DOI:
10.1039/D0RA06813F
(Review Article)
RSC Adv., 2020,
10, 39171-39186
Investigation of the process stability of different anammox configurations and assessment of the simulation validity of various anammox-based kinetic models
Received
7th August 2020
, Accepted 5th October 2020
First published on 26th October 2020
Abstract
Over the last 30 years, the successful implementation of the anammox process has attracted research interest from all over the world. Various reactor configurations were investigated for the anammox process. However, the construction of the anammox process is a delicate topic in regards to the high sensitivity of the biological reaction. To better understand the effects of configurations on the anammox performance, process-kinetic models and activity kinetic models were critically overviewed, respectively. A significant difference in the denitrification capabilities was observed even with similar dominated functional species of anammox with different configurations. Although the kinetic analysis gained insight into the feasibility of both batch and continuous processes, most models were often applied to match the kinetic data in an unsuitable manner. The validity assessment illustrated that the Grau second-order model and Stover–Kincannon model were the most appropriate and shareable reactor-kinetic models for different anammox configurations. This review plays an important role in the anammox process performance assessment and augmentation of the process control.
1. Introduction
With the development of the economy of the world, eutrophication has spread fast and led to serious aquatic environmental pollution, especially in the developing countries. The excessively discharged nitrogen can cause many negative impacts, such as eutrophication. Therefore, nitrogen removal shall be processed in a sustainable way, especially for wastewater treatment with a low C/N ratio. With the lower capital investment, less operational maintenance, no additional organic carbon source, 90% reduction of sludge and the limited emission of N2O, the anaerobic ammonia oxidation (anammox) process is a new nitrogen removal technology that has gained widespread attention.1,2 However, despite the increasing interest in the anammox application, there were still many difficulties, such as the low bacterial growth rate and the sensitivity to the environmental factors.3
Anammox processes can be conducted in many different configurations. Each process has its own optimized operations and characteristics based on the design of the reactor. However, it is generally known that common reactors have certain shortcomings, and that appropriate operation conditions should be fully investigated to prevent the process from failure without optimized operation.4 Different reactors have different capabilities of nitrogen removal. Among the applied reactors, the Sequencing Batch Reactor (SBR), Up Flow Anaerobic Sludge Blanket (UASB) and Membrane Bio-Reactor (MBR) were the most popular configurations with different configuration structures that benefit the biomass enrichment in the form of the biofilm or for avoiding sludge wash out.5–7 The aim of optimizing the operational conditions is to enhance the efficiency of nitrogen removal.8 The effects of environmental factors at various operational options on the anammox microbial community were also reported.9 Moreover, the microbial residence time plays a critical role in the taxonomic composition and functional description of the microbial communities.10
A large number of previous studies focused on experimental and mathematical methods.11–13 The process-kinetic models are powerful tools for investigating the performances of different configurations during the anammox process. Appropriate kinetic mathematical models are enabled to assess, control, and mitigate the process inhibition and process optimization.
These models can be employed to achieve many key objectives involving the optimization of the chemical processes, assessment of the reaction hazards, and the design of emergency relief systems.14,15 Process-kinetic models enhance the comprehension of the constructed system, and are conducive to the formulation and validation of a hypothesis. The prediction of the system's behaviour at a variety of conditions was developed to reduce the operation risks. However, certain selection criteria and conditions must be obeyed when the proper evaluation and application of the process models were performed for understanding the process or predicting the performance. In order to identify a suitable model, a correct model type shall be selected, and the parameters of the model shall be estimated with the available data. To a great extent, the validity of the studies relies on the model, which is defined by the proper preference of a mathematical model first, and then the validity of the kinetics evaluation. Generally, an evaluation on the process performance is still characterized by the application of the simple kinetics models and methods for evaluating their reactions.
Kinetic models are proved to be a conventional way to estimate and prospect the performances and operations of different processes. It is very important to choose a suitable model relating to the kinetics evaluation of the anammox process assessment. Quantitative process-kinetics can gain insight into the underlying operational strategy with feasibility investigations in both batch and continuous processes. However, it is a pity that most frequently used models cannot fit the kinetic data well. It is necessary for us to know which model is better, and which is perfect in the simulated conditions. Moreover, the optimized operation conditions of the anammox process are also important for the lab scale and full-scale process design.
A majority of previous papers of different configurations (EGSB, UASB, SBR, MBR, and CSTR) were related to discussions of the treatment performance or engineering application. However, only a limited number of articles are available for solving the specific problems of the kinetics evaluation. So far, studies in investigating the anammox process-kinetics with different configurations are relatively limited, and the assessment of anammox performance in different reactors is rare. Thus, the performance of the anammox system in different reactors is reviewed in this paper with regards to validation of the commonly used process kinetic models.
2. Process-kinetics approaches
Different reactors used in the anammox process were summarized, and the performance comparison and kinetic simulation were also evaluated. Currently, we are mainly focusing on the application of four kinds of substrate removal models, including the first-order substrate removal model, the Grau second-order substrate removal model, the modified Stover–Kincannon model and the Monod equation, to investigate the anammox process kinetics.
2.1 First-order substrate removal model
With regard to the application of the first-order substrate removal model to the bioreactor, the change rate of the substrate concentration in the completely mixed system may be expressed as follows:12 |
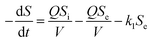 | (1) |
Because the change rate (−dS/dt) can be neglected under a pseudo-steady-state situation, the equation is derived as:
|
 | (2) |
where
Si and
Se are the total nitrogen concentrations (g L
−1) in the influent and effluent, HRT is the hydraulic retention time (day),
k1 is the constant of the first-order substrate removal rate constant (1/d),
Q is the inflow rate (L/d) and
V is the volume of the reactor (L).
2.2 Grau second-order substrate removal model
The general equation of a second-order model is described as shown below.16 By integration and linearization, the above equation can be expressed as eqn (3): |
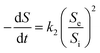 | (3) |
By integration and linearization, the above equation is expressed as eqn (4):
|
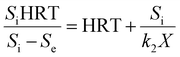 | (4) |
If Si/(k2X) is considered as a constant (a) and (Si − Se)/Si is replaced by the substrate removal efficiency (E), b is a constant for the Grau second-order model, the above equation can be represented as a simple one:
2.3 Modified Stover–Kincannon model
As for the Stover–Kincannon model,16 initially, the equation was applied to predict the development of the attached-growth biomass in a rotating biological contactor. Later, the model was further modified, and widely used to describe and predict the performances of the bioreactor.17,18 The original model is expressed, as shown below: |
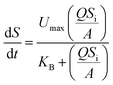 | (5) |
where dS/dt is the substrate removal rate (kg m−3 d−1), Umax is the constant of the maximum utilization rate (kg m−3 d−1), A is the surface area of the disc (L), and KB is the constant of the saturation value (kg m−3 d−1). If the volume of the reactor (V) takes the place of the surface area (A), the Stover–Kincannon model can be modified as below:18 |
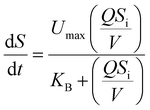 | (6) |
where the Stover–Kincannon model takes dS/dt as a function of the loading rate of substrate at a steady state:
, so it can be further expressed as shown below in eqn (7): |
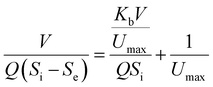 | (7) |
2.4 Monod model
The Monod model was extensively employed.19 The modified versions of the Monod equation were developed concerning the model anammox process in terms of the oxygen concentration, ammonia concentration and missing alkalinity:20 |
 | (8) |
where K is the maximum substrate utilization rate (kg m−3 d−1), and Ks is the half saturation concentration (g L−1).
