DOI:
10.1039/C7SM02012K
(Paper)
Soft Matter, 2018,
14, 132-139
Templated co-assembly into nanorods of polyanions and artificial virus capsid proteins
Received
10th October 2017
, Accepted 28th November 2017
First published on 29th November 2017
Abstract
Recombinant triblock polypeptides C–Sn–B, where C is a 400 amino acid long hydrophilic random coil block, Sn is a multimer of the silk-like octapeptide S = (GAGAGAGQ), and B = K12 is an oligolysine, have previously been shown to encapsulate double stranded DNA into rod-shaped, virus-like particles. In order to gain insight of the co-assembly process, and in order to be able to use these proteins for templating other types of nanorods, we here explore their co-assembly with a range of polyanionic templates: poly(acrylic acids) (PAA) of a wide range of lengths, poly(styrene sulphonate) (PSS) and the stiff anionic polysaccharide xanthan. The formation of the complexes was characterized using Dynamic Light Scattering (DLS), cryogenic Transmission Electronic Microscopy (Cryo-TEM) and Atomic Force Microscopy (AFM). Except at very high molar masses, we find that flexible anionic PAA and PSS lead to co-assembly of proteins with single polyanion chains into nanorods, with a packing factor as expected on the basis of charge stochiometry. Only for very long PAA templates (8 × 105 Da) we find evidence for heterogeneous complexes with thin and thick sections. For the very stiff xanthan chains, we find that its stiffness precludes co-assembly with the artificial viral capsid proteins into condensed and regular nanorods. Given the simple and robust formation of rod-like structures with a range of polyanionic templates, we anticipate that the artificial virus proteins will be useful for preparing high-aspect ratio nanoparticles and scaffolds of precise size and find applications in nanotechnology and materials science for which currently natural rod-like viruses are being explored.
Introduction
An improved understanding of the fundamental aspects of the self-assembly process of viral coat proteins has paved the way to establish virus-like particles as an important class of nanomaterials, with applications ranging from nanomedicine to material science.2–7 Recently, we have designed a triblock polypeptide that mimics key aspects of the highly cooperative co-assembly of viral coat proteins with nucleic acid templates.1 The polypeptide has a binding block “B” consisting of 12 lysines (B = K12) that is required for nucleic acid binding, whereas a 400 amino-acid long hydrophilic random coil sequence, denoted as “C”, provides colloidal stability. Cooperative co-assembly arises through a silk-like midblock Sn = (GAGAGAGQ)n that by itself self-assembles into stiff filaments.8 When mixed with nucleic acid template molecules, the artificial viral coat proteins (called C–S10–B and C–S14–B) were found to form rod-shaped virus-like particles, each one encapsulating a single nucleic acid molecule.1,9 As compared to natural protein capsids, the strictly modular design and simple primary sequence of our artificial viral capsid proteins are key advantages when using artificial virus particles as programmable building blocks for developing supramolecular nanomaterials.
Previously, we have extensively characterized the co-assembly of the artificial capsid proteins with single- and double stranded DNA but not with other negatively charged polyelectrolytes. For many natural viral capsid proteins, co-assembly with nucleic acids relies on non-specific electrostatic interactions, typically mediated by flexible tails rich in basic amino acids such as arginine and lysine.10–12 Not surprisingly therefore, many researchers have found that various other flexible polyanions can be used as alternative templates for the in vitro assembly of natural capsid proteins.13–15
Since binding of our artificial viral coat proteins to the nucleic acids also relies on just non-specific electrostatic interactions of the nucleic acids with the flexible oligolysine tails, we anticipate that the artificial viral coat proteins should co-assemble into regular rod-like structures with a range of polyanionic templates. Therefore, we here study the co-assembly of the artificial viral coat proteins with a range of other linear polyanionic templates: poly-acrylic acid (PAA), poly-styrene sulfonate (PSS) and xanthan; differing in contour length, charge density and stiffness. Using DLS, cryo-TEM and AFM we investigate how the template parameters influence the co-assembly of the templates with the artificial capsid proteins into rod-like structures. This will complement previous studies that have focused only in complexing nucleic acids with cationic block copolymers.16–18 In this way, we expect to gain a deeper understanding of the co-assembly process, such that we can ultimately provide guidelines for selecting polymer templates required to prepare certain specific functional nanomaterials.6,13–15,19–21
