DOI:
10.1039/C7QO00755H
(Research Article)
Org. Chem. Front., 2018,
5, 98-105
A selenophene substituted diketopyrrolopyrrole nanotheranostic agent for highly efficient photoacoustic/infrared-thermal imaging-guided phototherapy†
Received
25th August 2017
, Accepted 26th September 2017
First published on 27th September 2017
Abstract
Phototherapy, a light-induced cancer therapy, has presented great promise for multimodal cancer treatment. Herein, a selenophene substituted diketopyrrolopyrrole based nanotheranostic photosensitizer (SeDPP-TPA) has been designed and synthesized. The introduction of selenophene enables SeDPP-TPA to produce a high yield of singlet oxygen (1O2) (ΦΔ = 40.2%), and show a bathochromic shift in absorbance (λmax at 648 nm), enhanced charge transfer and biocompatibility. After nanoprecipitation, SeDPP-TPA nanoparticles (NPs) are prepared with excellent water dispersibility and passive tumor-targeting properties based on the enhanced permeability and retention effect. Besides, SeDPP-TPA NPs present a high photothermal conversion efficiency (η = 37.9%). An in vitro cytotoxicity test shows that the half-maximal inhibitory concentration (IC50) of SeDPP-TPA NPs on tumor cells is ∼12 μg mL−1, indicating their excellent phototherapy efficiency. What is more, because of the thermal expansion and tumor-targeting properties, SeDPP-TPA NPs can also act as a contrast agent for photoacoustic imaging to visualize the therapy in vivo. Coupled with the infrared thermal imaging properties of the photothermal agent, SeDPP-TPA NPs are proved to be a multifunctional theranostic agent for dual-modal imaging-guided phototherapy.
Introduction
Phototherapy, including photodynamic therapy (PDT) and photothermal therapy (PTT), has drawn tremendous attention because of its high therapeutic efficacy and controllable tumor targeting properties, while causing no damage to normal organs and tissues.1 In PDT, photosensitizers (PSs) are the therapeutic agents used to generate reactive oxygen species (ROS), especially singlet oxygen (1O2) with illumination, and further to selectively kill tumor cells.2 In contrast, in PTT, light is converted into heat energy through photothermal agents at the tumor sites, which induces cellular hyperthermia based cancer cell apoptosis or necrosis.3 In comparison with PDT or PTT alone, synergistic therapy has demonstrated an enhanced PD/PT therapeutic efficacy.4 However, the current combination of PDT and PTT mainly focuses on the combination of PSs (e.g. chlorin e6, indocyanine green) and photothermal agents (e.g. Au nanorods, polydopamine nanoparticles) through physical adsorption or chemical reactions, which usually involves complicated synthetic routes, multiple illumination, and more systemic toxicity.5 Therefore, it is of great importance to synthesize a photothermogenic photosensitizer, which will simultaneously generate ROS and heat under illumination.
It is well known that the introduction of heavy atoms (e.g. Ru, Ir, iodine and bromine) into PSs can efficiently improve the ROS production via enhancing intersystem crossing (ISC).6 Moreover, recent studies demonstrated that the incorporation of heavy atoms into PSs can also reduce their energy gap between the highest occupied molecular orbital (HOMO) and the lowest unoccupied molecular orbital (LUMO) with the donor–acceptor–donor (D–A–D) structure,7 which is much beneficial for the bathochromic shift in absorbance as well as the intramolecular charge transfer of organic dyes. Organic selenium (Se) compounds usually have a large dipole moment, which means more polarizable property compared to the structural analogue organosulfur compounds.8 Therefore, the bandgap can be reduced, and the optical properties can be fine tuned by introducing Se into PSs. Besides, Se compounds also exhibit weak Se–Se interactions, which is important for the formation of uniformly packed structures by cofacial interactions and improving the charge-transport properties in the aggregated state.9 This phenomenon has been proven to be much beneficial for photothermal conversion. Besides, Se is a significant trace element in biological systems. Proteins incorporating Se have a wide range of applications from anti-inflammatory and anti-oxidant effects to the production of active thyroid hormones. The nutritional functions of Se are achieved by the action of 25 selenoproteins, which have selenocysteine as the active center.10 Thus, the introduction of Se into PSs may simultaneously improve the bathochromic shift in absorbance, enhance the photothermal conversion, and improve the biological activity.
