New insights into the enzymatic hydrolysis of lignocellulosic polymers by using fluorescent tagged carbohydrate-binding modules†
Received
4th September 2017
, Accepted 15th December 2017
First published on 15th December 2017
Abstract
The development of a bio-based economy requires the utilization of lignocellulosic biomass in a cost-effective way. The economic viability of lignocellulosic biomass-based industries is hindered by our imperfect understanding of biomass structure and suboptimal industrial processes. To achieve such goals requires direct and rapid monitoring of lignocellulosic polymers as they are physically, chemically, and/or enzymatically treated. In this study, the recently reported fluorescent protein tagged carbohydrate binding modules method (FTCM) was used to specifically track mechanical, chemical and enzymatic-induced variations of hemicelluloses at the surface of different wood fibers. Our results showed that susceptibility to hydrolysis in kraft pulp was higher for xylan, while mannan was more vulnerable in mechanical pulps. Furthermore, FTCM rapidly and efficiently detected enzymatic inactivation and the apparent complementarity (additive and/or synergistic effect) between cellulase and other enzymes (xylanase and mannanase), significantly bolstering cellulose and hemicelluloses hydrolysis. Subsequent addition of xylanase and mannanase enzymes directly proved that xylan was acting as a physical shield which was covering mannan in bleached kraft pulp. This study suggests that mannan was closely associated with cellulose or was deeply embedded in the cell wall organization of such fibers. FTCM provided direct support for previous models on fiber structure that were based on time-consuming and complicated approaches (i.e. chromatography, spectroscopy and microscopy). FTCM allowed for the monitoring of layers of polymers as they were exposed after treatments, providing key information regarding hydrolysis optimization and the specific susceptibility of xylan and mannan to biomass treatments. We believe that by applying this simple and rapid method on site, biomass industries could substantially improve cost-effectiveness of production of biofuels and other lignocellulosic biomass-based products.
Introduction
Lignocellulosic biomass is the most abundant, renewable and sustainable feedstock allowing our ever-increasing energy demand to be satiated while fossil fuels progressively disappear.1–5 In addition, the greenhouse gas mitigation and near carbon neutrality afforded by the conversion of biomass to bio energy (biofuel) and chemicals are important advantages over conventional fossil fuels.2,6–8 The development of a bio-based economy, however promising, is faced with challenges related to the cost-effective utilization of the lignocellulosic biomass. Improving biomass processes would increase cost effectiveness and competitiveness for large scale applications.3,9,10 The main obstacle for biofuel and chemicals production is associated with the inherent recalcitrant nature of lignocellulosic biomass.3,11–14 Due to the structural complexity of lignocellulosic biomass, the bioconversion of biomass to biofuel is a multiple stage process.8,15 The enzymatic hydrolysis of the lignocellulosic component to fermentable sugars is a crucial step in this bioconversion. It is considered as one of the major rate limiting and costly step.16–21
The complex recalcitrance nature of biomass is partly attributed to hemicelluloses. They constitute about 20–30% of the total biomass, and are the second most common polysaccharides22–24 in nature, after cellulose. Unlike cellulose, hemicelluloses are heterogeneous polymers of pentoses (xylose, arabinose), hexoses (mannose, glucose, galactose) and/or uronic acids (glucuronic acid, galacturonic acid).22,25 Hemicelluloses in hardwood (from angiosperms) mostly consist of xylan, whereas softwood (from gymnosperms) typically contains glucomannans.26 The hemicelluloses have frequently been recognized to act as a physical barrier, that cover the outer surface of cellulose fibers and interfibrillar space, limiting the accessibility of cellulase enzymes to cellulose.27–35 The hemicellulose-degrading activities in most commercially available cellulase enzymes are too low to achieve sufficient hydrolysis of the hemicelluloses.36,37 Therefore, addition of enzyme extracts or additives with higher level of hemicellulases are important for eliminating the significant hindering effect of residual hemicelluloses (mostly xylan and mannan) on the enzymatic hydrolysis of cellulose.27,29,35,38–43 In order to increase the efficiency of wood fiber utilization, it is important to utilize all wood fiber constituents (including hemicelluloses and lignin) in an economically feasible way (providing other valuable wood-derived materials beside biofuel). However, this requires a better understanding of the ultrastructure of the cell wall and its organization, which are not yet fully understood.20 Different chromatography, spectroscopy and microscopy techniques have been used to study lignin–carbohydrate complexes,38 polymers interactions44 and plant cell wall deconstruction.20 For instance, an FT-IR study of softwood fiber (kraft pulp) dedicated to investigate the interactions between wood polymers revealed that glucomannan was closely associated to cellulose while there existed no mechanical interactions between xylan and cellulose.44 Current models suggest that hemicelluloses play a major role in biomass recalcitrance and are closely associated with both lignin and cellulose, forming lignin–hemicellulose complexes and cellulose–hemicellulose complexes.38 These techniques revealed important information on hemicellulose's location and their influence on the recalcitrance nature of fibers, and enhanced our understanding of the structural arrangement of fibers. They are however invasive, time-consuming, complex and are dependent on specialized equipment and expertise.
The inherent recalcitrance nature of plants directly or indirectly impacts enzyme accessibility,12,35,38,43 inactivation,45 inhibition40,41,46–55 and, as a consequence, cost of use.9,10,27 The recent improvement in enzymes stabilization, activity, cost-effectiveness41,43,56–61 and development of new promising pretreatment conditions62–64 improved production yields. However, the high dose requirements of these enzymes often jeopardize commercial viability.27,42,45,65–68 Therefore, investigating biomass recalcitrance of typical wood biomass substrates, and correlating process parameters such as enzyme dosage, temperature, incubation time, inactivation and inhibition, with polymers hydrolysis efficiency is important. We anticipate that such advances would support engineers for increasing yields and mitigate production costs associated with lignocellulosic biomass based industries.
One of the major difficulties in studying biomass recalcitrance and process parameters is the lack of rapid, high throughput and reliable tools21 for monitoring and/or tracking hemicelluloses at the surface of wood fibers. Over the past decade, several techniques have been developed for direct and rapid detection of biomass polymers.69–71 Among these techniques, carbohydrate-binding modules (CBMs) are more powerful and advantageous as detection probes compared to others (such as chemical dyes, monoclonal antibodies etc.) due to their high specificity towards lignocellulosic polymers.70–72 CBMs are the non-catalytic polysaccharide-recognizing modules of glycoside hydrolases enzymes.70–76 Until now, CBMs have been implemented for various fundamental research on plant cell chemistry and their structure,77,78 cellulose accessibility and surface morphology,74,79,80 as well as for several industrial applications.72,81–83
Considering the importance of lignocellulosic biomass tracking, we have recently established a novel, rapid, high-throughput, easy-to-use, unambiguous and affordable approach to track lignocellulosic polymers at the surface of mechanically, chemically and enzymatically treated pulps.82,83 This approach is based on the use of four highly specific probes made of fluorescent-tagged carbohydrate binding modules. The CBM part of these genetically modified probes recognizes and binds to biopolymers (i.e. mannan, xylan, crystalline and amorphous cellulose) while the fluorescent protein part makes it possible to quickly detect and measure binding of probes to their intended targets. This approach, called fluorescent tagged CBM method (FTCM), proved to be instrumental for our understanding of lignocellulosic biomass processing, and exhibited both process optimizing and outcome predicting potential.82,83
Here we investigated the potential of FTCM for bolstering our understanding of hemicelluloses hydrolysis and factors that have an impact on such hydrolysis. To this end, we used two fluorescent-tagged fusion proteins of FTCM: mOrange2-CBM15 (OC15) and eCFP-CBM27 (CC27). The family 15 CBM (CBM15) is a xylan recognizing module of a xylanase (Xyn10C) from Cellvibrio japonicus84 and family 27 CBM (CBM27) consists of the mannan recognizing module of mannanase (Man5) from Thermotoga maritima.85 Both CBM15 and CBM27 are classified as type B CBMs and have been demonstrated to bind specifically to xylooligosaccharides and mannooligosaccharides, respectively.84,85 Mono-orange2 (mOrange2) and cyan fluorescent protein (CFP) were used as fluorescent proteins (detector molecules), and can be quantitatively measured with very high sensitivity and specificity, due to their independent fluorescent signals (each probe has its specific pair of emission and absorption maxima). For this study, we used four different pulp samples (unbleached mechanical pulp, bleached mechanical pulp, unbleached kraft pulp and bleached kraft pulp) to investigate and track variations in hemicelluloses after various treatments. Our results showed that FTCM can monitor the impact of mechanical and chemical treatment on the surface distribution of hemicelluloses, and helped understand and optimize enzymatic-induced hydrolysis of lignocellulosic polymers. We anticipate that FTCM can be developed into a monitoring tool for optimization of treatments and process strategies leading to a cost effective hydrolysis of hemicelluloses.
Experimental
Materials and methods
Chemicals and microbial strains.