2.5 Other models used for the anammox-based process
Generally, the anammox bacteria cooperated with other functional bacteria to degrade the wastewater in the applied fields, such as SHARON and anammox process based on the continuity model.21 The ASM2, ASM2d and ASM3 models were also used to simulate the anammox process, which is enriched from municipal activated sludge.22 The more complex microbial communities with AOB, NOB DHB and anammox were simulated with complex models, as reported.23
3. Anammox processes conducted in different reactors
In recent years, the interest in anammox has been increased significantly with more and more articles being published as time has passed, as showed in Fig. 1a. Since 2005, an average of 78 more papers has been published every year. In 2014, 300 papers were published. Fig. 1b shows the number of anammox processes conducted in different reactors, as previously reported. The sequencing batch reactor (SBR) was recognized as the most dominantly employed bioreactor, which was the topic in one third of published papers, followed by the upflow anaerobic sludge blanket (UASB), membrane bio-reactor (MBR), expanded granular sludge bed (EGSB), continuous stirred-tank reactor (CSTR), sequencing biofilm batch reactor (SBBR) and upflow anaerobic fixed bed (UAFB), subsequently. While the number of applied UASBs was two-thirds of that of applied SBRs, both SBRs and UASBs were the most popular reactors conducted for the anammox process. Many advantages of the reactor are summarized in Table 1. The high NLR (nitrogen loading rate) and NRR (nitrogen removal ratio), enrichment granular sludge, fast start up and high settleability can be obtained when the UASB or EGSB is applied. However, the MBR (with a long sludge retention time (SRT)) brought a fast startup of the process and a high accumulation rate for anammox enrichment, which was commonly evaluated with special purposes (Table 1). The general comparison of the anammox process conducted in different processes is shown in Table 2. The contents regarding the general process descriptions, applications, advantages and disadvantages, and design criteria are given for each technology based on previous research studies as the referenced applications. The advantages and disadvantages of each reactor constructed for anammox are listed in Table 2. Biofilm, granule and flocculent were the three main kinds of anammox conducted in different reactors, among which the biofilm and granule were the most popular ones. A previous investigation showed that UASB and SBR were the most popular bioreactors that can make granules or keep high anammox biomass. The long retention time of the active sludge is the key factor to influence the successful development of a high-rate anaerobic wastewater treatment. The long retention time of sludge can be realized due to the specific reactor configuration design and fixed biomass in the form of static biofilms, particle-supported biofilms or granule sludge. It was observed that the UASB with upflow can easily change the sludge into granules. The high loading rate of UASB with high removal efficiency was often reported.7 In contrast, the engineering applications of anammox included more than 100 full-scale partial nitridation/anammox installations worldwide by 2014. Most of them were conducted in the one-stage configuration, and the most commonly applied reactors were SBRs, followed by granular systems and MBBRs.24
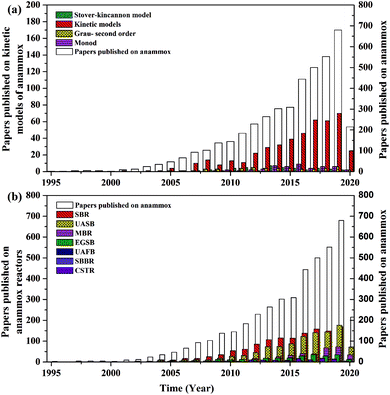 |
| Fig. 1 Published papers in each year: (a) kinetic models used in the anammox process and (b) enrichment reactors. (Web of Scopus, access data: 2020.04.06). | |
Table 1 Anammox processes conducted in different configurations (most popular reactors)a
|
UASB |
EGSB |
CSTR |
SGBR/SASBR |
(G/F) MBR |
Nov-BFR |
UAFB |
MBBR/SBR |
SGBR: static granular bed reactor; SASBR: static anaerobic sludge bed reactor; Nov-BFR: Noven biofilm reactor; MBBR: moving bed biofilm reactor. |
|
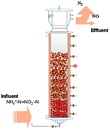 |
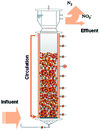 |
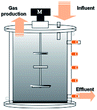 |
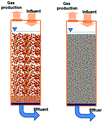 |
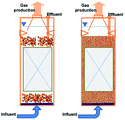 |
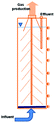 |
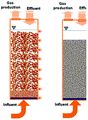 |
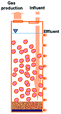 |
Advantage |
High NLR and NRR; enrichment granular sludge; high settleability |
High NLR and NRR; enrichment granular sludge; fast start up; high settleability |
Intensive mixing; ideal model for simulation |
High stability of process enrichment granular sludge; fast start up; high substrate con.; adjust process timing via PLC, convent operation |
High stability of process; fast start up/enrichment; high sludge concentration; high NRR with free cell or/granular sludge; high effluent quality; high degree of automation |
High stability; fast enrichment; biofilm culturing; high degree of automation |
High stability of process; fast start up/enrichment; high sludge concentration; high NRR with free cell or/granular sludge |
High stability; fast enrichment; biofilm culturing |
Disadvantage |
Dead zone areas and substrate inhibition; influent hierarchy; sludge washout; sludge floatation with layer distribution |
Costly to operate; sludge washout; sludge floatation consume energy |
Low SRT; consume energy |
Costly to operate; sludge washout; negative effect on downstream processes; high peak flow may disrupt performance |
Costly to operate; membrane cleaning and replacement; process sensitive to sustained peak hour flow; sophisticated operation |
Costly to operate |
Costly to operate; membrane cleaning; lighter, fluffier sludge flocs |
Costly to operate; biofilm fall off |
Table 2 Performance of anammox process conducted in different reactorsa
Reactor type |
Inoculum sludge |
Operation day (d) |
T (°C) |
pH |
Ammonium removal (%) |
NLR (kg N m−3 d−1) |
NH4+–N influent (mg L−1) |
NO2−–N influent (mg L−1) |
Sludge concentration (VS L−1) or anammox purity (%) |
Reference |
NLR: nitrogen loading rate; anammox purity: the percentage of anammox in the biomass; MBBR: moving bed biofilm reactor; EMBRs: external MBR; FBR: fixed bed reactor; PAN-An R: partial nitrification and anammox reactor; GSBF: granular fluidized bed reactor; SGSR: stirred gas solid reactor. |
UASB |
Anaerobic granules |
400 |
35 ± 1 |
7.0 |
86.5–92.3 |
6.4 |
1027 |
1409 |
— |
46 |
UASB |
Aerobic granules |
300 |
30 |
7.0 |
90 |
0.5 |
200 |
220 |
— |
26 |
UASB |
Nitrifying + anammox |
>1400 |
Normal |
6.3–7.3 |
<90 |
9.5 |
400–550 |
575–175 |
50–60 |
47 |
UASB |
Nitrifying |
220 |
30–33 |
7.5–8.0 |
97 |
0.16 |
115 |
130 |
— |
48 |
UASB |
Anammox granules |
235 |
35 |
7.8–8.0 |
99.29 (TN) |
1.03 |
458 |
575 |
91.2–92.4 |
49 |
UASB |
AN-GS A-AS |
320 |
−35 |
7.3–7.9 |
95 |
1.25 |
— |
— |
— |
50 |
UASB |
Anaerobic granular sludge anammox sludge |
214/450 |
35 ± 1 |
6.8–7 |
70–99.9 |
74.3–76.7 |
−200 |
240 |
42–57 g VS L−1 |
7 |
1 L UASB |
Synthetic medium |
70 |
35 ± 1 |
6.8–7.0 |
93.2 ± 7.1 |
|
30–70 |
30–70 |
— |
51 |
SBR |
AS biomass |
200–600 |
25 |
8.0 |
— |
— |
— |
— |
— |
52 |
SBR |
Anammox |
320 |
33 |
7.0–8.0 |
99–100 |
0.43 |
180 |
250 |
— |
53 |
SBR |
Activated sludge |
365 |
36 ± 0.3 |
7.5–8.2 |
99.9 |
1.6 |
1268 |
166.14 |
85.0 |
6 |
SBR |
Anammox |
400 |
35 ± 1 |
6.7–7.0 |
60 (TN) |
1.0 |
700 |
0 |
— |
54 |
SBR |
Anammox |
400 |
35 ± 1 |
7.5 |
78 (TN) |
0.36 |
200–250 |
0 |
<35 |
25 |
SBR |
Anammox granules |
218 |
30 ± 1 |
7.5–8.0 |
98 |
0.3 |
150 |
150 |
— |
55 |
2 L SBR |
Nitritation–anammox synthetic wastewater |
180 |
33 ± 1 |
|
85–94 |
0.18 g N per g VSS per d |
500 |
— |
1.8 g VSS per L 18–19% Ca. Jettenia (16.8%) and Nitrosomonas (20.1%) |
56 |
18 L SBR Pa + AN |
Raw optoelectronic industrial wastewater |
94 |
37 |
7.8–8.0 |
|
10–33 g m−3 d−1 |
183–200 |
— |
— |
57 |
3 L SBR |
0.6 kg N m−3 d−1 piggery waste production/no dilution |
50 d start up |
30 |
8.1 ± 1 |
91 ± 10 97 |
0.5–0.6 g Nper L per d |
— |
NO2–N removed/NH4–N removed molar ratio was 1.28 ± 14% |
274 ± 45% 2.3 g TSS per L and 79% VSS/TSS |
58 |
3 L SBR CANON |
Trace N2H4 (4 mg L−1) was added into the influent |
80 |
31 ± 1 |
— |
70 ± 7 (TN) |
0.33 ± 0.06 kg N per L per d |
150 mg N per L |