Experimental
Materials
Na-PAA with molar masses (MW) of 2.5 and 8.0 × 105 g mol−1 both with polydispersities DP = MW/MN = 1.6, were purchased from polysciences, Inc; and Na-PAA 1.25 × 106 g mol−1 was purchased from Sigma-Aldrich. PSS with MW = 2.63 × 103 g mol−1 and DP = MW/MN = 1.04 was bought from Polymer Source Inc. Xanthan gum from Xanthomonas campestris was bought from Sigma-Aldrich. Literature values of persistence lengths, contour lengths and charge densities of the polyanonic templates that were used here and in previous work are given in Table 1. The cationic artificial virus coat proteins, “C–S10–B”, “C–S14–B” and the control diblock “C–B”, were produced biosynthetically in a recombinant Pichia pastoris yeast strain and purified as described before.1,9
Table 1 Physical parameters of linear polyanionic templates to be co-assembled with artificial virus capsid proteins
|
PAA 2.5 × 105 Da |
PAA 8.0 × 105 Da |
PAA 1.25 × 106 Da |
PSS 2.6 × 105 Da |
Xanthan double helix |
dsDNA 2.5 kpb |
ssDNA 7249 nt |
PAA: polyacryic acid, PSS: poly-styrene sulfonate, dsDNA: double stranded DNA, ssDNA: single stranded DNA, kbp: kilo pair of bases, nt: nucleotides, PL: persistence length, LC: contour length, ξ: linear charge density (number of charges per nm). Ref. 22. Ref. 23 and 24. Ref. 17. Ref. 24. |
P
L (nm) |
1.2a |
1.2a |
1.2a |
2.6a |
370b |
50 |
3c |
L
C (μm) |
0.9 |
3.1 |
4.8 |
0.4 |
0.8–1.1b |
0.85 |
2.47 |
ξ (charge per nm) |
4.1 |
4.1 |
4.1 |
4.1 |
1.67d |
5.8 |
2.9 |
Sample preparation
Stock solutions of the proteins C–S10–B, C–S14–B and C–B (up to 5 mg mL−1) were prepared by dissolving freeze-dried protein powders in demi water, and heating them for 10 minutes at 65 °C. Polyanion PAA and PSS stock solutions were prepared by dissolving polymer powders in demi-water or MQ-water at concentrations of 0.2 or 0.002 mg mL−1. A xanthan stock solution of 0.1 mg mL−1 was heated up to 80 °C and cooled down slowly in order to allow the formation of the stiff helical secondary structure. For preparing the complexes, required aliquots of freshly prepared stock solutions of the proteins C–S10–B, C–S14–B and C–B and the various polyanion templates were mixed at the final desired bulk charge ratio (+/−), and diluted to the desired concentrations in a 10 mM phosphate buffer pH 7.4, 0.1 mM dithiothreitol (DTT) buffer. No DTT was used for complexes with the control diblocks C–B. The requirement for DTT comes from the fact that we need to prevent disulfide bridge formation of the N-terminal cysteine residues of C–S10–B and C–S14–B. The bulk charge ratio +/− is defined as a total number of positively charged lysine groups of the B domain over the total number of (negatively) chargeable groups of the polyanions. Compositions of all samples used for AFM imaging are given in Table 2.
Table 2 Compositions of samples for single molecule AFM imaging
Polyanion |
Concentration (μg mL−1) |
Protein |
mg mL−1 |
Charge ratio (+/−) |
ND: not determined. |
PAA (2.5 × 105 Da) |
4.0 |
C–S10–B |
1.56 |
7.5 |
PAA (8.0 × 105 Da) |
4.0 |
C–S10–B |
1.56 |
7.5 |
PAA (8.0 × 105 Da) |
4.0 |
C–S10–B |
0.2 |
1 |
PAA (2.5 × 105 Da) |
2.4 |
C–S14–B |
0.98 |
7.5 |
PAA (8.0 × 105 Da) |
2.4 |
C–S14–B |
0.98 |
7.5 |
PSS (2.6 × 105 Da) |
4.0 |
C–S10–B |
0.616 |
7.5 |
PSS (2.6 × 105 Da) |
2.4 |
C–S14–B |
0.387 |
7.5 |
Xanthan |
1–2 |
C–S10–B |
1.56 |
ND |
Xanthan |
1–2 |
C–B |
1.56 |
ND |
Dynamic light scattering
Samples consisted of C–S10–B (0.5 mg mL−1) mixed with Na-PAA 1.25 × 106 g mol−1 (0.002 mg mL−1) in phosphate buffer with DTT. The measurements were done using a low volume quartz cuvette in a Zetasizer Nano ZSP (Malvern Inc.) equipped with a He–Ne laser of 633 nm. Measurements of scattered light intensity and hydrodynamic radius (RH) were an average of at least 5 runs and were performed at a scattering angle of 173 degrees.
Cryo-TEM
Images were acquired using a JEOL 1230 TEM fitted with a LaB6 filament. For taking the images, the TEM was operated at an accelerating voltage of 100 kV. Sample solutions were Na-PAA 1.25 × 106 g mol−1 (24 μg mL−1) with C–S10–B (1.56 mg mL−1) in phosphate 10 mM buffer. They were prepared using a Vitrobot Mark IV (FEI) vitrification robot operating at 25 °C with 95–100% humidity. Approximately 10 μL of solution were deposited on a 300 mesh copper grid with lacey carbon or QUANTIFOIL®-Holey carbon support (Electron Microscopy Sciences), blotted, and plunged into a liquid ethane reservoir cooled by liquid nitrogen. The vitrified samples were transferred to a Gatan 626 cryo-holder under liquid nitrogen and acquired using a Gatan 831 bottom-mounted CCD camera.