Unless otherwise noted, all chemicals were reagent grade and purchased from Sigma-Aldrich and/or Fisher Scientific. Escherichia coli XL10 cells (Agilent Technologies) were used for all DNA manipulations while E. coli BL21-Gold(DE3)pLysS competent cells (Agilent Technologies) were used for recombinant proteins expression. Trichoderma viride xylanase (endo-1,4-β-xylanase) from glycoside hydrolase (GH) family 11 (E-XYTRI; Megazyme), Cellvibrio japonicus mannanase (endo-1,4-β-mannanase) from glycoside hydrolase (GH) family 26 (E-BMACJ; Megazyme) and Trichoderma reesei Celluclast 1.5L (C2730; Sigma-Aldrich) were used for the hydrolysis of lignocellulosic biomass. Carboxymethyl cellulose sodium salt (C5678; Sigma), xylan from beechwood (X4252; Sigma) and galactomannan (P-GALML; Megazyme) were used for affinity gel electrophoresis (AGE) and for enzymatic assays using the 3,5-dinitrosalicylic acid (DNS) method.
Construction, production and purification of CBM recombinant probes.
Probes were produced and purified from recombinant E. coli BL21-Gold(DE3)pLysS cells as described by Khatri et al. (2016)82 and Hébert-Ouellet et al. (2017)83 (note that in this study, probe eGFP-CBM3a was named GC3a; probe mOrange2-CBM15 was named OC15 and probe eCFP-CBM27 was named CC27, for the sake of simplicity). Following affinity and size exclusion chromatography steps, the probes purities were verified using SDS-PAGE (additional files 1 and 8). The amount of protein was quantified using the Bradford method.86 Concentrated protein solutions were stored at −80 °C following flash freezing.
Affinity gel electrophoresis (AGE).
AGE was used as described by Khatri et al. (2016)82 for qualitative assessment of the CC27 (10 μg) specificity toward selected ligands.
Isothermal titration calorimetry (ITC).
ITC was employed as described by Khatri et al. (2016)82 to measure the affinity of the CC27 probe towards selected hexaoses (xylohexaose (O-XHE; Megazyme), mannohexaose (O-MHE; Megazyme), cellohexaose (O-CHE; Megazyme)). All experiments were performed in triplicates. Data were analyzed and fitted using the NanoAnalyze software v2.3.6 (TA Instruments).
Pulp characterization.
The mechanical and kraft pulps used for this study were provided by an Eastern Canadian pulp and paper company. Both mechanical and kraft pulping were performed using a mixture of softwood (80–85%) and hardwood (20–15%). Four different grades of pulps: unbleached mechanical pulp (UBMP), bleached mechanical pulp (BMP), unbleached kraft pulp (UBKP) and bleached kraft pulp (BKP), were used. The cellulose, hemicellulose, and lignin contents of these pulps were analyzed in triplicates using NREL/TP-510-42618 protocol87 as described in Khatri et al. (2016).82
Handsheets preparation.
UBMP, BMP, UBKP and BKP were used as lignocellulosic substrates for the preparation of handsheets and paper discs. Handsheets (basis weight of 60 ± 2 g m−2) were prepared from pulps according to the Tappi standard method T 205 sp-02 as described in Khatri et al. (2016).82 These handsheets were then used for the preparation of the paper punches. These punches were defined as paper discs having a diameter of 3 mm.
Enzymatic digestion of paper discs.
The enzymatic digestions of paper discs were performed in triplicates using Trichoderma viride xylanase, Cellvibrio japonicus mannanase and Trichoderma reesei Celluclast 1.5L enzyme(s). Celluclast 1.5L is a mixture of fungal hydrolytic enzymes containing mostly two cellobiohydrolases, two endoglucanases and various accessory enzymes such as hemicellulases.27,88 We used the 3,5-dinitrosalicylic acid (DNS) method97 to monitor the accessory enzymes activities (such as xylanase and mannanase) in the Celluclast 1.5L (additional file 12). Xylanase and mannanase treatment concentrations ranged from 0.1 to 0.4 U per paper discs. Celluclast 1.5L enzyme was used at 0.1 U per paper discs. Cocktail CX was prepared by mixing Celluclast 1.5L (0.1 U per paper disc) with Trichoderma viride xylanase (0.1 U per paper disc). Cocktail CM was prepared by mixing Celluclast 1.5L (0.1 U per paper disc) with Cellvibrio japonicus mannanase (0.1 U per paper disc) and cocktail CXM was prepared by mixing Celluclast 1.5L (0.1 U per paper disc) with Trichoderma viride xylanase (0.1 U per paper disc) and Cellvibrio japonicus mannanase (0.1 U per paper disc). Units used here were as specified by respective enzyme suppliers. All experiments were performed with paper discs placed/glued at the bottom of 96-well black microtiter plate (Corning). All reactions were performed at room temperature or 50 °C, in sodium phosphate buffer (100 mM), pH 7.0 supplemented with 0.5 mg mL−1 BSA under continuous agitation (150 rpm) in order to reduce enzyme adsorption. Unless otherwise noted, after each enzymatic digestion, the reactions were removed and paper discs were washed (3 × 5 minutes) with buffer (20 mM Tris–HCl, pH 7.5 with 20 mM NaCl and 5 mM CaCl2) and later washed (3 × 5 minutes) with 0.05% (v/v) Tween 20. This buffer was shown to remove most proteins from paper discs in a previous report.83 Following Tween 20 washing, paper discs were washed again with buffer (without Tween) before analyzing the variations in biopolymers levels, or digested again with refreshing enzymes solution every hour to reach maximum possible hydrolysis when specified.
Lignocellulosic polymers tracking on the surface of paper discs using the OC15, CC27 and GC3a probes.
The FTCM tracking assay was performed as described by Khatri et al. (2016).82 All fluorescence readings were acquired at room temperature with a Synergy Mx microplate reader (BioTek). These fluorescence values were then converted into μg mm−2 using the appropriate standard curves (additional files 6, 7 and 9) and the surface area of the paper discs.
Results and discussion
Determination of the CC27 probe specificity using affinity gel electrophoresis (AGE)
In this study we have used three FTCM probes (GC3a, OC15 and CC27). GC3a and OC15 were previously characterized and shown to be specific to their intended target.82,83 Affinity gel electrophoresis (AGE) was used to qualitatively evaluate the specificity of the CC27 probe (mannan specific) towards soluble polysaccharides.89 In AGE, interactions between the studied protein and the gel-embedded polysaccharide are typically revealed by a reduced mobility compared to the mobility of the protein in absence of saccharide. Fig. 1 shows that CC27 interacts only with galactomannan (Fig. 1B). Similar to BSA, no binding was detected between CC27 and beechwood xylan (Fig. 1C) or carboxymethyl cellulose (CMC) (Fig. 1D). These results confirm that the well-known specific binding of CBM27 to mannan is unaltered by its fusion with CFP in the CC27 probe. BSA, which has no affinity towards carbohydrates, was used as negative control.89
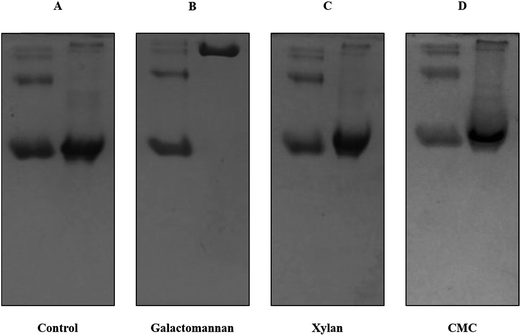 |
| Fig. 1 Affinity gel electrophoresis (AGE) of the CC27 probe. (Panel A) control (no polysaccharide), (panel B) galactomannan, (panel C) xylan, (panel D) CMC. In each panel the first well contained BSA (10 μg) and the second well was loaded with the CC27 probe (10 μg). All soluble polysaccharides were used at final concentration of 0.5% (w/v) and a 12% polyacrylamide gel was used for affinity analysis. | |
Determination of CC27 probe affinity using isothermal titration calorimetry (ITC)
The affinity of the recognition module of CC27 was investigated to quantify its sensitivity for a representative derivative of mannan. To this end, the affinity of CC27 toward various hexaoses was investigated by ITC (Table 1 and additional file 2). Analysis of the binding isotherms showed that the recognition module of CC27 probe bound tightly to mannohexaose (Ka = 692.6 × 103 M−1), but not to cellohexaose or xylohexaose (Table 1). The affinity value is similar to the one previously reported for CBM27 (Ka (×104 M−1) = 136.5 ± 17.68) confirming that the binding site of the recognition module of the CC27 probe is unaltered by its fusion with CFP.85
Table 1 Affinity of the CC27 probe for various hexaoses as determined by ITCa
Ligand |
K
a × 103 (M−1) |
K
d (M) |
n
|
n: number of ligand binding sites. NB: no binding detected. —: binding not detected.
|
Xylohexaose |
NB |
— |
— |
Mannohexaose |
692.6 ± 0.5 |
4.413 × 10−6 ± 0.2 |
1.1 ± 0.3 |
Cellohexaose |
NB |
— |
— |
Tracking hemicelluloses at the surface of wood biomass
Pulps composed of a mixture of softwood (80–85%) and hardwood (20–15%) from an Eastern Canadian paper mill were used as lignocellulosic biomass samples. Four different types of pulps were used in this study, allowing for the comparison of mechanically and chemically treated wood biomass: unbleached mechanical pulp (UBMP), bleached mechanical pulp (BMP), unbleached kraft pulp (UBKP) and bleached kraft pulp (BKP). These pulps were first investigated to determine differences in the hemicelluloses polymer content and their exposure at fiber surface.