— |
Ca. Scalindua, 3.14 × 109 to 5.86 × 1010 copies per g (dry sludge) |
59 |
MBBR |
Synthetic wastewater 2 L of virgin carrier media |
86–121 |
33.4–35 |
7.5 ± 0.1 |
0.57–0.64 ± 0.17 |
0.03–0.22 |
348.36–507 |
0.48–0.77 |
Ca. Brocadia fulgida shift to Ca. Kuenenia stuttgartiensis AOB1.83–1.4 NOB |
60 |
10 L MBR |
Synthetic wastewater |
|
30 |
6.8–7.5 |
85% (TN) |
1 g N per L per d |
12.9 mM |
3.4 mM (1.21) |
Growth rate of 0.21 d−1 |
5 |
15 L/8 L MBR |
Synthetic wastewater |
250 |
37 |
7.1–7.5 |
|
|
1680 |
1680 |
0.23 day as free cells |
61 |
1.6 L MBR |
Synthetic medium |
63 |
30 ± 1 |
7.0 ± 0.2 |
82.14 |
293/1680 |
840 |
840 |
Ca. Brocadia 60% |
62 |
1.8 L GSBF |
Effluent of the A-stage of the WWTP |
20 °C/1–52 15 °C/60–127 10 °C/134–285 |
20 and 10 |
7–7.5 |
— |
50 ± 7 mg N per g VSS per d |
0.4 g N per L per d |
50 ± 7 mg N per g VSS per d |
Ca. Brocadia 0.35 g TSS/12 g TSS |
63 |
6 L SBBR |
Synthetic ammonium-rich wastewater |
30 |
35 ± 1 |
74 |
|
— |
470 |
— |
— |
64 |
SBBRs |
Synthetic medium |
90 |
35 ± 1 |
7.0–7.8 |
88 |
1.62 kg N m−3 d−1 |
|
— |
Ca. Anammoxoglobus (DQ317601.1) 40.1% |
65 |
2.6 L FBR |
Synthetic wastewater |
65 |
35 ± 0.2 |
8 ± 0.2 |
|
0.05–0.06 kg N m−3 d−1 0.25 kg N m−3 d−1 |
50 mg L−1 |
50 mg L−1 |
Ca. Kuenenia stuttgartiensis |
66 |
EMBRs |
— |
50 |
33 ± 1 |
8.0 ± 0.3 |
|
0.8 |
— |
— |
— |
67 |
5 L AnR |
Synthetic medium |
102 |
32 |
7.8 ± 0.3 |
90 |
0.30 g N L−1 d−1 |
1230 ± 61 mg L−1 |
— |
— |
68 |
4. Characteristic and stability analysis of the anammox process in different reactor setups
Different reactors used for the anammox process are summarized in Table 2. The performance such as the startup time, nitrogen-loading rate, removal efficiency, substrate concentration, and the purification of sludge is described and compared in detail. The most commonly used SBR reactor has an NLR that varied from 0.3 kg m−3 d−1 to 1.6 kg m−3 d−1 with the highest removal efficiency over 99%.6 The comparison of the most commonly used UASB and SBR processes with the maximum NRR and startup time is shown in Fig. 2. Generally, the UASB process can obtain a high NRR over 2 kg m−3 d−1. However, that of the SBR reactor was lower than 2 kg m−3 d−1 with a shorter time for startup. By comparing the anammox sludge with an organic C content lower than 35%25 and a content higher than 99%,6 it was found that the anammox sludge with higher purity can obtain a higher nitrogen removal efficiency. Similarly, the UASB reactors with different purities and operation conditions had relatively large differences in the NRRs, which might vary from 0.5 kg m−3 d−1 (ref. 26) to 76 kg m−3 d−1. To data, the highest NRR obtained was 76 kg m−3 d−1 when the UASB reactor was fed with substrate of low concentration under short HRT.7 Other reactors for the anammox process were reported to have different NRRs varying from 0.3 kg m−3 d−1 to 1.62 kg m−3 d−1 (Table 2).
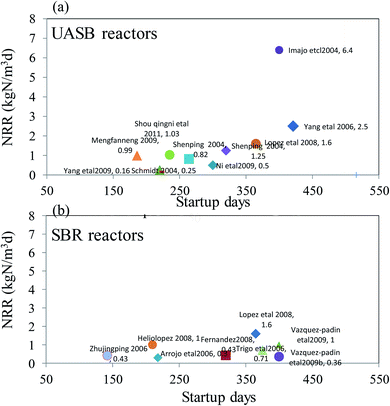 |
| Fig. 2 The start up time and maximum NRR comparison of the anammox process of the most used reactors (a) UASB and (b) SBR. | |
Over the past twenty years, many studies carried out have focused on the optimization of the anammox process parameters. The relative issues involving the substrate inhibition, temperature effect, organic matter and salinity have been extensively investigated.27 The drawback of the anammox process with quite low bacteria growth rate can be overcome by MBR, which is more effective than most other reactors for enrichment.5 A reactor configuration with a high capacity of biomass retention is essential for the anammox process. Recently, a novel process conducted in an MBR with pure free-cell suspension of highly active anammox was successful, with a higher growth rate than the specific maximum growth rate ever reported for the biomass, 0.21–0.23 d−1.5 This strategy has a number of potential capacities in the cultivation of seeding anammox in terms of practical engineering projects.2 MBR or MBBR and Nov-BFR with carriers can accumulate a certain amount of anammox to keep the process stable. Additionally, inherent with each technology are the advantages and disadvantages of the process, and/or operation and maintenance. Discharging standards are often the primary consideration in selecting a treatment technology.
Anammox processes conducted in different reactors can accumulate different kinds of anammox bacteria following the substrata and operation conditions. Because of its potentials in nitrogen removal during the biological process, the high treatment performance and stability of the anammox process have been constantly studied for engineering application. Most of the lab-scale enrichment anammox bacteria were ‘Ca. Brocadia’ and ‘Ca. Kuenenia’, which are almost mono-species in the anammox process, illustrating a competition relationship of the anammox species owing to their different substrate affinities. It should be worth noting that under specific physiological conditions, Ca. Kuenenia outgrows ‘Ca Brocadia’, resulting in overgrowth when the substrate concentration is low.28,29 Meanwhile, the ecological roles of the functional microbe and their potentials should be extensively explored in order to attain new perspectives for further design, operation, and maintenance of the process.
Generally, a large number of gas bubbles will be generated in gas tunnels and gas pockets in some high-loading bioreactors inoculated with anammox bacteria of high activity. It is speculated that the gas bubbles entrapped in the gas pockets might be a pivotal factor to cause sludge floatation, especially in the upflow reactor, such as a UASB or an EGSB. After the hollows are filled with gas bubbles, the anammox granules will unavoidably float and be washed out of the reactor, leading to a failure of the anammox process. The granule floatation can be undoubtedly responsible for instability, or even failure of the anammox reactor.30,31 The existence of this washout obstacle compels the implementation of a large number of studies in pursuit of a feasible way to overcome this obstacle.
5. Process-kinetic analysis of different anammox processes
Bibliometric analysis showed that the investigation on the reactor-kinetic models in the published paper was only one-tenth of the total. Meanwhile, the Stover–Kincannon model was the most commonly used one, which was followed by the Monod model and the Grau-second model (Fig. 1b). It was confirmed by many studies that the anammox bacteria were very sensitive to operational conditions, such as dissolved oxygen, temperature, pH and composition of organic matters. Unfavorable conditions will largely reduce the activity of the anammox bacteria, and eventually cause a failure in bioreactor performance. Therefore, it is of practical significance to effectively augment the activity of the anammox bacteria in order to achieve a high-rate process under the mainstream conditions, which might cause a large decrease in the specific activity of anammox. Most of the literature reached a consensus in that the process control for anammox is a prerequisite to practically ensure the successful performance under unstable influent conditions.32
The common bioreactors have certain shortcomings since different reactors have different optimal operating conditions. For instance, the UASB reactor often encounters unsatisfactory substrate removal efficiency, severe sludge washout, and large dead zone areas. An EGSB is costly in term of the huge consumption of energy and operation cost. In addition, a biofilm reactor has a long sludge age and sporadic sludge flotation. The main objectives of process modeling are to control and evaluate the performance of the process, as well as to optimize the system design and scale up the pilot plant investigations.33 For example, the concentrations and activities of anammox measured in different reactors could be used in conjunction with models to control the process parameters, involving the influent loading rate and hydraulic residence time in order to maximize nitrogen removal. To date, process control is widely considered in the literature as an essential factor to guarantee good performance in various bioreactors.
