-catalyzed azide–alkyne cycloaddition, Beilstein J. Org. Chem., 2013, 9, 2715–2750 Search PubMed.
- B. H. Lipshutz, W. Chrisman and K. Noson, Hydrosilylation of aldehydes and ketones catalyzed by [Ph3P(CuH)]6, J. Organomet. Chem., 2001, 624, 367–371 Search PubMed.
- N. Kornblum and H. E. DeLaMare, The base catalyzed decomposition of a di-alkyl peroxide, J. Am. Chem. Soc., 1951, 73, 880–881 Search PubMed.
-
(a) E. W. Elliott, A. L. Ginzburg, Z. C. Kennedy, Z. Feng and J. E. Hutchison, Single-Step Synthesis of Small, Azide-Functionalized Gold Nanoparticles: Versatile, Water-Dispersible Reagents for Click Chemistry, Langmuir, 2017, 33, 5796–5802 Search PubMed;
(b) D. Baranov and E. N. Kadnikova, Synthesis and characterization of azidoalkyl-functionalized gold nanoparticles as scaffolds for “click”-chemistry derivatization, J. Mater. Chem., 2011, 21, 6152 Search PubMed.
- Y. Zhou, S. Wang, K. Zhang and X. Jiang, Visual Detection of Copper(II) by Azide- and Alkyne-Functionalized Gold Nanoparticles Using Click Chemistry, Angew. Chem., Int. Ed., 2008, 47, 7454–7456 Search PubMed.
- J. L. Brennan, N. S. Hatzakis, T. R. Tshikhudo, N. Dirvianskyte, V. Razumas, S. Patkar, J. Vind, A. Svendsen, R. J. M. Nolte, A. E. Rowan and M. Brust, Bionanoconjugation via Click Chemistry: The Creation of Functional Hybrids of Lipases and Gold Nanoparticles, Bioconjugate Chem., 2006, 17, 1373–1375 Search PubMed.
- V. Poonthiyil, T. K. Lindhorst, V. B. Golovko and A. J. Fairbanks, Recent applications of click chemistry for the functionalization of gold nanoparticles and their conversion to glyco-gold nanoparticles, Beilstein J. Org. Chem., 2018, 14, 11–24 Search PubMed.
- P. Zhao, M. Grillaud, L. Salmon, J. Ruiz and D. Astruc, Click Functionalization of Gold Nanoparticles Using the Very Efficient Catalyst Copper(I) (Hexabenzyl)tris(2-aminoethyl)-amine Bromide, Adv. Synth. Catal., 2012, 354, 1001–1011 Search PubMed.
- E. Boisselier, L. Salmon, J. Ruiz and D. Astruc, How to very efficiently functionalize gold nanoparticles by “click” chemistry, Chem. Commun., 2008, 5788–5790 Search PubMed.
-
(a) M. Brust, M. Walker, D. Bethell, D. J. Schiffrin and R. Whyman, Synthesis of Thiol-derivatised Gold Nanoparticles in a Two-phase Liquid-Liquid System, Chem. Commun., 1994, 801–802 Search PubMed;
(b) M. Brust, J. Fink, D. J. Schiffrina, D. Bethella and C. Kielyb, Synthesis and Reactions of Functionalised Gold Nanoparticle, Chem. Commun., 1995, 1655–1656 Search PubMed.
- Due to limited freedom of rotation, hydrogens near the Au–S interface are often invisible to solution phase NMR.
(a) C. Guo and J. L. Yarger, Characterizing gold nanoparticles by NMR spectroscopy, Magn. Reson. Chem., 2018, 56, 1074–1082 Search PubMed;
(b) L. E. Marbella and J. E. Millstone, NMR Techniques for Noble Metal Nanoparticles, Chem. Mater., 2015, 27, 2721–2739 Search PubMed.
- Oxford Instruments, EDS in the TEM Explained (12 pages), 2013, https://nano.oxinst.com/product/eds-for-tem Search PubMed.
- X. Zhang, P. Liu and L. Zhu, Structural Determinants of Alkyne Reactivity in Copper-Catalyzed Azide-Alkyne Cycloadditions, Molecules, 2016, 21, 1–17 Search PubMed.
- A. Horn and P. H. Dussault, Synthesis of α-cyano and α-sulfonyl cyclic ethers via intramolecular reactions of peroxides with sulfone-and nitrile-stabilized carbanions, J. Org. Chem., 2019, 84, 14611–14626 Search PubMed.
- A. S. Olson, A. J. Jameson, S. K. Kyasa, B. W. Evans and P. H. Dussault, Reductive Cleavage of Organic Peroxides by
Iron Salts and Thiols, ACS Omega, 2018, 3, 14054–14063 Search PubMed.