Comparison of CC27 with OC15 binding to pulps (Fig. 2) revealed that mannan exposure is 2.3-fold higher than xylan exposure in both mechanical pulps (UBMP and BMP). Only minute differences were observed between exposures of both hemicelluloses studied here in kraft pulps: mannan exposure was 1.13-fold higher than xylan exposure in UBKP and only 1.08-fold higher in BKP (Fig. 2). The dominance of mannan for all pulps is compatible with the high softwood content of the four different pulps studied here.26 Our results also indicate that mannan was the dominant hemicellulose at the surface of mechanical pulps in agreement with an earlier study on lignin–hemicellulose complexes.38 Even though the pulp was primarily composed of softwood fibers, kraft processing led to the exposure of similar amounts of xylan and mannan on the surface of both kraft pulps (Fig. 2, UBKP and BKP). Note that the trends observed in FTCM signals, which responds to surface polymers, were in accordance with the bulk measurements of simple sugars by chemical composition analysis (NREL/TP-510-42618) of these pulps (additional file 3).
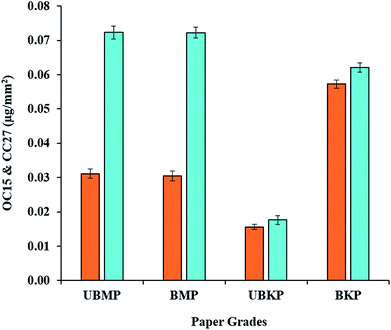 |
| Fig. 2 Tracking hemicelluloses on the surface of UBMP, BMP, UBKP and BKP papers using OC15 and CC27 probes. UBMP, BMP, UBKP and BKP paper discs were incubated with the OC15 probe (0.5 μg μL−1) (for xylan detection) and the CC27 probe (0.5 μg μL−1) (for mannan detection) for 1 h at room temperature under agitation. Three percent (w/v) milk (20 mM Tris–HCl, pH 7.5 with 20 mM NaCl and 5 mM CaCl2) was used to minimize the auto-fluorescence of paper discs and the non-specific binding of the OC15 and CC27 probes. The fluorescence values were converted to OC15 (μg mm−2) and CC27 (μg mm−2) by using the standard curves (additional file 6 and 7). Orange color represents the OC15 probe detection and cyan color represents the CC27 probe detection. Error bars represent the standard deviation. | |
Bleaching of mechanical pulp resulted in no significant difference between the exposure of xylan and mannan (Fig. 2). These results can be attributed to the pulping methodology involved. Mechanical pulping is a high yield process which tends to retain most wood constituents when producing UBMP and during the transformation of UBMP to BMP.90 FTCM indicates that the distribution of hemicelluloses at the surface of mechanically treated fibers were comparable to fiber bulk composition (revealed by NREL/TP-510-42618) (additional file 3).
The goal of kraft pulping process is to degrade and/or dissolve lignin with minimum dissolution or degradation of hemicelluloses.90 UBKP was characterized by the smallest exposure of hemicelluloses, a possible consequence of the dissolution or degradation of lignin–hemicelluloses complexes38 during kraft pulping. Bleaching of kraft pulp completely changed hemicelluloses exposure. As shown in Fig. 2, BKP binds 3.6-fold the amount of OC15 and 3.5-fold the amount of CC27 in comparison to UBKP, indicating that the surface exposure of xylan and mannan has increased after the bleaching process. Kraft pulping did not remove all lignin (lignin still represents 4.3% according to chemical composition analysis, additional file 3) in UBKP. Bleaching revealed additional hemicelluloses at the surface of BKP, resulting from the removal of this residual lignin. These “deep” hemicelluloses would become accessible after the full removal of lignin. Another explanation for this higher exposure or detection of hemicelluloses would involve mannan and xylan redeposition onto the surface of cellulose fibers during kraft processes.91–93
The strength of FTCM lies in its ability to detect changes in hemicelluloses exposure at the surface of fibers. While the trends observed were in general compatible with overall composition analysis (additional file 3), the amplitude of changes at the surface could not be predicted by chemical analysis. For instance, mannan dropped by 71% when comparing both mechanical pulps (UBMP and BMP) with UBKP (Fig. 2). Chemical analysis detected a mere decrease of 30–34% in mannan for the same comparison. In kraft pulps, the impact of bleaching on hemicelluloses exposure (an increase of 67%) (Fig. 2) could not be predicted by chemical composition analysis (showing an increase of only 13–15%, additional file 3). We also confirmed that the fluorescent proteins (alone, without CBM) did not bind to the biomass surface (data not shown), and we found no difference in binding signals, regardless of using the OC15 and CC27 probes together or separately (additional file 4 and 5). This indicates that the probes did not interfere with one another, as expected from the different targets to which they are specific. We also confirmed that there was no unspecific interactions between lignin and probes (data not shown).
We also investigated physical parameters such as roughness and porosity of paper discs made from pulps and their impact on probe binding. For the four pulps and all probes used in this study, we found that porosity had no obvious impact on probe binding (even when porosity was varied over a 40-fold range, see additional files 10 and 11†). Probes are much smaller than pores or crevices that may be of various sizes or numbers in pulps with different porosities. Roughness was found to vary by about 10% when we compared papers made from pulp in our experiments. Increased roughness did result into moderate increase of probe binding, but we did not observe any change in comparative binding of probes due to roughness (i.e. relative binding of a probe vs. binding of other probes is unchanged by roughness, see additional files 10 and 11†).
Investigation of reaction parameters by FTCM
Fig. 3 and 4 show probe binding to various pulps using increasing concentrations of enzymes. Our results revealed that the maximal impact of xylanase and mannanase enzymes on pulps were detected at the minimal loading used here (0.1 U of enzyme/paper disc). All the pulps (UBMP, BMP, UBKP and BKP) showed no significant loss in the exposure of xylan and mannan as we increased the concentration from 0.1 U to 0.4 U per paper disc. This suggests that a 0.1 U per paper disc concentration of both xylanase and mannanase was sufficient for the maximal digestion of available/exposed xylan and mannan. The impact of temperature was also studied. All the pulps showed maximal hydrolysis or decrease in the exposure of xylan and mannan at 50 °C. This suggests that both xylanase and mannanase enzymes were comparatively more active at 50 °C than at room temperature. At room temperature, an overnight treatment showed relatively higher decrement in the exposure of hemicelluloses than a treatment duration of one hour. In contrast, at 50 °C, both 1 h and overnight treatments led to a maximal and similar decrement for both xylanase and mannanase enzymes treatments. FTCM unambiguously reveals that using 0.1 U of enzyme/paper disc for 1 h at 50 °C was sufficient for maximal reduction in available/exposed xylan and mannan. Production of reducing sugars by enzymatic hydrolysis was also monitored using the DNS method. Conditions chosen for this control experiment were the ones that lead to maximal removal of xylan (Fig. 3D). Using same biomass and same treatments, we found that reducing sugar production correlated with the decrease in xylan as detected by the probes (see additional file 13†).