Modeling and parameter identification are subjects with wide ranges, offering a realm of approaches and methods. In many practical industrial projects, most knowledge is available in the form of heuristic rules achieved from rich experience in a variety of processes. Until now, the successful application of anammox in practical projects is still facing huge challenges. The kinetics involves operational and environmental factors, affecting the utilization rates of the substrates. By means of the kinetics evaluation, the optimization on the plant design and prediction of the performance of treatment plants can be conducted,14,33 and the process models relating to the anammox process can be further discussed (Table 3).11,14,34–38 Furthermore, the anammox enrichment culture can be significantly promoted because the kinetics can provide a convincing basic recipe for dealing with the operational and environmental factors affecting the performance of substrate removal.11 It is well known that many mathematical models, such as first-order substrate removal models, Grau second-order substrate removal models, Stovere–Kincannon models, and Monod models are widely adopted in the field of wastewater treatment (Table 3). For example, the first-order and second-order substrate removal models are effective for estimating the kinetic constants in anammox processes.14,39,40 In addition, the Monod model was initially applied to represent the growth of microorganisms, and widely employed to express the degradation kinetics. Moreover, the Stovere–Kincannon model is one of the most expensively developed mathematical models for determining the kinetic constants in immobilized bioreactors. Also, it was reported that the Stovere–Kincannon model has been investigated in continuously operated mesophilic and thermophilic upflow anaerobic filters for the treatment of soybean wastewater, paper pulp liquors, and simulated starch wastewater, and the determination of kinetic constants in a packed bed reactor for decolonization.11
Table 3 Comparison of the kinetics used for the anammox reactora
Reactor |
V (L) |
T (°C) |
Substrate |
Inoculum |
NLR (g L−1 d−1) |
First-order |
Grau second-order |
Modified Stover–Kincannon |
Monod |
Ref. |
HRT (d) |
k1 (1/d) |
R2 |
a (1/d) |
b |
k2 (1/d) |
R2 |
Rm |
KB |
R2 |
Ks (g L−1) |
Mmax (1/d) |
Kd (1/d) |
Y (mg VSS per mg N) |
R2 |
ILAB: internal-loop airlift bio-particle reactor; ANMR: non-woven membrane reactor; MAR: marine anammox reactor; VAF: upflow anaerobic filter; R2: correlation coefficient; k1 and k2 is the substrate removal rate constant (1/d) of each equation. |
ILAB |
24/20 |
30 ± 1 |
Synthetic wastewater |
PN-anammox granular |
420 ± 30 NH3–N mg L−1: |
1.0–0.066 |
66.9 |
0.6102 |
1.09 |
0.02 |
2.064 |
0.9954 |
22.29 |
27.25 |
0.981 |
0.002446 |
0.007408 |
0.0059 |
0.3511 |
0.71–0.94 |
34 |
Upflow filter |
|
35 |
|
Cultured activated sludge |
0.93–7.34 |
0.08–0.6 |
0.44 |
0.172 |
1.4 |
0.96 |
— |
0.985 |
12.4 |
12 |
0.979 |
— |
— |
— |
— |
— |
69 |
ANMR |
|
35 |
|
Flocculent anammox sludge |
0.107–0.746 |
0.6–3.0 |
5.31 |
0.707 |
0.11 |
1.11 |
— |
0.995 |
7.89 |
8.98 |
0.999 |
0.192 |
0.169 |
— |
— |
0.599 |
35 |
ANMR |
|
35 |
|
Flocculent anammox sludge |
115–297/153–313 |
0.6–2.9 |
5.305 |
0.706 |
0.11 |
1.11 |
|
0.9954 |
8.98 |
7.89 |
0.9986 |
0.192 |
|
0.1693 |
|
0.599 |
49 |
MAR |
0.8/0.65 |
25 |
Salty synthetic |
Cultured anammox sludge |
0.08–1.94 |
0.25–1.0 |
7.44 |
0.756 |
0.06 |
1.14 |
— |
0.991 |
6.41 |
7.37 |
0.993 |
0.107 |
0.952 |
— |
0.952 |
0.993 |
11 |
AUF |
|
35 |
|
Cultured activated sludge |
270–305 |
0.08–0.6 |
|
|
1.4 |
0.96 |
|
0.9855 |
12 |
12.4 |
0.9792 |
|
|
|
|
|
12 |
|
|
|
|
|
288–307 |
|
|
|
|
|
|
|
|
|
|
|
|
|
|
|
|
UABF |
|
35 |
|
Anammox granules |
360–700 |
1–0.25 h |
|
|
0.04 |
1.06 |
|
0.999 |
35.7 |
38.1 |
0.999 |
|
|
|
|
|
36 |
|
|
|
|
|
475–924 |
|
|
|
|
|
|
|
|
|
|
|
|
|
|
|
|
UABF |
|
35 |
|
Anammox granules |
400/528 |
1–0.25 h |
|
|
0.05 |
1.04 |
|
0.998 |
20.7 |
21.6 |
0.998 |
|
|
|
|
|
37 |
UASB |
1 |
25 |
Synthetic wastewater |
Preservation treatments- 4 + distilled water |
50–300/60–300 |
3–9.62 h |
|
|
|
|
|
|
55.5 |
56.6 |
0.99 |
|
|
|
|
|
38 |
UASB |
1 |
25 |
Synthetic wastewater |
Preservation treatments–40 + dimethyl sulfoxide |
50–300/60–300 |
3–9.65 h |
|
|
0.999 |
1.165 |
|
0.9916 |
66.7 |
67.9 |
0.992 |
|
|
|
|
|
38 |
UASB |
50 |
37 |
Synthetic wastewater |
Anammox granules |
167–278/191–411 |
0.2–3.2 |
11.64 |
0.8043 |
0.09 |
1.03 |
— |
0.9985 |
27.8 |
27.5 |
0.999 |
|
|
|
|
|
49 |
SUASB |
8.6 |
35 ± 1 |
Synthetic wastewater |
Nitrifying sludge and mature anammox granule |
5.63 |
2.5–24 h |
|
|
|
|
|
|
14.8 |
14.5 |
0.989 |
|
|
|
|
|
70 |
UASB |
1 |
35 ± 1 |
Synthetic wastewater |
0.16 × 100.24 kg Sm3 d1would |
14.6 |
|
|
|
|
|
|
|
95.29 |
84.03 |
0.9758 |
|
|
|
|
|
71 |
UASB |
1 |
35 ± 1 |
Synthetic wastewater |
Copper(II) recovery |
|
0.4–2.4 h |
|
|
|
|
|
|
151.5 |
157.9 |
0.73 |
|
|
|
|
|
72 |
UASB |
1 |
35 ± 1 |
Synthetic wastewater |
OTC recovery |
|
0.4–2.4 h |
|
|
|
|
|
|
212.8 |
228.4 |
0.746 |
|
|
|
|
|
72 |
UASB |
1.5 |
35 ± 1 |
Synthetic wastewater |
Re-startup |
|
|
|
|
|
|
|
|
49.5 |
56.8 |
0.985 |
|
|
|
|
|
41 |
UASB |
1.5 |
35 ± 1 |
Synthetic wastewater |
Re-startup |
|
|
|
|
|
|
|
|
454.5 |
528 |
0.995 |
|
|
|
|
|
41 |
UASB |
1.5 |
35 ± 1 |
Synthetic wastewater |
Re-startup |
|
|
|
|
|
|
|
|
384.6 |
447.7 |
0.99 |
|
|
|
|
|
41 |
UASB |
1.5 |
35 ± 1 |
Synthetic wastewater |
Re-startup |
|
|
|
|
|
|
|
|
476.2 |
553 |
0.996 |
|
|
|
|
|
41 |
UASB |
1.5 |
35 ± 1 |
Synthetic wastewater |
Re-startup |
|
|
|
|
|
|
|
|
144.9 |
153.7 |
0.99 |
|
|
|
|
|
41 |
UASB |
1 |
35 ± 1 |
Synthetic wastewater |
Single feed |
70–266 |
1.52–2.06 h |
|
|
|
|
|
|
15 |
17.5 |
0.934 |
|
|
|
|
|
42 |
UASB |
1 |
35 ± 1 |
Synthetic wastewater |
Multi feed |
70–267 |
1.52–2.06 h |
|
|
|
|
|
|
27.5 |
37.5 |
0.932 |
|
|
|
|
|
42 |
UASB |
|
|
|
Activated sludge |
0.15–2.8 |
0.2–3.2 |
11.6 |
0.804 |
0.09 |
1.03 |
— |
0.999 |
12.1 |
11.4 |
0.999 |
0.092 |
0.225 |
— |
— |
0.773 |
16 |
UASB recycled |
50 |
37 |
Synthetic wastewater |
Anammox granular |
0.28–1 |
<1 |
|
|
|
|
|
|
27.8 |
27.5 |
0.999 |
|
|
|
|
|
49 |
PN reactor |
5 |
28 ± 2 |
Reject water wtp |
Lab-scale fill-draw reactor |
|
|
|
|
|
|
|
|
13.9 |
30 |
0.96 |
|
|
|
|
|
73 |
UAF |
2.5 |
36 |
Reject water wtp |
Mesophilic digester of a municipal |
|
|
|
|
|
|
|
|
31.2 |
42.1 |
0.97 |
|
|
|
|
|
73 |
UAF |
2 |
30 |
Synthetic wastewater |
Wastewater treatment plant |
0.93–7.34 g L−1 |
2–14.4 |
0.4395 |
0.175 |
1.397 |
0.964 |
|
0.986 |
12.4 |
12 |
0.979 |
|
|
|
|
|
12 |
ILAR |
3.8 |
30 ± 1 |
Synthetic wastewater |
Municipal wastewater treatment plant |
(NH4)2SO4 0.39–6.96 g L−1 |
12–21 h |
|
|
|
|
|
|
5.77 |
5.39 |
0.9572 |
|
|
|
|
|
74 |
A detailed comparison of different substrate removal kinetic models for anammox reactors is listed in Table 4. Compared with the various processes conducted in the UASB, MAR, ANMR and up-filters, the result of the first-order simulation showed that the UASB had the highest K1 of 11.64 (d−1) with R2 being 0.80, and the up-filter had the lowest K1 of 0.43 (d−1) with R2 being 0.439.12,15 All of the first-order simulations proved that R2 was low, indicating the poor fitting of the data. While these data simulated by second-order simulation proved a higher R2, which was 0.99 or 0.98, respectively. Meanwhile, the modified Stover–Kincannon also gave a high R2 of 0.98 in a UAF system.12 In the UASB system, among the most commonly used modified Stover–Kincannon model, Rmax varied from 15 to 476, as reported due to the different operational conditions.41,42 The difference in the Umax values was probably due to the different adopted reactor configurations, different treated wastewater characteristics and developed microorganisms in various studies. The Stover–Kincannon model was suitable for the kinetic analysis of a complicated anaerobic process. This is partially due to the simplification of this model without diffusion of the modeling substrate, hydraulic dynamics and other parameters, which might be key factors for the reactor performance, but were difficult to measure. The methods of validity assessment between the experiment and prospect can be conducted by the linear regression equation, y = x (Fig. 3). The modified Stover–Kincannon model and the Grau second-order model are both practical because in the case that Umax is equal to kB, the Grau second-order model can be transformed to the modified Stover–Kincannon model. Thus, the total nitrogen concentration in the effluent predicted by the Grau second-order and modified Stover–Kincannon models may display high correlation with actual measured data. Conversely, the Monod model and the first-order model were proven to be not feasible in many cases. The Grau second-order model and the modified Stover–Kincannon model were both acceptable to predict the total nitrogen concentration in the effluent since their plot lines nearly coincided with the actual line (Fig. 3). The process kinetic models also illustrated that the substrate concentration and granule size should be carefully controlled as the main process parameters. Different types of kinetic models should be used to obtain the appropriate model. For the activity test simulations, most popular kinetic models used for the activity simulation are shown in Table 5. The specific anammox activity (SAA) kinetic simulation of the EGSB-anammox biomass (a–f)13 and the differences between the anammox (g), DMX-deammonification system (h), and the NF-nitrifying system (i)43 were compared and verified (Fig. 4). Other simple kinetic ASM1 models for granular biomass floatation were also investigated.44 Among the kinetic models, the basic Monod equation for the DMX process, the extended Edwards and Luong equation for the AMX process, and the Andrews equation for the NF process were used and validated to be the most appropriate equations for the process.