Atomic force microscopy
Aproximately 5 μL of polyanion–protein complex solution were incubated overnight (17–23 h) at room temperature before AFM imaging. The samples were deposited onto a clean silicon substrate, incubated for 5 min, followed by washing with 1 mL of 0.2 μm filtered Milli-Q water to remove salts and non-absorbed particles. Finally, the sample was slowly dried under a N2 steam. Images were collected at 1024 lines per image and at scan rate of 0.977 Hz using a Digital Instruments NanoScope V and silicon nitride cantilevers (Veeco, NY, USA) with a nominal spring constant of 0.32 N m−1. Imaging was performed at room temperature and using the ScanAsyst™ air mode.
Image analysis
Images were processed using NanoScope Analysis 1.20 and lengths measured with ImageJ software.
Results and discussion
The triblock architecture of the artificial virus protein is illustrated in Fig. 1a. The cationic domain (12 lysines) interacts with the polyanionic template leading to the formation of co-assembled supramolecular structures. From previous studies it is known that dsDNA is condensed into structures approximately three times shorter than the contour length of the bare DNA template. Similar pDNA condensed strutures have found when block catiomers of PEG-polylysine have been used.16 Based on these findings, a hypothetical model of the co-assembled structure is shown in Fig. 1b. Similar structures are expected to form for highly flexible templates such as PAA and PSS. For stiffer templates such as xanthan, the bending energy penalty involved in packing might possibly preclude this kind of co-assembled structures.
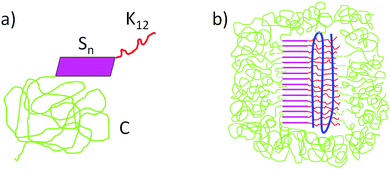 |
| Fig. 1 (a) Triblock architecture of the artificial virus coat protein C–Sn–B, C: 400 amino acid hydrophilic “coil” domain, Sn: silk-like self-assembly domain and K12: binding domain (12 lysines). (b) Hypothetical co-assembled structure formed by cationic artificial viral coat protein and anionic dsDNA.1 | |
Protein–PAA complex formation
First of all, we evaluate the formation of complexes between the virus-mimetic protein and a flexible polyanionic template. We use DLS as a method for detecting interactions and complex formation of the C–S10–B protein interacting with PAA (molar mass of 1.25 × 106 g mol−1). We follow the intensity of scattered light and hydrodynamic radius of mixtures of protein and PAA as a function of assembly time. In order to differentiate between the effects of protein self-assembly and protein–PAA co-assembly, we firstly follow assembly of the protein by itself, in the absence of a polyanion template. Next, we add PAA and follow co-assembly (Fig. 2a). The measurement sequence is started by heating the protein at 65 °C for 10 min in order to disassemble any protein aggregates and in order to make all proteins co-assembly competent.1,25Fig. 2a shows that co-assembly is associated with a much larger increase of both the intensity of scattered light, and the size than protein-only assembly. After adding the protein to the PAA there is first a notable peak in the intensity of the scattered light before it stabilizes at a lower value at long assembly times. This peak could be due to the presence of initial inter-PAA aggregates bridged by proteins, or due to intermediate structures during the condensation of the PAA template by the protein. Fig. 2b shows the time evolution of the hydrodynamic radius of the complexes. At the concentration of protein used in this experiment, the proteins alone form particles with RH of around 80 nm. Upon the addition of PAA, RH eventually increases to 110 nm.
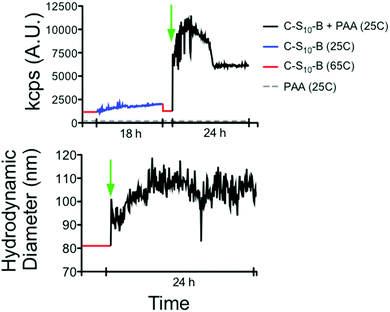 |
| Fig. 2 Kinetics of formation of complexes between C–S10–B and PAA 1.25 × 106 g mol−1 by dynamic light scattering. (Top graph) Light scattering intensity (kilo counts per second, kcps) and (Bottom graph) hydrodynamic diameter of protein (red and blue) and protein + PAA (black) at 25C. Green arrow indicates addition of PAA to protein sample. Protein was heated to 65C/10 min before addition of PAA. | |
Protein–PAA nanoparticle formation
Next we use cryogenic TEM to image the various assemblies that occur in solution (Fig. 3). Since imaging occurs after rapid vitrification of the solvent, particles observed using cryo-TEM are not affected by potential surface effects as for AFM. Fig. 3a and b shows rod-like particles that form due to co-assembly of C–S10–B and PAA. Proteins alone form much shorter rod-like aggregates (Fig. 3c) and PAA alone does not show any visible structure (Fig. 3d) in cryo-TEM. Rod-like structures observed for protein + PAA have sizes around 300 nm. They are highly polydisperse, most likely reflecting the polydispersity of the polyanion templates. There may also be polydispersity in the degree of condensation of the PAA template that leads to further polydispersity in the length of the rods.