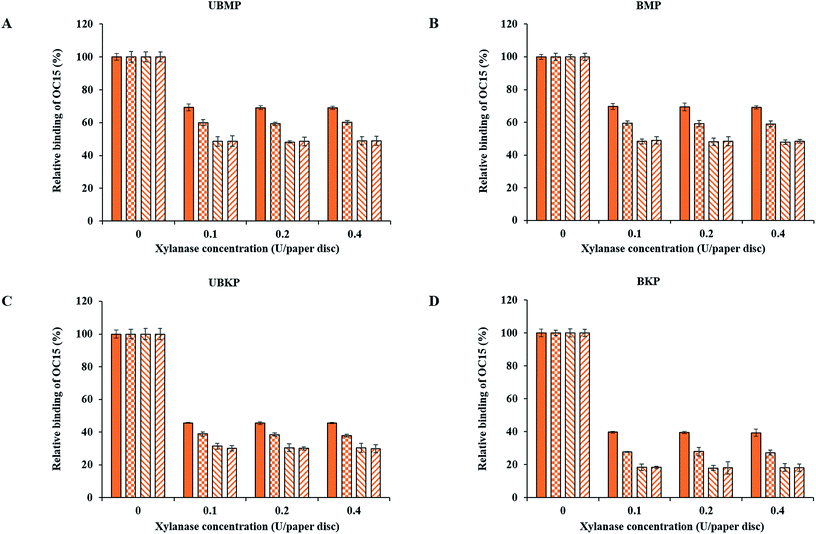 |
| Fig. 3 Tracking xylan for optimizing hydrolysis conditions for xylanase treatments using the OC15 probe. (A) UBMP, (B) BMP, (C) UBKP and (D) BKP paper discs were incubated with xylanase (0.1, 0.2 and 0.4 U per paper disc) at four different opted conditions (1 h; RT ( ), overnight; RT ( ), 1 h; 50 °C ( ) and overnight; 50 °C ( )) under continuous agitation (150 rpm). Following this, untreated and treated paper discs were incubated with the OC15 probe (0.5 μg μL−1) for 1 h at room temperature under agitation to detect xylan exposure. Three percent (w/v) milk (20 mM Tris–HCl, pH 7.5 with 20 mM NaCl and 5 mM CaCl2) was used to minimize the auto-fluorescence of paper discs and the non-specific binding of the OC15 probe. Orange color represents the OC15 probe detection. Error bars represent the standard deviation. | |
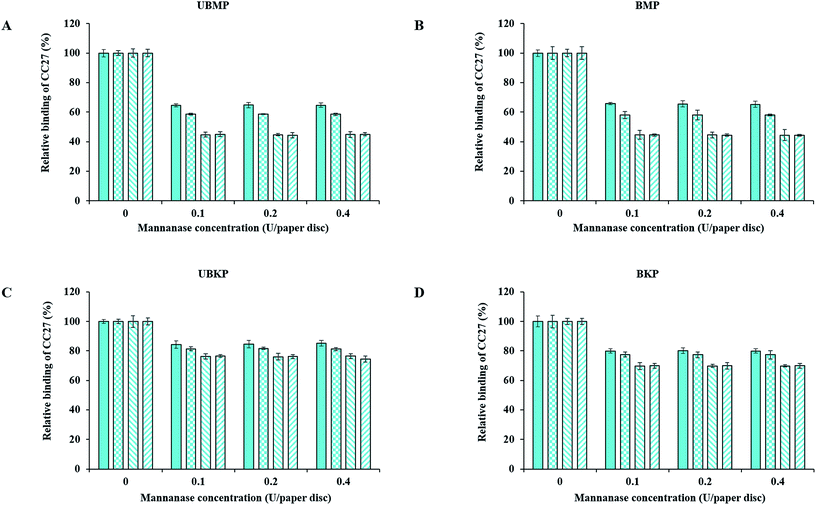 |
| Fig. 4 Tracking mannan for optimizing hydrolysis conditions for mannanase treatments using the CC27 probe. (A) UBMP, (B) BMP, (C) UBKP and (D) BKP paper discs were incubated with mannanase (0.1, 0.2 and 0.4 U per paper disc) at four different opted conditions (1 h; RT ( ), overnight; RT ( ), 1 h; 50 °C ( ) and overnight; 50 °C ( )) under continuous agitation (150 rpm). Following this, untreated and treated paper discs were incubated with the CC27 probe (0.5 μg μL−1) for 1 h at room temperature under agitation to detect mannan exposure. Three percent (w/v) milk (20 mM Tris–HCl, pH 7.5 with 20 mM NaCl and 5 mM CaCl2) was used to minimize the auto-fluorescence of paper discs and the non-specific binding of the CC27 probe. Cyan color represents the CC27 probe detection. Error bars represent the standard deviation. | |
The xylanase enzyme appeared to hydrolyze xylan more efficiently in BKP than all the other pulps (Fig. 3). Xylanase-treated BKP paper discs showed a maximum decrement of 82% in the exposure of xylan. This may be ascribed to the most efficient removal of lignin in BKP compared to all other pulps studied here, as described earlier (Fig. 2 and additional file 3). In contrast, the mannanase enzyme was more efficient on both mechanical pulps (UBMP and BMP) (Fig. 4) due to the very high exposure of mannan in mechanical pulps as described earlier (Fig. 2 and additional file 3). Both UBMP and BMP paper discs showed a maximum decrement of at least 55% in the exposure of mannan after mannanase hydrolysis under our assay conditions. Although hemicelluloses were detectable by our probes, these were not completely hydrolyzed or reachable by the enzyme used. This suggests a possible hindrance or inactivation of enzymes during our assay.
Mannanase hydrolysis showed that the relative amounts of mannan removed from mechanical pulps are higher than the percentage removed from the kraft pulps (Fig. 4). This suggests a higher digestibility of lignin-associated mannan (lignin–hemicelluloses complexes) in mechanical pulp. The hydrolysis of mannan polymers in kraft pulps might be hindered by significant exposure of xylan (mostly associated to cellulose–hemicelluloses complexes), in agreement with an earlier study on lignin–hemicellulose complexes.38
In contrast, xylanase hydrolysis showed that xylan was more susceptible to hydrolysis in kraft pulps compared to mannan. In kraft pulps, bleaching increased the exposure of xylan and eventually increased hydrolysis of xylan by a few percentage points. This suggests that after removal of lignin and the so-called lignin–hemicelluloses complexes via kraft pulping, xylan is more vulnerable or exposed at the surface of kraft pulps fibers than mannan. The xylanase hydrolysis in mechanical pulps seems to be hindered by the abundance of mannan, which is the main hemicellulose associated with lignin and/or lignin–hemicelluloses complexes, as shown by the lower binding of OC15 to xylan in both mechanical pulps (Fig. 2). This study revealed that FTCM, a rapid and high throughput approach, can improve our knowledge and understanding of biomass hydrolysis as well as the economic feasibility of lignocellulosic biomass based industries.
Addressing possible impact of enzyme inactivation
Above results suggested that neither of these enzymes were able to completely eliminate all the available hemicelluloses at the surface of fibers under our conditions. This might be explained, in part, by the inactivation of enzymes by reaction products, plant derived inhibitors, adsorption to fibers or denaturation of the enzymes over time.41 Recent studies have suggested that enzyme inhibition by their own end products and other components, generated during the bioconversion process, can be a key factor which impedes the hydrolysis processes.40,41,46–55 To further investigate a potential inactivation scenario, we chose BKP paper discs and treated them using optimum hydrolysis conditions (0.1 U of enzyme/paper disc, 50 °C). After a one-hour treatment, the enzymatic reactions were removed and the surfaces of the paper discs were tracked with the OC15 and CC27 probes, individually, for residual hemicelluloses. The tracking of xylan and mannan showed an 80% and 30% decrement, respectively (Fig. 5) (similar to the results described in Fig. 3D and 4D). Prolonging enzymatic reactions longer than one hour (and/or overnight) at 50 °C did not promote any further drop in the exposure of hemicelluloses. Therefore, we washed paper discs to remove the inactivated enzyme which might be inhibiting hydrolysis. To this end, the BKP paper discs, which were already incubated with enzymes for an hour, were washed with buffer and Tween (0.05%) before adding another load of freshly prepared enzymes. After refreshing enzymes (xylanase or mannanase) solution, the reactions were kept again under optimum hydrolysis conditions (0.1 U of enzyme/paper disc, 50 °C) for another hour. Later, the tracking of xylan and mannan showed an additional decrement of 2.3% and 5.3%, respectively, in the exposure of hemicelluloses (Fig. 5). Likewise, the enzymatic reactions were washed again before adding fresh enzymes for an additional one-hour reaction period and then measured by probes, for up to 24 hours. The results exhibited a gradual decrement in the binding of OC15 and CC27 probes. After 24 hours, the maximum decrement in the exposure of xylan was 92% and 50% in the exposure of mannan. The maximal impact was reached after 12 hours (in the case of xylanase hydrolysis) and 5 hours (in the case of mannanase hydrolysis), regardless of washing paper discs and adding freshly prepared enzymes (Fig. 5). This suggests that the xylanase and mannanase enzymes might have reached their maximum possible hydrolysis activity, or that such activity had no more detectable impact on the hemicelluloses. Nevertheless, FTCM indicates that there was an inactivation (and/or inhibition) which was somewhat overcome by washing followed by a fresh load of enzyme (xylanase or mannanase). FTCM reveals that despite an apparent interruption of net hydrolysis, there remains a large amount of mannan at the surface of BKP fibers. Such information cannot be provided by chromatographic analysis of hydrolysis products. Chemical analysis, which depends on total hemicellulose content would not be as sensitive as FTCM for detecting changes in hemicellulose hydrolysis at surface and for optimizing enzymatic processes. Note that measurements of reducing sugars production by enzymes were performed allowing to confirm that smaller quantities of sugars were released upon renewing enzymes, but that maximal production of sugars was generated in the first hour with the first exposure to enzyme (additional files 14 and 15†).