Table 4 Comparison of kinetic models applied to anammox reactors
Models |
Reactor |
Inoculum |
NLR (g L−1 d−1) |
HRT (d) |
Constants of models |
|
R2 |
Reference |
First-order model |
Upflow filter |
Cultured activated sludge |
0.93–7.34 |
0.08–0.6 |
0.44 |
|
0.172 |
69 |
ANMR |
Flocculent anammox sludge |
0.107–0.746 |
0.6–3.0 |
5.31 |
0.707 |
35 |
UASB |
Activated sludge |
0.15–2.8 |
0.2–3.2 |
11.6 |
0.804 |
16 |
MAR |
Cultured anammox sludge |
0.08–1.94 |
0.25–1.0 |
7.44 |
0.756 |
11 |
UASB |
Anammox granules |
167–278 |
0.2–3.2 |
11.64 |
0.8043 |
49 |
191–411 |
|
|
UASB |
Activated sludge |
0.93–7.34 |
1.5–12 |
0.458 |
0.43 |
13 |
UASB |
Anaerobic digestion sludge |
0.93–7.34 |
1.5–12 |
0.561 |
0.04 |
13 |
UASB |
|
0.93–7.34 |
1.5–12 |
0.798 |
0.18 |
13 |
|
|
|
|
a |
b |
|
|
Grau second-order model |
Upflow filter |
Cultured activated sludge |
0.93–7.34 |
0.08–0.6 |
1.4 |
0.964 |
0.985 |
69 |
ANMR |
Flocculent anammox sludge |
0.107–0.746 |
0.6–3.0 |
0.105 |
1.11 |
0.995 |
35 |
UASB |
Activated sludge |
0.15–2.8 |
0.2–3.2 |
0.0936 |
1.03 |
0.999 |
16 |
MAR |
Cultured anammox sludge |
0.08–1.94 |
0.25–1.0 |
0.0554 |
1.136 |
0.991 |
11 |
AUF |
Synthetic medium |
|
10.1–1.99 |
|
1.397 |
0.964 |
12 |
ANMR |
Flocculent anammox sludge |
115–297 |
0.6–2.9 |
0.1054 |
1.1101 |
0.9954 |
14 |
|
|
153–313 |
|
|
|
|
|
UASB |
Anammox granules |
167–278 |
0.2–3.2 |
0.09361 |
1.0287 |
0.9985 |
15 |
|
|
191–411 |
|
|
|
|
|
|
Activated sludge |
0.93–7.34 |
1.5–12 |
0.087 |
1.13 |
0.93 |
13 |
UASB |
Anaerobic digestion sludge |
0.93–7.34 |
1.5–12 |
0.051 |
1.14 |
0.98 |
13 |
|
|
0.93–7.34 |
1.5–12 |
0.091 |
1.20 |
0.97 |
13 |
|
|
|
|
Rm |
KB |
|
|
Modified stover–Kincannon model |
Upflow filter |
Cultured activated sludge |
0.93–7.34 |
0.08–0.6 |
12.4 |
12 |
0.979 |
69 |
ANMR |
Flocculent anammox sludge |
0.107–0.746 |
0.6–3.0 |
7.89 |
8.98 |
0.999 |
35 |
UASB |
Activated sludge |
0.15–2.8 |
0.2–3.2 |
11.4 |
12.1 |
0.999 |
16 |
MAR |
Cultured anammox sludge |
0.08–1.94 |
0.25–1.0 |
6.41 |
7.37 |
0.993 |
11 |
AUF |
Anammox sludge |
|
10.1–1.99 |
12 |
12.4 |
0.979 |
12 |
ANMR |
Flocculent anammox sludge |
115–297 |
0.6–2.9 |
8.98 |
7.89 |
0.9986 |
14 |
UASB |
Anammox granules |
167–278 |
0.2–3.2 |
12.1 |
11.4 |
0.999 |
15 |
|
|
191–411 |
|
|
|
|
|
|
Activated sludge |
0.93–7.34 |
1.5–12 |
0.892 |
1.019 |
0.94 |
13 |
UASB |
Anaerobic digestion sludge |
0.93–7.34 |
1.5–12 |
1 |
1.110 |
0.98 |
13 |
|
|
0.93–7.34 |
1.5–12 |
3.33 |
4.037 |
0.98 |
13 |
|
|
|
|
Rm |
Ks |
|
|
Monod model |
ANMR |
Flocculent anammox sludge |
0.107–0.746 |
0.6–3.0 |
0.169 |
0.192 |
0.599 |
35 |
EGSB |
Anammox granules |
0.76–22.87 |
0.06–0.33 |
0.632 |
0.208 |
0.986 |
75 |
UASB |
Activated sludge |
0.15–2.8 |
0.2–3.2 |
0.225 |
0.092 |
0.773 |
16 |
MAR |
Cultured anammox sludge |
0.08–1.94 |
0.25–1.0 |
0.952 |
0.107 |
0.993 |
11 |
UASB |
Anammox granules |
167–278 |
0.2–3.2 |
0.2246 |
0.0924 |
0.7725 |
15 |
191–411 |
|
|
|
|
|
UASB |
Activated sludge |
0.93–7.34 |
1.5–12 |
— |
— |
— |
13 |
Anaerobic digestion sludge |
|
|
|
|
|
|
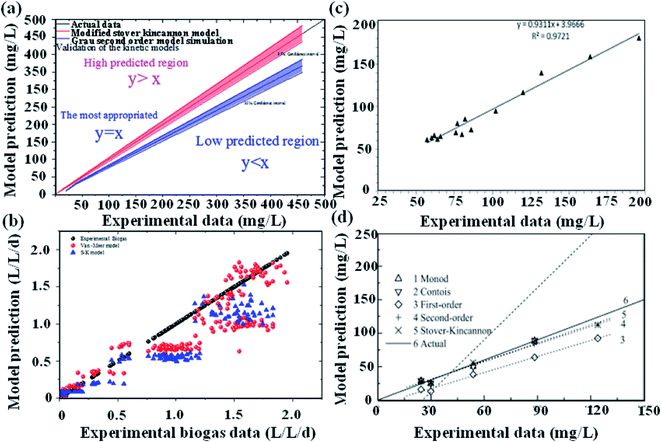 |
| Fig. 3 Reactor-kinetic validation assessment. (a), N2 gas production (b),13 substrate consumption (c),45 and the model comparison (d).15,45 | |
Table 5 Most popular kinetic models used for the activity simulationa
Simulation equations |
Reference |
Where q is the specific substrate conversion rate constant (d−1); qmax is the maximum specific substrate conversion rate constant (d−1); KS is the half saturation constant (mg N L−1); KI is the inhibition constant (mg N per L); KIH is the inhibition constant of Haldane (mg N per L); kip is the inhibition constant of Aiba; rmax is the maximum specific activity (mg L−1); Sm is the maximum removal efficiency (mg L−1 d−1). |
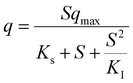 |
76 |
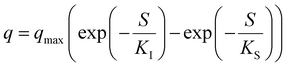 |
77 |
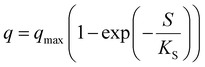 |
78 |
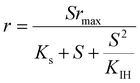 |
79 |
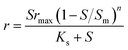 |
80 |
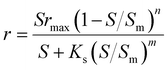 |
81 |
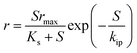 |
82 |
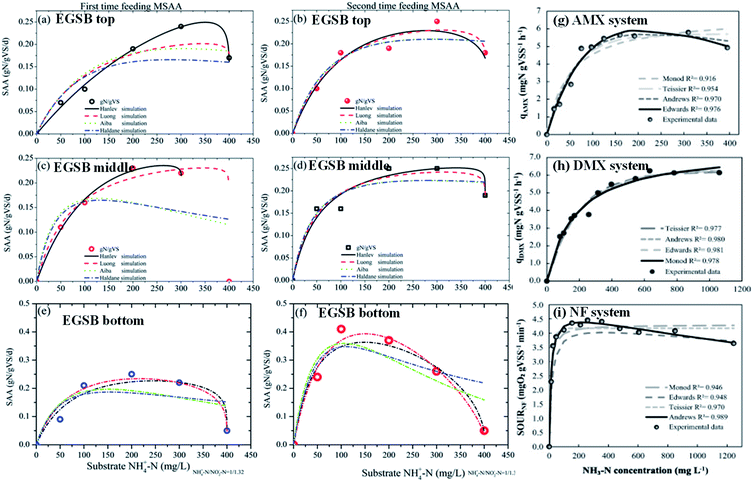 |
| Fig. 4 SAA kinetic simulation of the EGSB-anammox biomass (a–f)13 and the different systems of anammox (g), DMX-deammonification system (h), and NF-nitrifying system (i).43 | |
A simplified kinetic model lacks the capacity of expressing the detailed mechanism, but it can be used to properly describe the main characteristics of a reaction. A good model not only provides a better understanding of the complex biological and chemical fundamentals, but is also fundamental for the process design, start-up, dynamics predictions, and process control and optimization. Obviously, the simplified models with limited variables, which are confirmed suitable for practical engineering applications, were proved to be powerful tools for anammox at low growth rates. Although different kinetic models were investigated to describe the anammox process carried out in different reactors, the modified Stover–Kincannon and Grau second-order models seemed to be the most suitable for describing nitrogen removal.4,12,37 However, these models still had problems since the limitations of these kinetic models cannot describe cellular metabolism and regulation. Moreover, these models did not give any insight to the variables that could influence cell growth. In addition, these models shall be further modified when the special phase of the process was simulated to get the actual kinetics. More analysis methods and technologies for a more in-depth study shall be extensively carried out with the purpose of improving the understanding of the reaction mechanisms and microbial community dynamics.