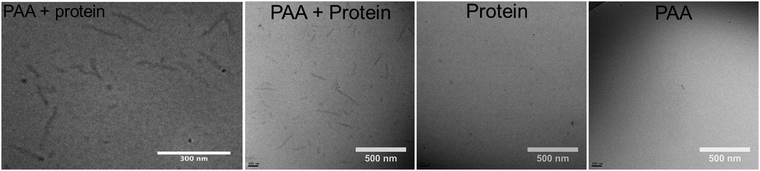 |
| Fig. 3 Cryo-TEM of nanoparticles. (Left) Protein + PAA (zoom in). (Center left) Protein + PAA (zoom out). (Center right) Control protein alone. (Right) Control PAA alone. | |
Protein self-assembly around anionic polyelectrolytes as templates
Having demonstrated that, like DNA, flexible PAA polyanion templates co-assemble in the bulk with C–S10–B to form rod-shaped assemblies, we can more confidently use AFM as a complementary imaging technique to visualize the rods and to get structural information, for example about the effect of the stiffness of the template on the co-assembly process.
Flexible anionic polyelectrolytes as templates
First we use AFM to again consider co-assembly of flexible and anionic PAA with the cationic artificial viral coat proteins C–S10–B and C–S14–B at a high charge ratio +/− = 7.5. AFM images are shown in Fig. 4. For both proteins, we find long and elongated rod-like structures and also small micelle-like structures (Fig. 4a and b). This corroborates the observed rod-like structures by cryo-TEM (Fig. 3a and b). The structures with the highest aspect ratio correspond to complexes formed between the PAA template + protein. The small structures correspond to self-assembled proteins alone, as observed in protein control (Fig. 4c), in cryo-TEM experiments (Fig. 3c), and in previous studies.1 The formation of the small aggregation by protein alone is due to the large excess of protein added to the sample. The rods are also obtained when no excess of protein is used (charge ratio +/− = 1) (Fig. 4d). It is interesting to compare the structure of the elongated rod-like complexes with the structures formed by complexation of PAA with the diblock protein C–B that lacks the silk-like self-assembly block. Previously,26 we have studied the complexes formed by the diblock C–B and long PAA (MW = 8 × 105 Da). For that case, AFM imaging showed distinct pearl-necklace structures of connected spherical micelles. This structure was hypothesized to arise from the balance between the long “C” block that favors expanded structures, and the cationic binding block B that favors condensation of the PAA into a complex coacervate.22,24–26 In contrast, complexes of PAA with the artificial viral coat proteins C–S10–B and C–S14–B have a regular rod-like shape, indicating that their structure is completely dominated by the tendency of the “Sn” blocks to stack into fibrils.8
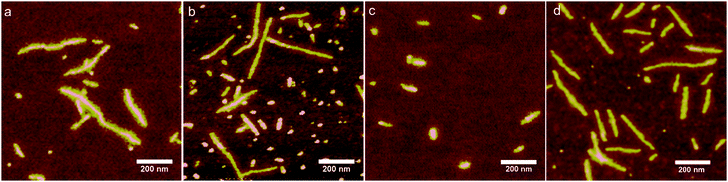 |
| Fig. 4 AFM images of complexes of polyacrylic acid (PAA, MW = 8.0 × 105 Da) with artificial capsid proteins. (a) PAA + protein C–S10–B, charge ratio +/− = 7.5. (b) PAA + protein C–S14–B, charge ratio +/− = 7.5. (c) Protein C–S10–B without PAA. (d) PAA + protein C–S10–B, charge ratio +/− = 1. For all images the simple was incubated for 24 h. | |
Next, PAA templates of different lengths were co-assembled with C–S10–B and C–S14–B proteins. High magnification AFM images of the resulting structures for PAA with molar masses of 2.5 × 105 g mol−1 and 8.0 × 105 g mol−1 with both C–S10–B (Fig. 5a and b) and C–S14–B (Fig. 5c and d), along with typical height profiles across selected complexes. In the images in Fig. 5 we observed that the structures formed with C–S10–B appear to be somewhat rougher and thicker than those formed with C–S14–B. Average heights of the PAA-complexes were determined from AFM images and are given in Table 3. For both proteins the height of the complexes is well defined. It is between 2.7–2.8 nm for C–S10–B + PAA rods and 2.6–3.0 nm for C–S14–B + PAA rods. Note that the height of the micelles in the C–S10–B protein-only control sample (Fig. 4c) was 2.6 nm. All these values are close to the expected height of approximately 2.8 nm of self-assembled Sn domains (with beta-roll or beta sheet secondary structure) as determined using small-angle X-ray scattering experiments and computer simulations.8,27–30 They are also very similar to heights determined previously for complexes with double stranded DNA (dsDNA), and these are also given in Table 3. The fact that protein–PAA complexes and protein structures have the same height strongly suggests that the structure of the complexes is the same, and consists of a stack of beta-roll or beta-sheet Sn blocks, with the PAA being wrapped by the B blocks located right next to the silk blocks.