Diketopyrrolopyrrole (DPP) is a kind of plane dye with bright red color, high molar extinction coefficient, and exceptional thermal- and photo-stability. More importantly, it is easy to be modified with functional groups.11 Indeed, DPP derivatives have already been developed as water-soluble PSs by the introduction of thiophene, bromine, and so on.12 Recently, a thiophene substituted DPP based organic dye (DPP-TPA) as a multifunctional agent has been designed and synthesized by our group,13 which presents excellent photothermal conversion efficiency (η = 34.5%) and high singlet oxygen (1O2) quantum yield (ΦΔ = 33.6%), and the half-maximal inhibitory concentration (IC50) of tumor cells is about 12.5 μg mL−1. To further increase the efficiency of tumor treatment and decrease the systemic toxicity, the substitution of thiophene with other functional groups is very important.
Herein, a selenophene-containing DPP based photosensitizer, 3,6-bis(5-(4-(diphenylamino) phenyl)selenophen-2-yl)-2,5-bis(2-ethylhexyl)pyrrolo[3,4-c]pyrrole-1,4(2H,5H)-dione (SeDPP-TPA), has been designed and synthesized. The introduction of selenophene enables the photosensitizer to produce a high yield of 1O2 (ΦΔ = 40.2%) and to show a bathochromic shift in absorbance (maximum peak at 648 nm). In addition, triphenylamine (TPA) is conjugated to the electron-deficient DPP core as the electron donor to construct a low band-gap D–A–D structure.14 By nanoprecipitation,15 hydrophilic SeDPP-TPA nanoparticles (NPs) are formed with passive tumor targeting properties based on the enhanced permeability and retention (EPR) effect.16 Moreover, due to the enhanced D–A–D structure, SeDPP-TPA NPs present a higher photothermal conversion efficiency (η = 37.9%), which results in a lower IC50in vitro (∼12 μg mL−1). Associated with photothermal conversion, photoacoustic imaging (PAI) involved the generation of ultrasonic waves by thermal expansion17 of SeDPP-TPA NPs which can also make the in vivo therapy visualized. Coupled with the natural infrared thermal imaging property,18 SeDPP-TPA NPs can serve as a multifunctional theranostic agent for dual-modal imaging guided photodynamic and photothermal therapy (Scheme 1).
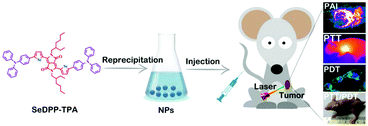 |
| Scheme 1 Schematic illustration of the formation and application of SeDPP-TPA NPs in dual-modal imaging guided photodynamic and photothermal therapy. | |
Experimental section
Materials and apparatus
Selenophene, palladium acetate, chlorosulfonyl isocyanate, and pivalic acid are purchased from Sigma-Aldrich. N-Bromosuccinimide, 3-(bromomethyl) heptane, anhydrous dimethylacetamide, and 4-bromo-N,N-diphenylaniline are purchased from Adamas. 1H NMR and 13C NMR spectra are recorded in CDCl3 or DMSO-D6 on a Bruker DRX NMR spectrometer (500 MHz) with tetramethylsilane as an internal standard. The absorption spectra are recorded on a UV-vis spectrophotometer (Shimadzu, Japan, UV-3600). The morphology of nanoparticles is observed on a scanning electron microscope (SEM, Japan, Hitachi S-4800). The size of the nanoparticles is measured by using a particle size analyzer (Brookhaven Instruments, USA, 90 Plus). Photoacoustic images are obtained with a Nexus128 small animal photoacoustic imaging system (Ann Arbor, MI, USA). Infrared thermal imaging is performed by using an infrared camera (FLIR, Arlington, VA, E50). All animal experiments are performed in compliance with the relevant laws and institutional guidelines, and the institutional ethics committee has approved the experiments.