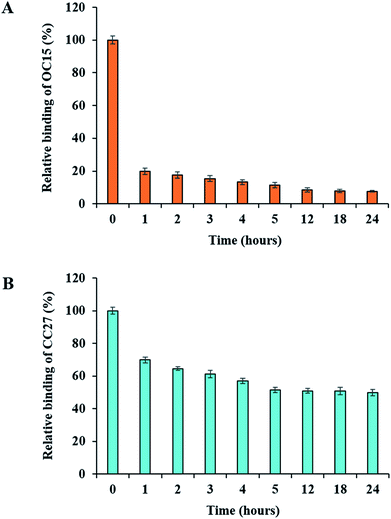 |
| Fig. 5 Tracking (A) xylan and (B) mannan to address the impact of xylanase and mannanase inactivation on BKP paper discs using the OC15 and CC27 probes. (A) Paper discs were incubated with xylanase (0.1 U per paper disc) at 50 °C under ontinuous agitation (150 rpm) with refreshing enzyme solution every hour up to 24 hours. Following this, untreated and xylanase treated BKP paper discs were incubated with the OC15 probe (0.5 μg μL−1) for 1 h at room temperature under agitation. (B) Paper discs were incubated with mannanase (0.1 U per paper disc) at 50 °C under continuous agitation (150 rpm) with refreshing enzyme solution every hour up to 24 hours. Following this, untreated and mannanase treated BKP paper discs were incubated with the CC27 probe (0.5 μg μL−1) for 1 h at room temperature under agitation. For fluorescence measurements, three percent (w/v) milk (in a buffer made of 20 mM Tris–HCl, pH 7.5 with 20 mM NaCl and 5 mM CaCl2) was used to minimize the auto-fluorescence of paper discs and non-specific binding of the probes. Orange color ( ) and cyan color ( ) represent the OC15 and CC27 probes detection, respectively. Error bars represent the standard deviations. | |
Investigating the impact of cellulose on the hydrolysis of hemicelluloses
Despite finding out the optimum hydrolysis conditions and achieving additional removal of hemicelluloses by spiking enzymes, both xylanase and mannanase enzymes were unable to completely eliminate/hydrolyze all the available hemicelluloses that could be detected by FTCM. To achieve additional hydrolysis of hemicelluloses and simultaneously improve our understanding of fiber deconstruction, we explored the potential impact of cellulase treatments on hemicelluloses availability. The use of enzyme cocktails comprised of cellulase and so-called accessory enzymes (xylanase and/or mannanase) has been previously studied and found to enhance the cellulose hydrolysis.27,29,35,37–40,42,43 Here we reexamined the apparent complementarity between enzymes using FTCM. To this end, BKP paper discs were hydrolyzed with commercial cellulase enzyme (Celluclast 1.5L) under conditions which were optimal for hemicelluloses hydrolysis (0.1 U per paper disc, 50 °C) for an hour. Celluclast 1.5L possesses some contaminant activity of both xylanase and mannanase (additional file 12). After hydrolysis, an eGFP-CBM3a (named GC3a here) probe was used to track the exposure of crystalline cellulose (as described by Hébert-Ouellet et al. 2017).83 Results in Fig. 6A suggest that Celluclast 1.5L treatment reduced exposure of crystalline cellulose by 63%. Then, supplementation of Celluclast 1.5L with a xylanase accessory enzyme (cocktail CX) and a mannanase accessory enzyme (cocktail CM) was investigated. Addition of hemicellulases led to an additional reduction of exposed cellulose, suggesting that they helped the hydrolysis of cellulose by the Celluclast 1.5L enzyme (Fig. 6A). Finally, the supplementation of Celluclast 1.5L with both xylanase and mannanase enzymes (cocktail CXM) drastically decreased the cellulose exposure (down by 88%, see Fig. 6A). These results confirm that to achieve highest cellulose hydrolysis at surface of BKP fibers, it is vital to supplement cellulase with accessory enzymes such as xylanase and mannanase. These results also suggest that not only one type but both types of hemicelluloses (xylan and mannan) restrict cellulose accessibility or cellulase action.
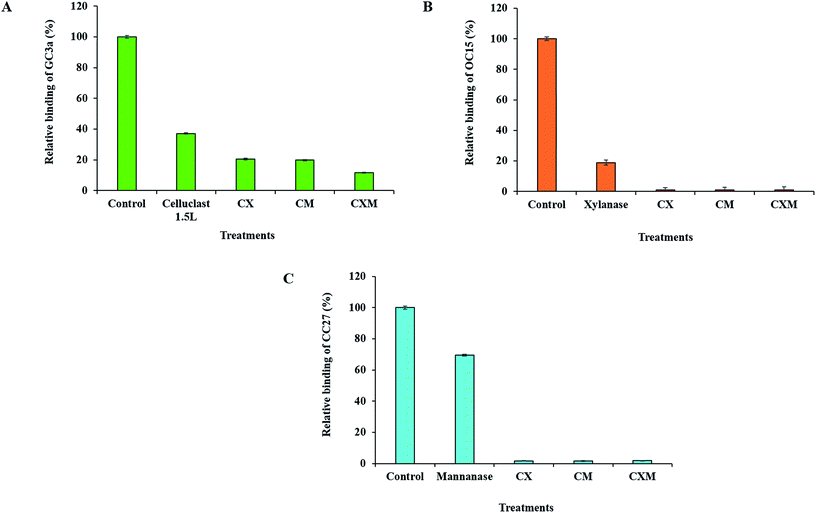 |
| Fig. 6 Impact of Celluclast 1.5L, xylanase, mannanase and their cocktails hydrolysis of BKP on the exposure of (A) crystalline cellulose, (B) xylan and (C) mannan polymers. (A) Paper discs were incubated with Celluclast 1.5L (0.1 U per paper disc), cocktail CX, cocktail CM and cocktail CXM at 50 °C for 1 h under continuous agitation (150 rpm). Following this, untreated and treated BKP paper discs were incubated with the GC3a probe (0.5 μg μL−1) for 1 h at room temperature under agitation. (B) Paper discs were incubated with xylanase (0.1 U per paper disc), cocktail CX, cocktail CM and cocktail CXM at 50 °C for 1 h under continuous agitation (150 rpm). Following this, untreated and treated BKP paper discs were incubated with the OC15 probe (0.5 μg μL−1) for 1 h at room temperature under agitation. (C) Paper discs were incubated with mannanase (0.1 U per paper disc), cocktail CX, cocktail CM and cocktail CXM at 50 °C for 1 h under continuous agitation (150 rpm). Following this, untreated and treated BKP paper discs were incubated with the CC27 probe (0.5 μg μL−1) for 1 h at room temperature under agitation. Green ( ), orange ( ) and cyan ( ) color were used for GC3a, OC15 and CC27 probes detection, respectively. Error bars represent the standard deviations. | |
We then investigated a potential reciprocal additive and/or synergistic action by supplementing xylanase and mannanase enzymes with Celluclast 1.5L and monitoring hemicelluloses removal after treatment. Using the same BKP paper discs treated as explained above, we found that the xylanase enzyme led to 81% decrement in the exposure of xylan (Fig. 6B), which is compatible with the result shown in Fig. 3D. BKP paper discs were also treated with cocktail CX, cocktail CM and cocktail CXM and all led to the nearly complete elimination of xylan at the surface of BKP fibers. Likewise, BKP paper discs hydrolyzed with the mannanase enzyme showed 30% decrement in mannan exposure (Fig. 6C). Subsequently, cocktail CX, cocktail CM and cocktail CXM promoted further hydrolysis of BKP paper, leading to the near complete elimination of mannan from the surface of BKP fibers. These results suggest that cellulose is an important barrier limiting access to hemicelluloses in BKP.
The presence of both hemicellulases (xylanase and mannanase) were required with Celluclast 1.5L for maximal hydrolysis of crystalline cellulose. In contrast, either one of the hemicellulases or both were required with Celluclast 1.5L for maximal hydrolysis of xylan and mannan. This suggests that xylan and mannan were providing protection to cellulose, but that a portion of cellulose remains protected by other fiber components, or that a portion of exposed cellulose remains stable despite enzymatic attack. Such results are compatible with the existence of deeply embedded hemicelluloses, part of a carbohydrate–hemicellulose complexes, shielding the cellulose fibers. Note that in BKP, the hemicelluloses associated with lignin are expected to be mostly absent. These results suggest that FTCM can also be used to design enzyme cocktails preparations for specific applications. Exposure of fibers to enzymes had a drastic impact on all polysaccharides at surface. The question of “what is left” at surface after such important decrease in probe binding is legitimate but not necessarily relevant. Note that when preparing paper discs prior to FTCM reading, the discs were washed, and any loosen material was removed. Hydrolysis of high surface fragments such as microfibrils and their removal prior to FTCM may explain the important decrease in probe binding observed here.
Exploring the impact of xylan polymers on the hydrolysis of mannan
The previous section has revealed the proximity of cellulose and “deep” hemicelluloses. Here we focused on the impact of xylan on the hydrolysis of mannan in order to address structural relationships and their potential impact on hydrolysis yield. To this end, BKP paper discs were hydrolyzed with mannanase enzyme as a control reaction. Results showed a decrement of at least 30% in the exposure of mannan for all the enzyme concentrations used (Fig. 7A, solid cyan color bars). The results were as expected and fully compatible with Fig. 4D.