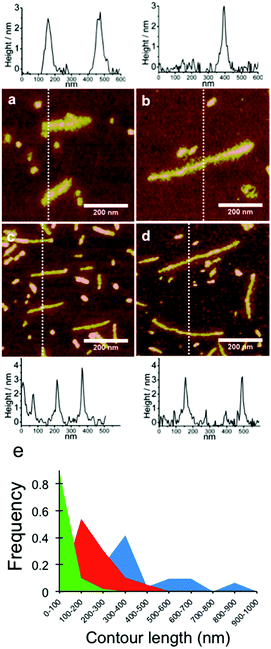 |
| Fig. 5 AFM images of complexes of polyacrylic acid with artificial capsid proteins C–S10–B and C–S14–B: effect of PAA template length. Charge ratio is +/− = 7.5. (a) C–S10–B + PAA 2.5 × 105 Da. (b) C–S10–B + PAA 8.0 × 105 Da. (c) C–S14–B + PAA 2.5 × 105 Da. (d) C–S14–B + PAA 8.0 × 105 Da. Below the images are height profiles along the sections indicated with the dashed lines. (e) Length distributions of self-assembled structures with C–S10–B. Green: protein only (N = 100), red: PAA 2.5 × 105 g mol−1 (N = 39), blue: PAA 8.0 × 105 g mol−1 (N = 31). | |
Table 3 Average heights (nm) of self-assembled structures as determined from AFM
|
PAA 2.5 × 105 g mol−1 |
PAA 8.0 × 105 g mol−1 |
PSS 2.63 × 105 g mol−1 |
Xanthan |
Protein only |
dsDNA |
C–S10–B |
2.7 ± 0.7 (N = 30) |
2.8 ± 0.7 (N = 30) |
2.5 ± 0.6 (N = 21) |
2.9 ± 0.8 (N = 42) |
2.6 ± 0.7 (N = 21) |
≈3.0 |
C–S14–B |
3.0 ± 0.7 (N = 20) |
2.6 ± 0.8 (N = 30) |
— |
— |
2.7 ± 0.7 (N = 21) |
≈3.0 |
C–B |
— |
— |
— |
1.1 ± 0.4 (N = 32) |
— |
≈1.0 |
As a consequence of the observed polydispersity of the PAA template lengths, the lengths of the complexes are also rather polydisperse. Cryo-TEM images also have shown this. Nevertheless, it is clear from Fig. 5 that the average lengths of the complexes increase with the average length of the template PAA. Despite the large polydispersity of the PAA samples, we have attempted to measure the average lengths and length distributions for the complexes formed by C4-S10–B with PAA from AFM images. Average lengths for the complexes with C–S10–B are 212 ± 87 nm and 394 ± 193 nm for PAA molar masses of, respectively, 2.5 × 105 g mol−1 and 8 × 105 g mol−1. At the same protein concentration, the average length for the protein aggregates without PAA template was 76 ± 35 nm. The PAA complexes coexist with protein-only micelles due to the charge excess. When determining the average lengths of the complexes with PAA, we therefore ignored objects with sizes <100 nm. For both PAA cases, the final average length of the rod-like PAA–protein complexes is shorter than the average expected contour length of a PAA polymer chain (Table 1), indicating that PAA chains are folded inside the rod-like complexes, as we have also found for dsDNA and ssDNA.1
The full length distributions for the complexes with the two PAAs, as derived from multiple AFM images, are plotted in Fig. 5e, along with the length distribution of the protein-only control sample. The length distribution of the complexes with the longer 8.0 × 105 g mol−1 PAA sample shows multiple peaks: peak 1, average length: 290 nm (71%), peak 2: 585 nm (22.6%) and peak 3: 870 nm (6.4%). In the case of the shorter PAA 2.5 × 105 g mol−1 there is one main peak: 201 nm (95%). The presence of more than one peak may indicate that for the longer PAA, with molar mass 8.0 × 105 g mol−1, the complexes do not have a homogeneous population. Next, we calculate packing factors for the complexes with PAA. These are defined as: contour length of template/contour length of complex. For DNA, we found that packing factors were inversely proportional to charge density: for dsDNA, the observed packing factor was equal to about 3, whereas for ssDNA, that has a (roughly) twice lower charge density than DNA, the packing factor was around 6. For the PAA of 8.0 × 105 g mol−1, assuming each complex is encapsulating a single PAA with contour length 1.9 μm, we find packing factors of 10.6, 5.3 and 3.5 for peak 1, 2 and 3; respectively. For PAA of 2.5 × 105 g mol−1, assuming each complex is encapsulating a single PAA chain with contour length 0.6 μm, we find a packing factor of 4.8 for the single dominant peak. Analysis of the AFM images indeed supports the notion that the population of complexes is heterogeneous for the PAA of 8.0 × 105 g mol−1 complexes, whereas it is more homogeneous for the PAA of 2.5 × 105 g mol−1 complexes: for a fraction of the complexes with PAA of 8.0 × 105 g mol−1, the thickness and width along the contour of the complex are not constant. Some examples are shown in Fig. 6.