Synthesis of selenophene-2-carbonitrile (1)
Chlorosulfonyl isocyanate (5.69 g, 40.2 mmol) is added dropwise to an ice cooled dry dichloromethane (25 mL) solution of selenophene (5 g, 38.2 mmol). The reaction mixture is stirred at 10 °C for 1 hour and then for another 1 hour at room temperature. After that, the mixture was cooled to 0 °C, and then dimethylformamide (5.86 g, 80.05 mmol) is added dropwise over 30 min. After stirring for two hours at room temperature, the resulting mixture is poured into ice and extracted with dichloromethane. The organic layer is washed with brine, and dried over sodium sulfate. The solvent was removed under rotary evaporation and purified by distillation (Kugelrohr, 105 °C, 4 mbar) to obtain a yellow liquid (3.74 g, 62.4%). 1H NMR (300 MHz, CDCl3) δ 8.38 (d, J = 5.0 Hz, 1H), 7.88 (d, J = 2.7 Hz, 1H), 7.42–7.33 (m, 1H). 13C NMR (101 MHz, CDCl3) δ 142.28, 141.62, 129.89, 116.45, 112.63. MALDI-TOF MS (m/z): [M]+ calcd for C5H3NSe, 156.94; found, 156.9629.
Synthesis of 3,6-bis-(selenophenyl)-1,4-diketopyrrolo[3,4-c]pyrrole (2)
Sodium (1.05 g, 43.5 mmol) and iron(III) chloride (5 mg) are added to a stirred 2-methylbutan-2-ol solution (25 mL) and heated to reflux to clear. After that, the solution is cooled to 90 °C and selenophene-2-carbonitrile (4.45 g, 29 mmol) is added with diisopropyl succinate (2.64 g, 13 mmol). The mixture is stirred at 90 °C for 4 hours and then cooled to 50 °C. The reaction is quenched by using acetic acid (5 mL) and methanol (30 mL). Then, the mixture is stirred at 50 °C for 15 minutes and poured into methanol (50 mL). The precipitate is collected by filtration to obtain purple powder (2.12 g, 37%). 1H NMR (500 MHz, DMSO) δ 11.22 (s, 2H), 8.64 (d, J = 6.7 Hz, 2H), 8.36 (d, J = 5.2 Hz, 2H), 7.52 (dd, J = 5.8, 4.1 Hz, 2H). 13C NMR (101 MHz, DMSO) δ 161.79, 138.77, 137.44, 135.11, 133.65, 130.07, 108.63. MALDI-TOF MS (m/z): [M]+ calcd for C14H8N2O2Se2, 395.89; found, 394.9986.
Synthesis of SeDPP
Under an N2 atmosphere, 3,6-bis-(selenophenyl)-1,4-diketopyrrolo[3,4-c]pyrrole (0.79 g, 2.0 mmol), 3-(bromomethyl)heptane (0.988 g, 4.8 mmol), and anhydrous potassium carbonate (0.332 g, 2.4 mmol) are added to dimethylformamide (15 mL). The mixture is stirred overnight at 120 °C, and then cooled to room temperature and poured into 300 mL water and extracted with dichloromethane. The organic layer is further washed with brine, and dried with anhydrous sodium sulfate. The organic solvent is removed by rotary evaporation and purified by column chromatography (silica gel, PE/DCM = 2
:
1, v/v) (0.484 g, yield: 39%). 1H NMR (500 MHz, CDCl3) δ 8.67 (s, 2H), 8.04 (s, 2H), 7.51 (s, 2H), 4.05 (m, 4H), 1.27 (m, 18H), 0.86 (m, 12H). 13C NMR (126 MHz, CDCl3) δ 161.51, 141.73, 137.22, 136.51, 133.79, 130.99, 107.84, 77.24, 76.98, 76.73, 41.90, 33.57, 32.57, 29.81, 27.76, 26.05. MALDI-TOF MS (m/z): [M]+ calcd for C30H40N2O2Se2, 620.14; found, 620.4415.