- X. Wang, Y. Dong, S. Wittlin, D. Creek, J. Chollet, S. A. Charman, J. Santo Tomas, C. Scheurer, C. Snyder and J. L. Vennerstrom, Spiro-and dispiro-1,2-dioxolanes: contribution of iron (II)-mediated one-electron vs. two-electron reduction to the activity of antimalarial peroxides, J. Med. Chem., 2007, 50, 5840–5847 Search PubMed.
- D. A. Fleming, C. J. Thode and M. E. Williams, Triazole Cycloaddition as a General Route for Functionalization of Au Nanoparticles, Chem. Mater., 2006, 18, 2327–2334 Search PubMed.
- W. J. Sommer and M. Weck, Facile functionalization of gold nanoparticles via microwave-assisted 1,3 dipolar cycloaddition, Langmuir, 2007, 23, 11991–11995 Search PubMed.
-
(a) W. Limapichat and A. Basu, Reagentless functionalization of gold nanoparticles via a 3 + 2 Huisgen cycloaddition, J. Colloid Interface Sci., 2008, 318, 140–144 Search PubMed;
(b) C. J. Thode and M. E. Williams, Kinetics of 1,3-dipolar cycloaddition on the surfaces of Au nanoparticles, J. Colloid Interface Sci., 2008, 320, 346–352 Search PubMed.
- R. Cohen, Y. Mazuz, M. Tikhonov and C. M. Sukenik, Carboxylic Acid Decorated Self-Assembled Monolayer Films: New Acid Synthesis Chemistry and Reaction Chemistry Including Bridged Diacyl Peroxide Preparation, Langmuir, 2015, 31, 3049–3058 Search PubMed.
- R. Mello and M. E. González Núñez, Reactions of Peroxides on Solid Surfaces, in PATAI'S Chemistry of Functional Groups, ed. Z. Rappoport, DOI:10.1002/9780470682531.pat0880.
- K. L. Norrod and K. L. Rowlen, Ozone-induced oxidation of self-assembled decanethiol: contributing mechanism for “photooxidation”?, J. Am. Chem. Soc., 1998, 120(11), 2656–2657 Search PubMed.
-
(a) W. Liu, X. Liu, D. Knaebel, L. Luck and Y. Li, Synthesis and antibacterial evaluation of novel water-soluble organic peroxides, Antimicrob. Agents Chemother., 1998, 42, 911–915 Search PubMed;
(b) C. G. Burkhart and C. N. Burkhart, Antibacterial properties of soluble benzoyl peroxide, Int. J. Dermatol., 2008, 47, 301–302 Search PubMed.
- For an example of surface-specific peroxide chemistry involving an adsorbed peroxide, see: N. Walalawela and A. Greer, Heterogeneous photocatalytic deperoxidation with UV and visible light, J. Phys. Org. Chem., 2018, 31, e3807 Search PubMed.
- K. Tokumaru, Complications in photochemistry of organic peroxides, Res. Chem. Intermed., 1996, 22, 255–273 Search PubMed.
-
(a) R. Willand-Charnley, B. W. Puffer and P. H. Dussault, Oxacycle synthesis via intramolecular reaction of carbanions and peroxides, J. Am. Chem. Soc., 2014, 136, 5821–5823 Search PubMed;
(b) L. Lemee, M.-J. Bourgeois and E. Montaudon, Decomposition induced by acetylenic peroxides, Bull. Soc. Chim. Belg., 1996, 105, 467–472 Search PubMed.
- M. T. Wlodarczyk, S. A. Dragulska, O. Camacho-Vanegas, P. R. Dottino, A. A. Jarzęcki, J. A. Martignetti and A. J. Mieszawska, Platinum (II) complex-nuclear localization sequence peptide hybrid for overcoming platinum resistance in cancer therapy, ACS Biomater. Sci. Eng., 2018, 4, 463–467 Search PubMed.
-
(a) J. N. Eildal, A. Bach, J. Dogan, F. Ye, M. Zhang, P. Jemth and K. Strømgaard, Rigidified Clicked Dimeric Ligands for Studying the Dynamics of the PDZ1-2 Supramodule of PSD-95, ChemBioChem, 2015, 16, 64–69 Search PubMed;
(b) A. Krasiński, Z. Radić, R. Manetsch, J. Raushel, P. Taylor, K. B. Sharpless and H. C. Kolb, In situ selection of lead compounds by click chemistry: target-guided optimization of acetylcholinesterase inhibitors, J. Am. Chem. Soc., 2005, 127, 6686–6692 Search PubMed.