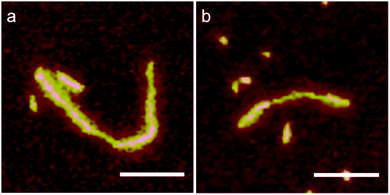 |
| Fig. 6 Non-homogeneous complexes for long PAA, 8.0 × 105 g mol−1 with C–S10–B at charge ratio +/− = 7.5 after 24 h of incubation. | |
Multiplying the packing factor of a particular PAA–protein complex with the linear charge density of the PAA (Table 1) we obtain the PAA charge density along the contour of the complexes. For the shorter PAA of 2.5 × 105 g mol−1, this translates to about 12 negative charges per 0.6 nm along the contour of the complex. For the complexes with DNA we have shown that the packing factors can be explained by simply assuming that (1) the core of the complexes consists of a single stack of silk blocks, and (2) that all lysine charges of the binding blocks B are neutralized by DNA phosphate charges. The same appears to hold for the shorter PAA, since the value of 12 charges per 0.6 nm, is very close to values for the height of a single silk-like self-assembly block in the stack: inter-protein distances found for related silk-like domains are 0.48 nm for a fully folded beta-roll and 0.7 to 0.8 nm for a partially unfolded meta-stable state.8,28 With this in mind, we argue that for the longer PAA of 8 × 105 g mol−1, it is the middle peak in the distribution (packing factor 5.3) that corresponds to fully charge neutralized complexes with a single silk-stack in their core. The large peak with a packing factor of 10.6 must consist of complexes that on average could have two silk stacks in their cores. Possibly, for these complexes the core has backfolded, indicating that for the case of the PAA complexes, there may be a tendency for sideways association of complexes. For now, the origin of the much smaller third peak with the somewhat lower packing factor is not so clear.
Next, we compare the results found for PAA with those for some other anionic polyelectrolytes. For poly-styrene sulfonate (PSS) with molar mass 2.6 × 105 g mol−1 and contour length of about 0.4 μm, we find very similar rod-like complexes (Fig. 7), with an average length of around 150 nm, which would imply a packing factor of around 3, roughly consistent with the expected packing factor based on the charge density of PSS. For this case, the relatively short length of the template makes it impossible to reliably distinguish between protein-only rods and protein–PSS complexes, so that we cannot obtain a more precise determination.
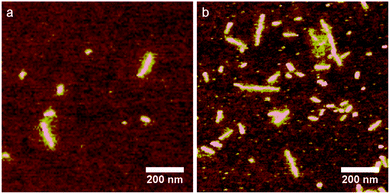 |
| Fig. 7 Complexes of poly-styrene sulfonate (PSS) of 2.6 × 105 g mol−1 with C–S10–B and C–S14–B. | |
Semiflexible anionic polyelectrolyte as template
The fact that the polyanionic templates are folded inside the rod-like particles implies that co-assembly involves a bending energy penalty. While for double stranded DNA with a persistence length PL = 50 nm it is clear that the bending energy penalty does not preclude co-assembly into the regular rod-shaped virus-like particles, this needs not be the case for polyanionic templates with still higher persistence lengths. Thus, we finally consider the case of xanthan, a semiflexible anionic polyelectrolyte with a persistence length that is significantly larger than PAA, PSS and DNA. In a recent study, a value for the persistence length of xanthan was found to be PL = 370 nm.31 A representative AFM image of xanthan co-assembled with C–S10–B is shown in Fig. 8a. We find that the complexes of xanthan with C–S10–B are typically branched, and distinctly curved rather than rod-shaped. Their average height is similar to that of the complexes with DNA, PAA and PSS: 2.9 ± 0.8 nm (Table 3). The average length of the complexes (sum of the lengths of all branches) was determined to be LC = 1.0 ± 0.4 μm (N = 37). We have previously demonstrated that for semiflexible DNA,9 but not for flexible PAA,26 complexation with C–B diblocks (rather than with the C–S10–B triblocks) lead to the formation of a bottle-brush structure with an enhanced persistence length but packing factor 1. For determining the contour lengths of the xanthan molecules we therefore coat them with the C–B diblocks, such that we can use identical substrates and imaging conditions for obtaining AFM images and contour lengths. A representative AFM image of a complex of xanthan with C–B is shown in Fig. 8b. We find an average height of 1.1 ± 0.4 nm (N = 32) and an average contour length of LC = 1,4 ± 0.5 μm (N = 24). Within the experimental uncertainty this is equal to a previous estimate of 0.8–1.2 μm (in 0.01 M of KCl) for a xanthan sample from the same provider, as determined using AFM.31 All in all, the experimental findings point to very different types of complexes for stiff xanthan when compared to complexes with more flexible polyanionic templates (DNA, PAA, and PSS). This indicates that the xanthan is probably too stiff to be folded into compact regular rod-shaped particles by the co-assembly with the C–S10–B artificial capsid proteins.