Synthesis of SeDPP-TPA
Under an N2 atmosphere, SeDPP (0.62 g, 1 mmol), Pd(OAc)2 (0.006 g, 0.025 mmol), 4-bromo-N,N-diphenylaniline (0.808 g, 2.5 mmol), pivalic acid (0.015 g, 0.15 mmol) and anhydrous potassium carbonate (0.345 g, 2.5 mmol) are stirred in anhydrous dimethylacetamide (5 mL) at 110 °C for 2 hours. After cooling to room temperature, the mixture is then poured into 100 mL water and extracted with dichloromethane. The organic layer is washed with brine, dried with anhydrous magnesium sulfate and removed by rotary evaporation. The crude product is further purified by chromatography (silica gel, DCM/PE = 8
:
1, v/v) (0.841 g, yield: 76%). 1H NMR (400 MHz, CDCl3) δ 8.90 (d, J = 4.2 Hz, 2H), 7.52 (m, 2H), 7.32 (s, 4H), 7.14 (d, J = 7.8 Hz, 8H), 7.09–7.02 (m, 16H), 4.05 (s, 4H), 1.77 (s, 2H), 1.31 (m, 16H), 0.88 (s, 12H). 13C NMR (101 MHz, CDCl3) δ 161.91, 144.63, 137.05, 136.42, 134.45, 129.47, 128.23, 126.24 125.05, 124.23, 122.58, 119.52, 107.84, 47.23, 38.16, 32.03, 30.96, 30.26, 29.84, 29.71, 25.53, 22.75, 14.10. MALDI-TOF MS (m/z): [M]+ calcd for C66H66N2O2Se2, 1106.35; found, 1106.7521. Elemental analysis calcd for C66H66N2O2Se2: C, 71.73; H, 6.02; N, 5.07; O, 2.90; Se, 14.29%; found: C, 71.45; H, 6.23; N, 5.13%.
Preparation of SeDPP-TPA NPs
A SeDPP-TPA (200 μL, 2 mg mL−1) tetrahydrofuran solution is added dropwise into water (5 mL) with vigorous stirring at room temperature. Then, the mixture is stirred for another 5 minutes and tetrahydrofuran is removed by nitrogen-blowing. SeDPP-TPA NPs are obtained by centrifugation.
Singlet oxygen detection
1,3-Diphenylisobenzofuran (DPBF) is used as a probe to detect singlet oxygen. SeDPP-TPA (10−5 mol L−1) mixed with DPBF (10−5 mol L−1) of dichloromethane solution is prepared in a dark room. Then, the absorption spectra of the solution are immediately recorded after each laser irradiation (660 nm, 1.0 W cm−2). Methylene blue is used as the reference to calculate the singlet oxygen quantum yield of SeDPP-TPA.
In vitro photoacoustic imaging
SeDPP-TPA NPs (0, 5, 10, 20, and 40 μg mL−1, respectively) in PBS solution are introduced into Eppendorf tubes, which are put in deionized water for PAI. The images are obtained by using a Nexus128 small animal PAI system with excitation at 680 nm wavelength.
In vitro photothermal effect
SeDPP-TPA NPs (0, 20, and 40 μg mL−1, respectively) in PBS solution are put into Eppendorf tubes and then irradiated with a 660 nm laser (0.5, 0.75, 1.0 W cm−2, respectively) for 10 min. The temperature is recorded by using an E50 infrared camera.
Cell lines
HeLa cell line is provided by the Institute of Biochemistry and Cell Biology, SIBS, CAS (China). HeLa cells are cultured in Dulbecco's modified Eagle's medium (DMEM, Gibco) containing 10% fetal bovine serum (FBS) at 37 °C under 5% CO2.