6. Conclusions
In the review, the process-kinetics of different anammox configurations with process performance was investigated. Among the configurations, SBR, UASB and MBR were the most commonly used and favored for bacterial accumulation. The simulation models of the kinetics were proved as valuable information for process construction and rector operation. The kinetic model validation proved that the modified Stover–Kincannon model and Graus second-order model could be appropriate for different reactor anammox processes. Rather than the correlation coefficient, the validation revealed that the modified Stover–Kincannon model was more appropriate for nitrogen removal kinetics in most configurations. Based on the simulation of correlated indexes, the activity kinetic models illustrated that the modified Han–levenspiel, Luong and Andrews models were the most appropriate kinetic models for the activity simulation in the anammox process. The appreciate configurations could improve the denitrification efficiency, and contribute to the biomass enrichment in the projects of engineering application.
Conflicts of interest
There are no conflicts of interest to declare.
Acknowledgements
This work was supported by the National Natural Science Foundation of China (Grant No. 51608304) and the Scientific and Technological Projects of Henan Province (Grant No. 172102210420).
References
- D. Zhang, S. Xu, P. Antwi, L. Xiao, W. Luo, Z. Liu, J. Li, H. Su, C. Lai and F. Ayivi, Accelerated start-up, long-term performance and microbial community shifts within a novel upflow porous-plated anaerobic reactor treating nitrogen-rich wastewater via ANAMMOX process, RSC Adv., 2019, 9, 26263–26275 RSC.
- S. He, Q. Niu, H. Ma, Y. Zhang and Y.-Y. Li, The Treatment Performance and the Bacteria Preservation of Anammox: A Review, Water, Air, Soil Pollut., 2015, 226, 1–16 Search PubMed.
- M. Strous, J. G. Kuenen and M. S. M. Jetten, Key physiology of anaerobic ammonium oxidation, Appl. Environ. Microbiol., 1999, 65, 3248–3250 CrossRef CAS.
- S. Q. Ni, P. H. Lee, A. Fessehaie, B. Y. Gao and S. W. Sung, Enrichment and biofilm formation of Anammox bacteria in a non-woven membrane reactor, Bioresour. Technol., 2010, 101, 1792–1799 CrossRef CAS.
- T. Lotti, R. Kleerebezem, C. Lubello and M. Van Loosdrecht, Physiological and kinetic characterization of a suspended cell anammox culture, Water Res., 2014, 60, 1–14 CrossRef CAS.
- H. Lopez, S. Puig, R. Ganigue, M. Ruscalleda, M. D. Balaguer and J. Colprim, Start-up and enrichment of a granular anammox SBR to treat high nitrogen load wastewaters, J. Chem. Technol. Biotechnol., 2008, 83, 233–241 CrossRef CAS.
- C. Tang, P. Zheng, C. Wang, Q. Mahmood, J. Zhang, X. Chen, L. Zhang and J. Chen, Performance of high-loaded ANAMMOX UASB reactors containing granular sludge, Water Res., 2011, 45, 135–144 CrossRef CAS.
- Q. Niu, S. He, Y. Zhang, Y. Zhang, M. Yang and Y.-Y. Li, Bio-kinetics evaluation and batch modeling of the anammox mixed culture in UASB and EGSB reactors: batch performance comparison and kinetic model assessment, RSC Adv., 2016, 6, 3487–3500 RSC.
- Y. Wang, Q. Niu, X. Zhang, L. Liu, Y. Wang, Y. Chen, M. Negi, D. Figeys, Y.-Y. Li and T. Zhang, Exploring the effects of operational mode and microbial interactions on bacterial community assembly in a one-stage partial-nitridation anammox reactor using integrated multi-omics, Microbiome, 2019, 7, 122 CrossRef.
- C. Mansfeldt, S. Achermann, Y. J. Men, J. C. Walser, K. Villez, A. Joss, D. R. Johnson and K. Fenner, Microbial residence time is a controlling parameter of the taxonomic composition and functional profile of microbial communities, ISME Journal, 2019, 13, 1589–1601 CrossRef.
- X. W. Huang, Q. Y. Wei, K. Urata, Y. Tomoshige, X. H. Zhang and Y. Kawagoshi, Kinetic study on nitrogen removal performance in marine anammox bacterial culture, J. Biosci. Bioeng., 2014, 117, 285–291 CrossRef CAS.
- R. C. Jin and P. Zheng, Kinetics of nitrogen removal in high rate anammox upflow filter, J. Hazard. Mater., 2009, 170, 652–656 CrossRef CAS.
- Q. Niu, Y. Zhang, H. Ma, S. He and Y.-Y. Li, Reactor kinetics evaluation and performance investigation of a long-term operated UASB-anammox mixed culture process, Int. Biodeterior. Biodegrad., 2016, 108, 24–33 CrossRef CAS.
- S. Q. Ni, P. H. Lee and S. W. Sung, The kinetics of nitrogen removal and biogas production in an anammox non-woven membrane reactor, Bioresour. Technol., 2010, 101, 5767–5773 CrossRef CAS.
- S. Ni, S. Sung, Q. Yue and B. Gao, Substrate removal evaluation of granular anammox process in a pilot-scale upflow anaerobic sludge blanket reactor, Ecol. Eng., 2012, 38, 30–36 CrossRef.
- P. Grau, M. Dohanyos and J. Chudoba, Kinetics of multicomponent substrate removal by
activated sludge, Water Res., 1975, 9, 637–642 CrossRef.
- K. Kapdan and S. Aslan, Application of the Stover-Kincannon kinetic model to nitrogen removal by Chlorella vulgaris in a continuously operated immobilized photobioreactor system, J. Chem. Technol. Biotechnol., 2008, 83, 998–1005 CrossRef.
- H. Q. Yu, F. Wilson and J. H. Tay, Kinetic analysis of an anaerobic filter treating soybean waste-water, Water Res., 1998, 32, 3341–3352 CrossRef CAS.
- J. Monod, The Growth of Bacterial Cultures, Annu. Rev. Microbiol., 1949, 3, 371–394 CrossRef CAS.