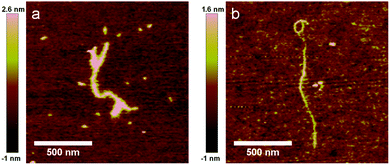 |
| Fig. 8 Complexation of semiflexible polyanion xanthan with proteins. (a) Xanthan + C–S10–B and (b) xanthan + C–S14–B. | |
Conclusions
We have shown that the artificial virus capsid proteins C–S10–B and C–S14–B form regular, rod-like nanoparticles when flexible synthetic polyanions such as PAA and PSS and natural polyanions as dsDNA are used as template for co-assembly. The rod-like nanoparticles most likely have a core formed by a single stack of silk blocks. Charge neutralization of the pendant binding blocks, B = 12 lysines, determines the stoichiometry of the complexes and requires that the polyanionic templates be folded up multiple times in length along the silk-like core. If the stiffness of the polyanionic template is too large, as is the case for xanthan, the large bending energy associated with folding the template along the silk-stack (as required for charge neutralizing the pendant B blocks) precludes a proper co-assembly into regular rod-shaped complexes. Given the easy and robust formation of regular rod-like structures with a range of polyanionic templates, we anticipate that the artificial virus coat proteins will be very useful for preparing precisely controlled high-aspect ratio nanoparticles and scaffolds for many of the same applications in nanotechnology and materials science for which currently natural rod-like viruses are being explored.4,32,33
Conflicts of interest
There are no conflicts to declare.
Acknowledgements
The authors thank F. A. de Wolf and M. W. T. Werten for their help to produce the polypeptides. A. H.-G. thanks the financial support from the Dutch Polymer Institute (DPI), project #698 SynProt, the Consejo Nacional de Ciencia y Tecnología (CONACyT), México, and the DGAPA-UNAM for the PAPIIT project AI201917. M. C. S. thanks the financial support from the European Research Council (ERC advanced grant no. 267254 BioMate).
References
- A. Hernandez-Garcia,
et al., Design and self-assembly of simple coat proteins for artificial viruses, Nat. Nanotechnol., 2014, 9, 698–702 CrossRef CAS PubMed.
- M. Comellas-Aragones,
et al., A virus-based single-enzyme nanoreactor, Nat. Nanotechnol., 2007, 2, 635–639 CrossRef CAS PubMed.
- T. Douglas and M. Young, Host-guest encapsulation of materials by assembled virus protein cages, Nature, 1998, 393, 152–155 CrossRef CAS.
- Z. Liu, J. Qiao, Z. Niu and Q. Wang, Natural supramolecular building blocks: from virus coat proteins to viral nanoparticles, Chem. Soc. Rev., 2012, 41, 6178–6194 RSC.
- L. Naldini,
et al., In vivo gene delivery and stable transduction of nondividing cells by a lentiviral vector, Science, 1996, 272, 263–267 CAS.
- P. J. Yoo,
et al., Spontaneous assembly of viruses on multilayered polymer surfaces, Nat. Mater., 2006, 5, 234–240 CrossRef CAS PubMed.
- S. G. Zhang, Fabrication of novel biomaterials through molecular self-assembly, Nat. Biotechnol., 2003, 21, 1171–1178 CrossRef CAS PubMed.
- A. A. Martens,
et al., Triblock Protein Copolymers Forming Supramolecular Nanotapes and pH-Responsive Gels, Macromolecules, 2009, 42, 1002–1009 CrossRef CAS.
- A. Hernandez-Garcia, M. W. T. Werten, M. C. Stuart, F. A. de Wolf and R. de Vries, Coating of Single DNA Molecules by Genetically Engineered Protein Diblock Copolymers, Small, 2012, 8, 3491–3501 CrossRef CAS PubMed.
- P. van der Schoot and R. Bruinsma, Electrostatics and the assembly of an RNA virus, Phys. Rev. E: Stat., Nonlinear, Soft Matter Phys., 2005, 71, 12 CrossRef PubMed.
- D. L. Caspar and K. Namba, Switching in the self-assembly of tobacco mosaic virus, Adv. Biophys., 1990, 26, 157–185 CrossRef CAS PubMed.
- P. Ni,
et al., An Examination of the Electrostatic Interactions between the N-Terminal Tail of the Brome Mosaic Virus Coat Protein and Encapsidated RNAs, J. Mol. Biol., 2012, 419, 284–300 CrossRef CAS PubMed.
- M. A. Kostiainen, P. Hiekkataipale, J. A. de la Torre and R. J. M. Nolte, Cornelissen JJLM. Electrostatic self-assembly of virus-polymer complexes, J. Mater. Chem., 2011, 21, 2112–2117 RSC.