- P. H. Dussault, I. Q. Lee, H. J. Lee, R. J. Lee, Q. J. Niu, J. A. Schultz and U. R. Zope, Peroxycarbenium-mediated
C-C bond formation: applications to the synthesis of hydroperoxides and peroxides, J. Org. Chem., 2000, 65, 8407–8414 Search PubMed.
- J. E. Baldwin, L. Bischoff, T. D. W. Claridge, F. A. Heupel, D. R. Spring and R. C. Whitehead, An approach to the manzamine alkaloids modelled on a biogenetic theory, Tetrahedron, 1997, 53, 2271–2290 Search PubMed.
- R. J. Armstrong, W. Niwetmarin and V. K. Aggarwal, Synthesis of Functionalized Alkenes by a Transition-Metal-Free Zweifel Coupling, Org. Lett., 2017, 19, 2762–2765 Search PubMed.
- J. van der Louw, J. L. van der Baan, F. J. de Kanter, F. Bickelhaupt and G. W. Klumpp, Allylmetallation of 1-silylalkynes by 2-(bromozincmethyl)-2-alkenyl ethers followed by Pd (0)-catalyzed cyclization: a one-pot synthesis of 4-methylenecyclopentenes, Tetrahedron, 1992, 48, 6087–6104 Search PubMed.
- B. R. Brutiu, W. A. Bubeneck, O. Cvetkovic, J. Li and N. Maulide, On the formation of seven-membered rings by arene-ynamide cyclization, Monatsh. Chem., 2019, 150, 3–10 Search PubMed.
- M. Schmittel and C. Vavilala, Kinetic isotope effects in the thermal C2-C6 cyclization of enyne-allenes: experimental evidence supports a stepwise mechanism, J. Org. Chem., 2005, 70, 4865–4868 Search PubMed.
- J. M. Smith, T. Qin, R. R. Merchant, J. T. Edwards, L. R. Malins, Z. Liu, G. Che, Z. Shen, S. A. Shaw, M. D. Eastgate and P. S. Baran, Decarboxylative Alkynylation, Angew. Chem., Int. Ed., 2017, 56, 11906–11910 Search PubMed.
- J. Maury, L. Feray, M. P. Bertrand, A. Kapat and P. Renaud, Unexpected conversion of alkyl azides to alkyl iodides and of aryl azides to N–tert-butyl anilines, Tetrahedron, 2012, 68, 9606–9611 Search PubMed.
- S. M. Smith and J. M. Takacs, Amide-Directed Catalytic Asymmetric Hydroboration of Trisubstituted Alkenes, J. Am. Chem. Soc., 2010, 132, 1740–1741 Search PubMed.
- T. Suzuki, Y. Ota, Y. Kasuya, M. Mutsuga, Y. Kawamura, H. Tsumoto, H. Nakagawa, M. G. Finn and N. Miyata, An unexpected example of protein-templated click chemistry, Angew. Chem., Int. Ed., 2010, 49, 6817–6820 Search PubMed.
- K. Motesharei and D. C. Myles, Molecular Recognition on Functionalized Self-Assembled Monolayers of Alkanethiols on Gold, J. Am. Chem. Soc., 1998, 120, 7328–7336 Search PubMed.
- M. H. Keylor, J. E. Park, C. J. Wallentin and C. R. J. Stephenson, Photocatalytic initiation of thiol-ene reactions: synthesis of thiomorpholin-3-ones, Tetrahedron, 2014, 70, 4264–4269 Search PubMed.
- C. Ligeour, A. Meyer, J. J. Vasseur and F. Morvan, Bis- and Tris-Alkyne Phosphoramidites for Multiple 5′-Labeling of Oligonucleotides by Click Chemistry, Eur. J. Org. Chem., 2012, 1851–1856 Search PubMed.
- F. Gonzàlez De Rivera, I. Angurell, O. Rossell, M. Seco and J. Llorca, Organometallic surface functionalization of gold nanoparticles, J. Organomet. Chem., 2012, 715, 13–18 Search PubMed.
- W. Haiss, N. T. K. Thanh, J. Aveyard and D. G. Fernig, Determination of Size and Concentration of Gold Nanoparticles from UV-Vis Spectra, Anal. Chem., 2007, 79, 4215–4221 Search PubMed.
Footnote |
† Electronic supplementary information (ESI) available: 1H and 13C NMR spectra of molecules, as well as 1H, IR, and XPS characterization of functionalized Au nanoparticles (100 pages). See DOI: 10.1039/d0ra09088c |
|
This journal is © The Royal Society of Chemistry 2020 |