- A. Dapena-Mora, J. Campos, A. Mosquera-Corral, M. Jetten and R. Méndez, Stability of the ANAMMOX process in a gas-lift reactor and a SBR, J. Biotechnol., 2004, 110, 159–170 CrossRef CAS.
- E. I. Volcke, M. C. van Loosdrecht and P. A. Vanrolleghem, Continuity-based model interfacing for plant-wide simulation: A general approach, Water Res., 2006, 40, 2817–2828 CrossRef CAS.
- A. Dapena-Mora, S. W. Van Hulle, J. Luis Campos, R. Méndez, P. A. Vanrolleghem and M. Jetten, Enrichment of Anammox biomass from municipal activated sludge: experimental and modelling results, J. Chem. Technol. Biotechnol., 2004, 79, 1421–1428 CrossRef CAS.
- A. Gali, J. Dosta, M. Van Loosdrecht and J. Mata-Alvarez, Two ways to achieve an anammox influent from real reject water treatment at lab-scale: Partial SBR nitrification and SHARON process, Process Biochem., 2007, 42, 715–720 CrossRef CAS.
- S. Lackner, E. M. Gilbert, S. E. Vlaeminck, A. Joss, H. Horn and M. C. M. van Loosdrecht, Full-scale partial nitridation/anammox experiences - An application survey, Water Res., 2014, 55, 292–303 CrossRef CAS.
- J. R. Vazquez-Padin, M. Figueroa, I. Fernandez, A. Mosquera-Corral, J. L. Campos and R. Mendez, Post-treatment of effluents from anaerobic digesters by the Anammox process, Water Sci. Technol., 2009, 60, 1135–1143 CrossRef CAS.
- B. J. Ni, Y. P. Chen, S. Y. Liu, F. Fang, W. M. Xie and H. Q. Yu, Modeling a Granule-Based Anaerobic Ammonium Oxidizing (ANAMMOX) Process, Biotechnol. Bioeng., 2009, 103, 490–499 CrossRef CAS.
- J.-w. CHEN, P. ZHENG, C.-j. TANG and Y. YU, Effect of Low pH on the Performance of High-loaded ANAMMOX Reactor [J], J. Chem. Eng. Chin. Univ., 2010, 2, 026 CrossRef CAS.
- J. van de Vossenberg, D. Woebken, W. J. Maalcke, H. J. Wessels, B. E. Dutilh, B. Kartal, E. M. Janssen-Megens, G. Roeselers, J. Yan and D. Speth, The metagenome of the marine anammox bacterium ‘Candidatus Scalindua profunda’illustrates the versatility of this globally important nitrogen cycle bacterium, Environ. Microbiol., 2013, 15, 1275–1289 CrossRef CAS.
- S. Ding, P. Zheng, H. Lu, J. Chen, Q. Mahmood and G. Abbas, Ecological characteristics of anaerobic ammonia oxidizing bacteria, Appl. Microbiol. Biotechnol., 2013, 97, 1841–1849 CrossRef CAS.
- H. F. Lu, P. Zheng, Q. X. Ji, H. T. Zhang, J. Y. Ji, L. Wang, S. Ding, T. T. Chen, J. Q. Zhang, C. J. Tang and J. W. Chen, The structure, density and settleability of anammox granular sludge in high-rate reactors, Bioresour. Technol., 2012, 123, 312–317 CrossRef CAS.
- J. W. Chen, Q. X. Ji, P. Zheng, T. T. Chen, C. H. Wang and Q. Mahmood, Floatation and control of granular sludge in a high-rate anammox reactor, Water Res., 2010, 44, 3321–3328 CrossRef CAS.
- J. A. Tora, J. Lafuente, C. Garcia-Belinchon, L. Bouchy, J. Carrera and J. A. Baeza, High-throughput nitridation of reject water with a novel ammonium control loop: Stable
effluent generation for anammox or heterotrophic denitritation, Chem. Eng. J., 2014, 243, 265–271 CrossRef CAS.
- M. Isik and D. T. Sponza, Substrate removal kinetics in an upflow anaerobic sludge blanket reactor decolorising simulated textile wastewater, Process Biochem., 2005, 40, 1189–1198 CrossRef CAS.
- G. Abbas, L. Wang, W. Li, M. Zhang and P. Zheng, Kinetics of nitrogen removal in pilot-scale internal-loop airlift bio-particle reactor for simultaneous partial nitrification and anaerobic ammonia oxidation, Ecol. Eng., 2015, 74, 356–363 CrossRef.
- I. K. Kapdan, Kinetic analysis of dyestuff and COD removal from synthetic wastewater in an anaerobic packed column reactor, Process Biochem., 2005, 40, 2545–2550 CrossRef CAS.
- A. A. Babaei, R. Azadi, N. Jaafarzadeh and N. Alavi, Application and kinetic evaluation of upflow anaerobic biofilm reactor for nitrogen removal from wastewater by Anammox process, Iran. J. Environ. Health Sci. Eng., 2013, 10, 2–7 CrossRef.
- N. Alavi, R. Azadi, N. Jaafarzadeh and A. A. Babaei, Kinetics of Nitrogen Removal in an Anammox Up-Flow Anaerobic Bioreactor for Treating Petrochemical Industries Wastewater (Ammonia Plant), Asian J. Chem., 2011, 23, 5220–5224 CAS.
- Y. Ji and R. Jin, Effect of different preservation conditions on the reactivation performance of anammox sludge, Sep. Purif. Technol., 2014, 133, 32–39 CrossRef CAS.
- T. T. Chen, P. Zheng, L. D. Shen, S. Ding and Q. Mahmood, Kinetic characteristics and microbial community of Anammox-EGSB reactor, J. Hazard. Mater., 2011, 190, 28–35 CrossRef CAS.
- B. K. Acharya, H. Pathak, S. Mohana, Y. Shouche, V. Singh and D. Madamwar, Kinetic modelling and microbial community assessment of anaerobic biphasic fixed film bioreactor treating distillery spent wash, Water Res., 2011, 45, 4248–4259 CrossRef CAS.
- G. F. Yang and R. C. Jin, Reactivation of effluent granular sludge from a high-rate Anammox reactor after storage, Biodegradation, 2013, 24, 13–32 CrossRef CAS.
- B.-S. Xing, T.-Y. Qin, S.-X. Chen, J. Zhang, L.-X. Guo and R.-C. Jin, Performance of the ANAMMOX process using multi-and single-fed upflow anaerobic sludge blanket reactors, Bioresour. Technol., 2013, 149, 310–317 CrossRef CAS.
- C. Marina, A. Kunz, M. Bortoli, L. A. Scussiato, A. Coldebella, M. Vanotti and H. M. Soares, Kinetic models for nitrogen inhibition in ANAMMOX and nitrification process on deammonification system at room temperature, Bioresour. Technol., 2016, 202, 33–41 CrossRef.
- J. L. Campos, A. V. del Rio, A. Pedrouso, P. Raux, E. A. Giustinianovich and A. Mosquera-Corral, Granular biomass floatation: A simple kinetic/stoichiometric explanation, Chem. Eng. J., 2017, 311, 63–71 CrossRef CAS.
- S. Tomar and S. K. Gupta, Investigating the role of co-substrate–substrate ratio and filter media on the performance of anammox hybrid reactor treating nitrogen rich wastewater, J. Biosci. Bioeng., 2016, 121, 310 CrossRef CAS.
- U. Imajo, T. Tokutomi and K. Furukawa, Granulation of Anammox microorganisms in up-flow reactors, Water Sci. Technol., 2004, 49, 155–163 CrossRef CAS.
- W. R. L. van der Star, W. R. Abma, D. Blommers, J. W. Mulder, T. Tokutomi, M. Strous, C. Picioreanu and M. C. M. Van Loosdrecht, Startup of reactors for anoxic ammonium oxidation: Experiences from the first full-scale anammox reactor in Rotterdam, Water Res., 2007, 41, 4149–4163 CrossRef CAS.
- Z. Q. Yang, S. Q. Zhou and Y. B. Sun, Start-up of simultaneous removal of ammonium and sulfate from an anaerobic ammonium oxidation (anammox) process in an anaerobic up-flow bioreactor, J. Hazard. Mater., 2009, 169, 113–118 CrossRef CAS.
- S. Ni, B. Gao, C. Wang, J. Lin and S. Sung, Fast start-up, performance and microbial community in a pilot-scale anammox reactor seeded with exotic mature granules, Bioresour. Technol., 2011, 102, 2448–2454 CrossRef CAS.
- P. SHEN, J. ZUO and Y. YANG, A Study on Start-up and Operation of Anaerobic Ammonia Oxidation Reactors Inoculated with Different Seed Sludge, China Biogas, 2004, 3, 3–7 Search PubMed.
- C.-J. Tang, P. Zheng, L.-Y. Chai and X.-B. Min, Characterization and quantification of anammox start-up in UASB reactors seeded with conventional activated sludge, Int. Biodeterior. Biodegrad., 2013, 82, 141–148 CrossRef CAS.