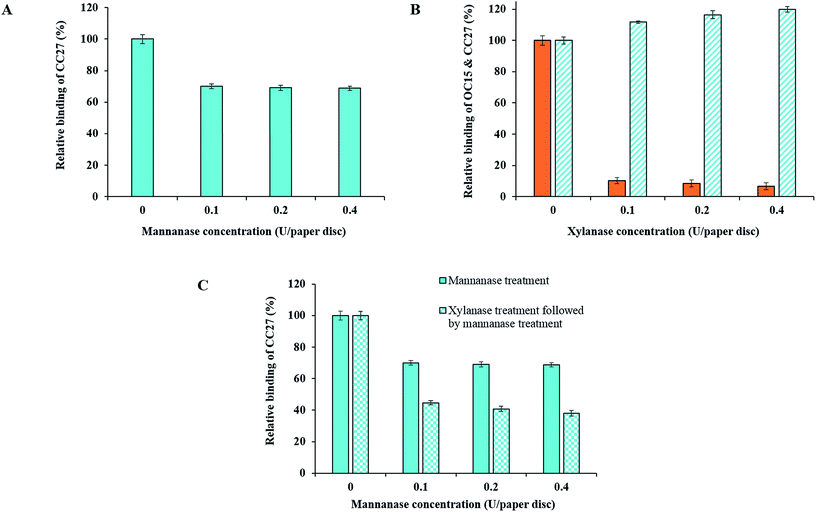 |
| Fig. 7 Impact of mannanase and xylanase hydrolysis of BKP on the exposure of mannan polymers. (A) Untreated and mannanase treated (0.1, 0.2 and 0.4 U per paper disc, at 50 °C for 1 h) BKP paper discs were incubated with the CC27 probe (0.5 μg μL−1) for 1 h at room temperature under agitation to detect mannan exposure ( ). (B) Untreated and xylanase treated BKP paper discs (0.1, 0.2 and 0.4 U per paper disc, at 50 °C with refreshing enzyme solution every hour up to 12 hours) were incubated with the OC15 probe (0.5 μg μL−1) for 1 h at room temperature under agitation ( ) for xylan detection. The same xylanase hydrolyzed BKP paper discs were also incubated with the CC27 probe (0.5 μg μL−1) to detect mannan exposure ( ). (C) Previously xylanase treated BKP paper discs (as described in panel B) were later treated with mannanase enzyme (0.1, 0.2 and 0.4 U per paper disc, at 50 °C for 1 h). Following this, untreated and mannanase treated BKP paper discs were incubated with CC27 probe (0.5 μg μL−1) ( ). Data of panel A ( ) is also shown in panel C for comparison. Orange color ( ) represents the OC15 probe detection and cyan color ( ) represents the CC27 probe detection. Error bars represent the standard deviations. | |
In another reaction, BKP paper discs were digested with the xylanase enzyme. In order to reach the maximum xylan hydrolysis, the reaction was performed as described above (section Addressing possible impact of enzyme inactivation) with refreshing enzyme solution every hour up to 12 hours. By refreshing enzyme solution, this treatment led to a stronger reduction in xylan exposure (an additional 10–13%) as detected with the OC15 probe (Fig. 7B, solid orange color bars). The results were as expected and fully compatible with Fig. 5A. The same xylanase hydrolyzed paper discs were then inspected using the CC27 probe to track mannan exposure. The results revealed that mannan exposure at the surface of xylanase hydrolyzed BKP paper discs increased by 20% when pulp was treated with a 0.4 xylanase U per paper disc (Fig. 7B, cyan color bars with upward diagonal stripes). Xylanase hydrolysis for 1 h or overnight, without refreshing enzyme solution (as described above in section Investigation of reaction parameters by FTCM), lead to no significant mannan exposure at the surface of xylanase hydrolyzed BKP paper discs (data not shown).
In another reaction, the xylanase hydrolyzed BKP paper discs were later washed and exposed to the hydrolysis with mannanase enzyme. In this case, mannan exposure decreased by 55% (in case of 0.1 mannanase U per paper disc) which is an additional decrement of 25% compared to when xylanase was not used as a pretreatment (Fig. 7C, cyan color bars with checker board). This study showed that xylan hydrolysis exposed more detectable mannan on the surface of BKP wood fibers, leading to higher enzymatic hydrolysis of mannan. This suggests that xylan was wrapping or covering mannan in the so-called cellulose–hemicellulose complexes. The mannan detected here seems to be deeply embedded in the BKP wood fibers.
Plant cell wall organization directly affects the nature of biomass recalcitrance.20,21 Therefore, it is important to understand the organization of hemicelluloses and FTCM offers a unique means of addressing this issue. So far, various studies looking for the arrangement of the different wood polymers in delignified samples have revealed that mannan is closely associated with cellulose and xylan is more associated with condensed lignin in the secondary cell wall of the softwood fibers.44,94 This FTCM study provides direct evidence in support of this suggested model. Using FTCM probes, we detected two different mannan populations in the wood fibers. The first mannan population was associated with lignin–hemicellulose complexes as seen in mechanical pulps. This population was dominant at the outer surface of wood fibers. In contrast, during kraft pulping, lignin and lignin–hemicellulose complexes get dissolved and/or degraded, which exposes deeper hemicelluloses. The second mannan population was found deeper in fiber, probably associated to the earlier proposed cellulose–hemicellulose complexes in kraft pulps. Overall the results suggest that xylan was acting as a physical shield which was covering or wrapping the mannan polymers in BKP fibers. This also indicates that the second population of mannan is hidden beneath the surface of xylan polymers, closely associated to cellulose or deeply embedded in the cell wall organization in BKP fibers. FTCM analysis fully supports the concept of a complex network of hemicelluloses around the cellulose fibers as reported by Varnai et al. (2011)43 but in addition, FTCM highlighted the presence of deeply embedded hemicelluloses. Working with bleached softwood dissolving pulp, Gübitz et al. (1998)38 proposed that hemicelluloses hydrolysis is stronger when cellulase enzymes are used with hemicellulases and proposed two different fractions of hemicelluloses: one associated with lignin and another one with cellulose. The FTCM results are compatible with the findings of Gübitz et al. (1998)38 which suggested two different mannan populations. This study also provides a rationale for the findings of Kansoh et al. (2004)95 and Clarke et al. (2000)96 which indicated that the use of the mannanase enzyme is not very efficient if xylan is still present in kraft pulps.
Overall, FTCM has been shown to help understanding cell wall ultrastructure and its organization by studying the presence of hemicelluloses in various parts of fibers, as lignin is progressively removed by kraft pulping and bleaching processes. Although most FTCM investigations reported here were compatible with chemical analysis (dependent on overall/bulk composition analysis; NREL/TP-510-42618), they revealed that changes in surface hemicelluloses after various treatments are much more important than indicated by chemical analysis. Monitoring surface modifications is much more informative on biomass recalcitrance than performing analysis of fiber bulk composition.
Conclusion
FTCM showed that it can specifically track mechanical, chemical and enzymatic-induced variations of hemicelluloses on the surface of different wood fibers in a rapid and high throughput format. Optimum hydrolysis parameters for both xylanase and mannanase enzymes, for all the studied pulps, were 0.1 U of enzyme/paper disc at 50 °C for a treatment duration of 1 h. FTCM identified the major factor limiting hydrolysis efficiency as enzyme inactivation (by any mechanism). By directly detecting polymers remaining after various enzymatic treatments, using CBM probes revealed additive and/or synergistic interactions between Celluclast 1.5L, xylanase and mannanase enzymes. The ability of FTCM to directly map layers of cellulose and hemicelluloses fractions as they were attacked by enzymes provided support for an embedded population of mannan, protected by xylan, probably associated to cellulose–hemicellulose complexes.38 We believe that this method can enhance our understanding of lignocellulosic polymers response to various treatments, therefore bolstering development of cost-effective processes for production of biofuels and other lignocellulosic biomass-based products.
List of abbreviations
AGE | Affinity gel electrophoresis |
BSA | Bovine serum albumin |
BKP | Bleached kraft pulp |
BMP | Bleached mechanical pulp |
CAZy | Carbohydrate active enzymes |
CBMs | Carbohydrate binding modules |
CBM15 | Family 15 carbohydrate binding module |
CMC | Carboxymethyl cellulose |
CFP | Cyan fluorescent protein |
DNS | 3,5-Dinitrosalicylic acid |
GFP | Green fluorescent protein |
GH | Glycoside hydrolase |
IPTG | Isopropyl-β-D-thiogalactopyranoside |
ITC | Isothermal titration calorimetry |
LB | Luria–Bertani |
mOrange2 | Mono-orange2 |
NREL | National renewable energy laboratory |
CC27 | Cyan fluorescent protein linked to a family 27 carbohydrate binding module |
GC3a | Green fluorescent protein linked to a family 3a carbohydrate binding module |
OC15 | Mono-orange2 fluorescent protein linked to a family 15 carbohydrate binding module |
SDS-PAGE | Sodium dodecyl sulfate – polyacrylamide gel electrophoresis |
UBKP | Unbleached kraft pulp |
UBMP | Unbleached mechanical pulp. |
Authors' contributions
VK carried out all the experiments and drafted the manuscript. FMM and MB helped to draft and revise the manuscript. All authors read and approved the final manuscript.