- R. D. Cadena-Nava,
et al., Exploiting Fluorescent Polymers To Probe the Self-Assembly of Virus-like Particles, J. Phys. Chem. B, 2011, 115, 2386–2391 CrossRef CAS PubMed.
- B. C. Ng, S. T. Chan, J. Lin and S. H. Tolbert, Using Polymer Conformation to Control Architecture in Semiconducting Polymer/Viral Capsid Assemblies, ACS Nano, 2011, 5, 7730–7738 CrossRef CAS PubMed.
- K. Osada,
et al., Quantized Folding of Plasmid DNA Condensed with Block Catiomer into Characteristic Rod Structures Promoting Transgene Efficacy, J. Am. Chem. Soc., 2010, 132, 12343–12348 CrossRef CAS PubMed.
- T. A. Tockary,
et al., Rod-to-Globule Transition of pDNA/PEG–Poly(L-Lysine) Polyplex Micelles Induced by a Collapsed Balance Between DNA Rigidity and PEG Crowdedness, Small, 2016, 12, 1193–1200 CrossRef CAS PubMed.
- K. Hayashi,
et al., Influence of RNA Strand Rigidity on Polyion Complex Formation with Block Catiomers, Macromol. Rapid Commun., 2016, 37, 486–493 CrossRef CAS PubMed.
- A. Kivenson and M. F. Hagan, Mechanisms of Capsid Assembly around a Polymer, Biophys. J., 2010, 99, 619–628 CrossRef CAS PubMed.
- I. J. Minten, Y. J. Ma, M. A. Hempenius, G. J. Vancso, R. J. M. Nolte and J. Cornelissen, CCMV capsid formation induced by a functional negatively charged polymer, Org. Biomol. Chem., 2009, 7, 4685–4688 CAS.
- R. C. Evans, Harnessing self-assembly strategies for the rational design of conjugated polymer based materials, J. Mater. Chem. C, 2013, 1, 4190–4200 RSC.
- M. Tricot, Comparison of experimental and theoretical persistence length of some polyelectrolytes at various ionic strengths, Macromolecules, 1984, 17, 1698–1704 CrossRef CAS.
- K. Kawakami and T. Norisuye, Second virial coefficient for charged rods: sodium xanthan in aqueous sodium chloride, Macromolecules, 1991, 24, 4898–4903 CrossRef CAS.
- L. Zhang, T. Takematsu and T. Norisuye, Potentiometric titration of xanthan, Macromolecules, 1987, 20, 2882–2887 CrossRef CAS.
- M. M. J. Smulders, M. M. L. Nieuwenhuizen, T. F. A. de Greef and P. van der Schoot, Schenning APHJ, Meijer EW. How to Distinguish Isodesmic from Cooperative Supramolecular Polymerisation, Chem. – Eur. J., 2010, 16, 362–367 CrossRef CAS PubMed.
- M. D. Golinska, F. de Wolf, M. A. C. Stuart, A. Hernandez-Garcia and R. de Vries, Pearl-necklace complexes of flexible polyanions with neutral-cationic diblock copolymers, Soft Matter, 2013, 9, 6406–6411 RSC.
- M. Schor and P. G. Bolhuis, The self-assembly mechanism of fibril-forming silk-based block copolymers, Phys. Chem. Chem. Phys., 2011, 13, 10457–10467 RSC.
- M. Schor, A. A. Martens, F. A. Dewolf, M. A. C. Stuart and P. G. Bolhuis, Prediction of solvent dependent beta-roll formation of a self-assembling silk-like protein domain, Soft Matter, 2009, 5, 2658–2665 RSC.
- J. M. Smeenk, M. B. J. Otten, J. Thies, D. A. Tirrell, H. G. Stunnenberg and J. C. M. van Hest, Controlled Assembly of Macromolecular β-Sheet Fibrils, Angew. Chem., Int. Ed., 2005, 44, 1968–1971 CrossRef CAS PubMed.
- B. Zhao, M. A. Cohen Stuart and C. K. Hall, Dock ’n Roll: Folding of a Silk-Inspired Polypeptide into an Amyloid-like Beta Solenoid, Soft Matter, 2016, 12, 3721–3729 RSC.
- T. A. Camesano and K. J. Wilkinson, Single Molecule Study of Xanthan Conformation Using Atomic Force Microscopy, Biomacromolecules, 2001, 2, 1184–1191 CrossRef CAS PubMed.
- S. E. Aniagyei, C. DuFort, C. C. Kao and B. Dragnea, Self-assembly approaches to nanomaterial encapsulation in viral protein cages, J. Mater. Chem., 2008, 18, 3763–3774 RSC.
- C. M. Soto and B. R. Ratna, Virus hybrids as nanomaterials for biotechnology, Curr. Opin. Biotechnol., 2010, 21, 426–438 CrossRef CAS PubMed.
|
This journal is © The Royal Society of Chemistry 2018 |
Click here to see how this site uses Cookies. View our privacy policy here.