- K. Pynaert, B. F. Smets, S. Wyffels, D. Beheydt, S. D. Siciliano and W. Verstraete, Characterization of an autotrophic nitrogen-removing biofilm from a highly loaded lab-scale rotating biological contactor, Appl. Environ. Microbiol., 2003, 69, 3626–3635 CrossRef CAS.
- I. Fernández, J. Vázquez-Padín, A. Mosquera-Corral, J. Campos and R. Méndez, Biofilm and granular systems to improve Anammox biomass retention, Biochem. Eng. J., 2008, 42, 308–313 CrossRef.
- J. Vazquez-Padin, I. Fernadez, M. Figueroa, A. Mosquera-Corral, J. L. Campos and R. Mendez, Applications of Anammox based processes to treat anaerobic digester supernatant at room temperature, Bioresour. Technol., 2009, 100, 2988–2994 CrossRef CAS.
- B. Arrojo, A. Mosquera-Corral, J. L. Campos and R. Mendez, Effects of mechanical stress on Anammox granules in a sequencing batch reactor (SBR), J. Biotechnol., 2006, 123, 453–463 CrossRef CAS.
- Z.-r. Chu, K. Wang, X.-k. Li, M.-t. Zhu, L. Yang and J. Zhang, Microbial characterization of aggregates within a one-stage nitridation–anammox system using high-throughput amplicon sequencing, Chem. Eng. J., 2015, 262, 41–48 CrossRef CAS.
- A. Daverey, S.-H. Su, Y.-T. Huang, S.-S. Chen, S. Sung and J.-G. Lin, Partial nitrification and anammox process: a method for high strength optoelectronic industrial wastewater treatment, Water Res., 2013, 47, 2929–2937 CrossRef CAS.
- D. Scaglione, E. Ficara, V. Corbellini, G. Tornotti, A. Teli, R. Canziani and F. Malpei, Autotrophic nitrogen removal by a two-step SBR process applied to mixed agro-digestate, Bioresour. Technol., 2015, 176, 98–105 CrossRef CAS.
- P. Xiao, P. Lu, D. Zhang, X. Han and Q. Yang, Effect of trace hydrazine addition on the functional bacterial community of a sequencing batch reactor performing completely autotrophic nitrogen removal over nitrite, Bioresour. Technol., 2015, 175, 216–223 CrossRef CAS.
- H. Park, S. Sundar, Y. Ma and K. Chandran, Differentiation in the microbial ecology and activity of suspended and attached bacteria in a nitridation-anammox process, Biotechnol. Bioeng., 2015, 112, 272–279 CrossRef CAS.
- W. R. van der Star, A. I. Miclea, U. G. van Dongen, G. Muyzer, C. Picioreanu and M. van Loosdrecht, The membrane bioreactor: a novel tool to grow anammox bacteria as free cells, Biotechnol. Bioeng., 2008, 101, 286–294 CrossRef.
- A. Gonzalez-Martinez, F. Osorio, A. Rodriguez-Sanchez, M. V. Martinez-Toledo, J. Gonzalez-Lopez, T. Lotti and M. C. M. van Loosdrecht, Bacterial community structure of a lab-scale anammox membrane bioreactor, Biotechnol. Prog., 2015, 31, 186–193 CrossRef CAS.
- T. Lotti, R. Kleerebezem, C. van Erp Taalman Kip, T. L. Hendrickx, J. Kruit, M. Hoekstra and M. C. van Loosdrecht, Anammox growth on pretreated municipal wastewater, Environ. Sci. Technol., 2014, 48, 7874–7880 CrossRef CAS.
- Q. Kong, J. Zhang, M. Miao, L. Tian, N. Guo and S. Liang, Partial nitrification and nitrous oxide emission in an intermittently aerated sequencing batch biofilm reactor, Chem. Eng. J., 2013, 217, 435–441 CrossRef CAS.
- Y.-C. Yu, D.-W. Gao and Y. Tao, Anammox start-up in sequencing batch biofilm reactors using different inoculating sludge, Appl. Microbiol. Biotechnol., 2013, 97, 6057–6064 CrossRef CAS.
- T. Wang, H. Zhang, F. Yang, Y. Li and G. Zhang, Start-up and long-term operation of the Anammox process in a fixed bed reactor (FBR) filled with novel non-woven ring carriers, Chemosphere, 2013, 91, 669–675 CrossRef CAS.
- Y. Tao, D.-W. Gao, H.-Y. Wang, M. de Kreuk and N.-Q. Ren, Ecological characteristics of seeding sludge triggering a prompt start-up of anammox, Bioresour. Technol., 2013, 133, 475–481 CrossRef CAS.
- S. M. Kotay, B. L. Mansell, M. Hogsett, H. Pei and R. Goel, Anaerobic ammonia oxidation (ANAMMOX) for side-stream treatment of anaerobic digester filtrate process performance and microbiology, Biotechnol. Bioeng., 2013, 110, 1180–1192 CrossRef CAS.
- C. P. Grady Jr, L. J. Harlow and R. R. Riesing, Effects of growth rate and influent substrate concentration on effluent quality from chemostats containing bacteria in pure and mixed culture, Biotechnol. Bioeng., 1972, 14, 391–410 CrossRef CAS.
- Y.-X. Ji, B.-S. Xing, G.-F. Yang, W.-M. Ni, L.-X. Guo and R.-C. Jin, Performance and hydrodynamic features of a staged up-flow ANAMMOX sludge bed (SUASB) reactor, Chem. Eng. J., 2014, 253, 298–304 CrossRef CAS.
- R.-C. Jin, G.-F. Yang, Q.-Q. Zhang, C. Ma, J.-J. Yu and B.-S. Xing, The effect of sulfide inhibition on the ANAMMOX process, Water Res., 2013, 47, 1459–1469 CrossRef CAS.
- Q.-Q. Zhang, G.-F. Yang, H. Wang, K. Wu, R.-C. Jin and P. Zheng, Estimating the recovery of ANAMMOX performance from inhibition by copper (II) and oxytetracycline (OTC), Sep. Purif. Technol., 2013, 113, 90–103 CrossRef CAS.
- L. Zhang, J. C. Yang and K. Furukawa, Stable and high-rate nitrogen removal from reject water by partial nitrification and subsequent anammox, J. Biosci. Bioeng., 2010, 110, 441–448 CrossRef CAS.
- B.-S. Xing, Y.-X. Ji, G.-F. Yang, H. Chen, W.-M. Ni and R.-C. Jin, Start-up and stable operation of partial nitridation prior to ANAMMOX in an internal-loop airlift reactor, Sep. Purif. Technol., 2013, 120, 458–466 CrossRef CAS.
- M. S. M. Jetten, O. Sliekers, M. Kuypers, T. Dalsgaard, L. van Niftrik, I. Cirpus, K. van de Pas-Schoonen, G. Lavik, B. Thamdrup, D. Le Paslier, H. J. M. Op den Camp, S. Hulth, L. P. Nielsen, W. Abma, K. Third, P. Engstrom, J. G. Kuenen, B. B. Jorgensen, D. E. Canfield, J. S. S. Damste, N. P. Revsbech, J. Fuerst, J. Weissenbach, M. Wagner, I. Schmidt, M. Schmid and M. Strous, Anaerobic ammonium oxidation by marine and freshwater planctomycete-like bacteria, Appl. Microbiol. Biotechnol., 2003, 63, 107–114 CrossRef CAS.
- J. F. Andrews, A mathematical model for the continuous culture of microorganisms utilizing inhibitory substrates, Biotechnol. Bioeng., 1968, 10, 707–723 CrossRef CAS.
- V. H. Edwards, The influence of high substrate concentrations on microbial kinetics, Biotechnol. Bioeng., 1970, 12, 679–712 CrossRef CAS.
- G. Teissier, Growth of bacterial populations and the available substrate concentration, Rev. Sci. Instrum., 1942, 3208, 209–214 Search PubMed.
- E. F. Armstrong, Enzymes By J.B.S. Haldane, M.A. Monographs on Biochemistry, Edited by R.H.A. Plimmer, D.Sc., and Sir F. G. Hopkins, M.A., M.B., D.Sc., F.R.S. Pp. vii+235. London: Longmans, Green & Co., 1930. Price 14s, J. Chem. Technol. Biotechnol., 2010, 49, 919–920 CrossRef.
- J. H. T. Luong, Kinetics of ethanol inhibition in alcohol fermentation, Biotechnol. Bioeng., 1985, 3, 280–285 CrossRef.
- K. Han and O. Levenspiel, Extended monod kinetics for substrate, product, and cell inhibition, Biotechnol. Bioeng., 1988, 4, 430–447 CrossRef.
- S. Aiba, M. Shoda and M. Nagatani, Kinetics of product inhibition in alcohol fermentation, Reprinted from Biotechnology and Bioengineering, 1968, X(Issue 6), 845–864 (Biotechnol. Bioeng., 2000, 67, 671–690) CrossRef.
Footnote |
† These authors contributed equally to this work. |
|
This journal is © The Royal Society of Chemistry 2020 |