Conflicts of interest
VK and MB declare that they have no competing interests. FMM was an employee of Buckman North America which financially supported parts of this work.
Acknowledgements
This research was supported in part by Buckman Laboratories of Canada and funded by grants from CRIBIQ and NSERC (CRDPJ445143-12). We would like to thank Dr Roberto Chica (University of Ottawa) for the donation of fluorescent protein and Dr Nicolas Doucet (INRS-Institut Armand-Frappier) for his expert assistance while performing isothermal titration calorimetry (ITC) measurements. The skillful technical assistance of Nikolas Beauchesne, Vincent Bolduc, and Guillaume L. Lemieux is acknowledged. We would like to thank Ojas Bhatia for his valuable editorial contributions.
Notes and references
- M. E. Himmel, S. Y. Ding, D. K. Johnson, W. S. Adney, M. R. Nimlos, J. W. Brady and T. D. Foust, Science, 2007, 315, 804–807 CrossRef CAS PubMed.
- C. E. Wyman, Trends Biotechnol., 2007, 25(4), 153–157 CrossRef CAS PubMed.
- L. R. Lynd, M. S. Laser, D. Bransby, B. E. Dale, B. Davison, R. Hamilton, M. Himmel, M. Keller, J. D. McMillan, J. Sheehan and C. E. Wyman, Nat. Biotechnol., 2008, 26(2), 169–172 CrossRef CAS PubMed.
- L. R. Lynd, J. H. Cushman, R. J. Nichols and C. E. Wyman, Science, 1991, 251(4999), 1318–1323 CAS.
- H. Jørgensen, J. B. Kristensen and C. Felby, Biofuels, Bioprod. Biorefin., 2007, 1, 119–134 CrossRef.
- C. E. Wyman, Bioresour. Technol., 1994, 50(1), 3–16 CrossRef CAS.
- A. E. Farrell, R. J. Plevin, B. T. Turner, A. D. Jones, M. O'hare and D. M. Kammen, Science, 2006, 311(5760), 506–508 CrossRef CAS PubMed.
- R. Kumar, M. Tabatabaei, K. Karimi and I. Sárvári Horváth, Biofuel Res. J., 2016, 3(1), 347–356 CrossRef CAS.
- A. Culbertson, M. Jin, L. da Costa Sousa, B. E. Dale and V. Balan, RSC Adv., 2013, 3(48), 25960–25969 RSC.
- Y. Hong, A. S. Nizami, M. Pour bafrani, B. A. Saville and H. L. MacLean, Biofuels, Bioprod. Biorefin., 2013, 7(3), 303–313 CrossRef CAS.
- L. R. Lynd, C. E. Wyman and T. U. Gerngross, Biotechnol. Prog., 1999, 15(5), 777–793 CrossRef CAS PubMed.
-
R. Kumar and C. E. Wyman, Bioalcohol production: Biochemical conversion of lignocellulosic biomass, ed. K. Waldon, Woodhead publishing limited, Oxford, 2010, pp. 73–121 Search PubMed.
-
R. Kumar and C. E. Wyman, Aqueous Pretreatment of Plant Biomass for Biological and Chemical Conversion to Fuels and Chemicals, ed. C. E. Wyman, John Wiley and Sons, Ltd, 2013, pp. 281–310 Search PubMed.
- P. A. Penttilä, A. Várnai, J. Pere, T. Tammelin, L. Salmén, M. Siika-aho, L. Viikari and R. Serimaa, Bioresour. Technol., 2013, 129, 135–141 CrossRef PubMed.
- M. Li, M. Tu, D. Cao, P. Bass and S. Adhikari, J. Agric. Food Chem., 2013, 61(3), 646–654 CrossRef CAS PubMed.
- K. Igarashi, T. Uchihashi, A. Koivula, M. Wada, S. Kimura, T. Okamoto, M. Penttilä, T. Ando and M. Samejima, Science, 2011, 333, 1279–1282 CrossRef CAS PubMed.
- L. Zhu, J. P. O'Dwyer, V. S. Chang, C. B. Granda and M. T. Holtzapple, Bioresour. Technol., 2008, 99, 3817–3828 CrossRef CAS PubMed.
- S. D. Mansfield, C. Mooney and J. N. Saddler, Biotechnol. Prog., 1999, 15, 804–816 CrossRef CAS PubMed.
- M. Hall, P. Bansal, J. H. Lee, M. J. Realff and A. S. Bommarius, FEBS J., 2010, 277(6), 1571–1582 CrossRef CAS PubMed.
- S. P. Chundawat, B. S. Donohoe, L. da Costa Sousa, T. Elder, U. P. Agarwal, F. Lu, J. Ralph, M. E. Himmel, V. Balan and B. E. Dale, Energy Environ. Sci., 2011, 4(3), 973–984 CAS.
- J. D. DeMartini, S. Pattathil, J. S. Miller, H. Li, M. G. Hahn and C. E. Wyman, Energy Environ. Sci., 2013, 6(3), 898–909 CAS.
- A. T. W. M. Hendriks and G. Zeeman, Bioresour. Technol., 2009, 100(1), 10–18 CrossRef CAS PubMed.
- S. Subramaniyan and P. Prema, Crit. Rev. Biotechnol., 2002, 22(1), 33–64 CrossRef CAS PubMed.
- B. C. Saha, J. Ind. Microbiol. Biotechnol., 2003, 30(5), 279–291 CrossRef CAS PubMed.
- F. M. Gírio, C. Fonseca, F. Carvalheiro, L. C. Duarte, S. Marques and R. Bogel-Łukasik, Bioresour. Technol., 2010, 101(13), 4775–4800 CrossRef PubMed.
- T. E. Timell, Wood Sci. Technol., 1967, 1(1), 45–70 CrossRef CAS.
- J. Hu, V. Arantes and J. N. Saddler, Biotechnol. Biofuels, 2011, 4(1), 1–14 CrossRef CAS PubMed.
- M. J. Selig, T. B. Vinzant, M. E. Himmel and S. R. Decker, Appl. Biochem. Biotechnol., 2009, 155(1–3), 397–406 CAS.
- R. Bura, R. Chandra and J. Saddler, Biotechnol. Prog., 2009, 25(2), 315–322 CrossRef CAS PubMed.
- M. Frommhagen, S. Sforza, A. H. Westphal, J. Visser, S. W. Hinz, M. J. Koetsier, W. J. van Berkel, H. Gruppen and M. A. Kabel, Biotechnol. Biofuels, 2015, 8(1), 1–12 CrossRef CAS PubMed.
- A. Suurnakki, T. Q. Li, J. Buchert, M. Tenkanen, L. Viikari, T. Vuorinen and L. Odberg, Holzforschung, 1997, 51(1), 27–33 CrossRef CAS.
- J. P. Vincken, A. de Keizer, G. Beldman and A. G. J. Voragen, Plant Physiol., 1995, 108(4), 1579–1585 CrossRef CAS PubMed.
- T. Oksanen, J. Buchert and L. Viikari, Holzforschung, 1997, 51(4), 355–360 CrossRef CAS.
- T. Kohnke, K. Lund, H. Brelid and G. Westman, Carbohydr. Polym., 2010, 81(2), 226–233 CrossRef.
- J. Zhang, M. Siika-aho, M. Tenkanen and L. Viikari, Biotechnol. Biofuels, 2011, 4(1), 1–10 CrossRef PubMed.
- A. Berlin, V. Maximenko, N. Gilkes and J. Saddler, Biotechnol. Bioeng., 2007, 97(2), 287–296 CrossRef CAS PubMed.
- K. Ohgren, R. Bura, J. Saddler and G. Zacchi, Bioresour. Technol., 2007, 98(13), 2503–2510 CrossRef PubMed.
- G. M. Gübitz, D. W. Stebbing, C. I. Johansson and J. N. Saddler, Appl. Microbiol. Biotechnol., 1998, 50(3), 390–395 CrossRef.
- M. P. Garcia-Aparicio, M. Ballesteros, P. Manzanares, I. Ballesteros, A. Gonzalez and M. J. Negro, Appl. Biochem. Biotechnol., 2007, 137, 353–365 CrossRef PubMed.
- R. Kumar and C. E. Wyman, Biotechnol. Prog., 2009a, 25(2), 302–314 Search PubMed.
- R. Kumar and C. E. Wyman, Biotechnol. Bioeng., 2014, 111(7), 1341–1353 CrossRef CAS PubMed.
- Q. Qing and C. E. Wyman, Biotechnol. Biofuels, 2011, 4(1), 18 CrossRef CAS PubMed.
- A. Varnai, L. Huikko, J. Père, M. Siika-aho and L. Viikari, Bioresour. Technol., 2011, 102(19), 9096–9104 CrossRef CAS PubMed.
- M. Åkerholm and L. Salmén, Polymer, 2001, 42(3), 963–969 CrossRef.
- D. Klein-Marcuschamer, P. Oleskowicz-Popiel, B. A. Simmons and H. W. Blanch, Biotechnol. Bioeng., 2012, 109(4), 1083–1087 CrossRef CAS PubMed.
- E. Ximenes, Y. Kim, N. Mosier, B. Dien and M. Ladisch, Enzyme Microb. Technol., 2010, 46, 170–176 CrossRef CAS.
- Y. Kim, E. Ximenes, N. S. Mosier and M. R. Ladisch, Enzyme Microb. Technol., 2011, 48(4–5), 408–415 CrossRef CAS PubMed.
- M. Mandels and E. T. Reese, Annu. Rev. Phytopathol., 1965, 3, 85–102 CrossRef CAS.
- E. T. Reese and M. Mandels, Biotechnol. Bioeng., 1980, 22(2), 323–335 CrossRef CAS PubMed.
- G. Halliwell and M. Griffin, Biochemistry, 1973, 135(4), 587–594 CrossRef CAS.
- R. Kumar and C. E. Wyman, Enzyme Microb. Technol., 2008, 42(5), 426–433 CrossRef CAS.
- R. Kumar and C. E. Wyman, Biotechnol. Bioeng., 2009b, 102(2), 457–467 Search PubMed.
- Q. Qing, B. Yang and C. E. Wyman, Bioresour. Technol., 2010, 101(24), 9624–9630 CrossRef CAS PubMed.
- M. Holtzapple, M. Cognata, Y. Shu and C. Hendrickson, Biotechnol. Bioeng., 1990, 36(3), 275–287 CrossRef CAS PubMed.
- P. Andric, A. S. Meyer, P. A. Jensen and K. Dam-Johansen, Biotechnol. Adv., 2010, 28(3), 308–324 CrossRef CAS PubMed.
- J. D. Stephen, W. E. Mabee and J. N. Saddler, Biofuels, Bioprod. Biorefin., 2012, 6(2), 159–176 CrossRef CAS.
- Y. H. P. Zhang, M. E. Himmel and J. R. Mielenz, Biotechnol. Adv., 2006, 24(5), 452–481 CrossRef CAS PubMed.
- Z. Zhang, A. A. Donaldson and X. Ma, Biotechnol. Adv., 2012, 30(4), 913–919 CrossRef CAS PubMed.
- R. Kumar and C. E. Wyman, Biotechnol. Bioeng., 2009c, 102(6), 1544–1557 Search PubMed.
- R. Kumar and C. E. Wyman, Biotechnol. Prog., 2009d, 25(2), 302–314 Search PubMed.
- B. Yang and C. E. Wyman, Biotechnol. Bioeng., 2006, 94(4), 611–617 CrossRef CAS PubMed.
- T. Y. Nguyen, C. M. Cai, O. Osman, R. Kumar and C. E. Wyman, Green Chem., 2015a, 18(6), 1581–1589 Search PubMed.
- T. Y. Nguyen, C. M. Cai, R. Kumar and C. E. Wyman, ChemSusChem, 2015b, 8(10), 1716–1725 Search PubMed.
- H. P. Zhang, S. Y. Ding, J. R. Mielenz, J. B. Cui, R. T. Elander, M. Laser, M. E. Himmel, J. R. Mcmillan and L. R. Lynd, Biotechnol. Bioeng., 2007, 97(2), 214–223 CrossRef PubMed.
- S. T. Merino and J. Cherry, Biofuels, 2007, 108, 95–120 CAS.
- T. L. Ogeda and D. F. S. Petri, Quim. Nova, 2010, 33(7), 1549–1558 CrossRef CAS.
- A. Y. Pribowo, J. Hu, V. Arantes and J. N. Saddler, Biotechnol. Biofuels, 2013, 6(1), 1–15 CrossRef PubMed.
- S. D. Risio, C. S. Hu, B. A. Saville, D. Liao and J. Lortie, Biofuels, Bioprod. Biorefin., 2011, 5(6), 609–620 CrossRef.
- J. D. DeMartini, S. Pattathil, U. Avci, K. Szekalski, K. Mazumder, M. G. Hahn and C. E. Wyman, Energy Environ. Sci., 2011, 4(10), 4332–4339 CAS.
- J. P. Knox, Cellulases, 2012, 510, 233–245 CAS.
- J. P. Knox, Curr. Opin. Plant Biol., 2008, 11(3), 308–313 CrossRef CAS PubMed.
- C. Oliveira, V. Carvalho, L. Domingues and F. M. Gama, Biotechnol. Adv., 2015, 33(3), 358–369 CrossRef CAS PubMed.
- A. B. Boraston, D. Bolam, H. Gilbert and G. J. Davies, Biochem. J., 2004, 382, 769–781 CrossRef CAS PubMed.
- S. Gao, C. You, S. Renneckar, J. Bao and Y. H. P. Zhang, Biotechnol. Biofuels, 2014, 7(1), 1–11 CrossRef PubMed.
- H. J. Gilbert, J. P. Knox and A. B. Boraston, Curr. Opin. Struct. Biol., 2013, 23(5), 669–677 CrossRef CAS PubMed.
-
C. Hervé, S. E. Marcus and J. P. Knox, The Plant Cell Wall, 2011, vol. 715, pp. 103–113 Search PubMed.
- S. Ding, Q. Xu, M. K. Ali, J. O. Baker, E. A. Bayer, Y. Barak, R. Lamed, J. Sugiyama, G. Rumbles and M. E. Himmel, BioTechniques, 2006, 41(4), 435 CrossRef CAS PubMed.
- T. Kawakubo, S. Karita, Y. Araki, S. Watanabe, M. Oyadomari, R. Takada, F. Tanaka, K. Abe, T. Watanabe, Y. Honda and T. Watanabe, Biotechnol. Bioeng., 2010, 105(3), 499–508 CrossRef CAS PubMed.
- K. Gourlay, V. Arantes and J. N. Saddler, Biotechnol. Biofuels, 2012, 5(1), 51 CrossRef CAS PubMed.
- J. Hong, X. Ye and Y. H. P. Zhang, Langmuir, 2007, 23(25), 12535–12540 CrossRef CAS PubMed.
- J. M. Fox, P. Jess, R. B. Jambusaria, G. M. Moo, J. Liphardt, D. S. Clark and H. W. Blanch, Nat. Chem. Biol., 2013, 9(6), 356–361 CrossRef CAS PubMed.
- V. Khatri, Y. Hebert-Ouellet, F. Meddeb-Mouelhi and M. Beauregard, Biotechnol. Biofuels, 2016, 9, 74 CrossRef PubMed.
- Y. Hébert-Ouellet, F. Meddeb-Mouelhi, V. Khatri, L. Cui, B. Janse, K. MacDonald and M. Beauregard, Green Chem., 2017, 19, 2603–2611 RSC.
- L. Szabó, S. Jamal, H. Xie, S. J. Charnock, D. N. Bolam, H. J. Gilbert and G. J. Davies, J. Biol. Chem., 2001, 276(52), 49061–49065 CrossRef PubMed.
- A. B. Boraston, T. J. Revett, C. M. Boraston, D. Nurizzo and G. J. Davies, Structure, 2003, 11(6), 665–675 CrossRef CAS PubMed.
- M. M. Bradford, Anal. Biochem., 1976, 72(1), 248–254 CrossRef CAS PubMed.
-
A. Sluiter, D. Crocker, B. Hames, R. Ruiz, C. Scarlata, J. Sluiter and D. Templeton, Laboratory Analytical Procedure (LAP), 2008, NREL/TP-510–42618 Search PubMed.
- K. Gourlay, J. Hu, V. Arantes, M. Penttilä and J. N. Saddler, J. Biol. Chem., 2015, 290, 2938–2945 CrossRef CAS PubMed.
- D. W. Abbott and A. B. Boraston, Methods Enzymol., 2012, 510, 211–231 CAS.
-
G. A. Smook, Handbook for pulp & paper technologists, Tappi, 1992 Search PubMed.
- L. Viikari, A. Kantelinen, J. Sundquist and M. Linko, FEMS Microbiol. Rev., 1994, 13(2–3), 335–350 CrossRef CAS.
- D. Tavast, Z. A. Mansoor and E. Brännvall, Ind. Eng. Chem. Res., 2014, 53(23), 9738–9742 CrossRef CAS.
- Å. Linder, R. Bergman, A. Bodin and P. Gatenholm, Langmuir, 2003, 19(12), 5072–5077 CrossRef.
- M. Åkerholm, B. Hinterstoisser and L. Salmén, Carbohydr. Res., 2004, 339(3), 569–578 CrossRef PubMed.
- A. L. Kansoh and Z. A. Nagieb, Antonie van Leeuwenhoek, 2004, 85(2), 103–114 CrossRef CAS PubMed.
- J. H. Clarke, K. Davidson, J. E. Rixon, J. R. Halstead, M. P. Fransen, H. J. Gilbert and G. P. Hazlewood, Appl. Microbiol. Biotechnol., 2000, 53(6), 661–667 CrossRef CAS PubMed.
- G. L. Miller, Anal. Chem., 1959, 31, 426–428 CrossRef CAS.
Footnote |
† Electronic supplementary information (ESI) available. See DOI: 10.1039/c7se00427c |
|
This journal is © The Royal Society of Chemistry 2018 |