Cellular uptake study
HeLa cells are incubated with a PBS solution of SeDPP-TPA NPs (20 μg mL−1, 100 μL) in a confocal dish at 37 °C for 24 hours in a 5% CO2 incubator. The medium is then removed and further rinsed with PBS. The fluorescence images are observed with an Olympus IX 70 inverted microscope with excitation using a 630 nm laser and collected from 650 to 700 nm. The cells are stained with the nuclei-specific dye DAPI.
Fluorescence images of cellular ROS
HeLa cells are incubated with the PBS solution of SeDPP-TPA NPs (20 μg mL−1, 100 μL) in a confocal dish at 37 °C for 24 hours in a 5% CO2 incubator. Then, the cells are incubated with 2,7-dichlorodihydrofluorescein diacetate (10 μM, 200 μL) for 1 h and irradiated with a laser (660 nm, 1 W cm−2) for 5 min. The fluorescence images are observed by using an Olympus IX 70 inverted microscope excited at 488 nm and collected from 500 to 550 nm. The cells are also stained with DAPI.
Cytotoxicity assay
The PBS solution of SeDPP-TPA NPs is diluted to different concentrations with DMEM. HeLa cells are divided into 2 groups in 96-well cell-culture plates. SeDPP-TPA NP solutions are added in the plates (200 μL) in control wells. One group of cells is maintained in the dark and the other group is irradiated with a laser for 5 minutes (660 nm, 1 W cm−2) after 24 hours. Both cells are incubated for another 48 hours and treated with 3-(4,5-dimethylthiazol-2-yl)-2,5-diphenyltetrazolium bromide (MTT) solution (500 μg mL−1) for 4 hours at 37 °C in 5% CO2, and then 150 μL of DMSO is added. The absorption at 570 nm is recorded by using a Bio-Tek microplate reader.
Animal models
HeLa cells are injected into the subcutaneous sites of nude mice at the age of 6 weeks. These mice are used for PAI, infrared thermal imaging and phototherapy when the volumes of tumors were about 100 mm3.
In vivo photoacoustic and infrared thermal imaging
100 μL of SeDPP-TPA NP (40 μg mL−1) PBS solution is injected into the HeLa tumor-bearing mice through a tail vein. Then, PAI of the tumor sites in different periods is conducted on a Nexus128 small animal PAI system with the excitation at 680 nm. Infrared thermal imaging of the tumor sites is conducted after 4 hours of the injection of SeDPP-TPA NPs into the mice, and then the tumors are irradiated with a 660 nm laser (1 W cm−2) for different times. The images are monitored by using an E50 infrared camera.
In vivo phototherapy
18 HeLa tumor-bearing mice are divided into 3 groups randomly (group 1: PBS with laser treatment, group 2: SeDPP-TPA NPs only, and group 3: SeDPP-TPA NPs with a laser). For each group, the mice are injected 100 μL PBS solution of SeDPP-TPA NPs (40 μg mL−1) or PBS through a tail vein, respectively. 2–4 hours later, the mice in group 1 and group 3 are irradiated for 8 min with a laser (660 nm, 1.0 W cm−2). The treatments are repeated every other day. Tumor volumes and body weight changes of mice are then measured. Tumor volumes are calculated using the following equation: V = width2 × length/2.
Ex vivo histology examination
These mice are sacrificed after the treatments, and the heart, liver, spleen, lung and kidney are separated from the bodies for further histological analysis. After dehydration, these tissues are stained with hematoxylin and eosin (H&E) and embedded in paraffin cassettes. The images of H&E stained tissues are observed by using an optical microscope.
Results and discussion
Synthesis and characterization
The detailed synthetic routes for the molecule SeDPP-TPA are shown in Scheme S1† with good yield according to the reported literature.19 Selenophene is introduced onto the DPP core for a bathochromic shift in absorbance and more ROS generation, and TPA is located at the periphery to extend the conjugation. Theoretical calculations of DPP-TPA and SeDPP-TPA were performed by using density functional theory (DFT) with the B3LYP/6-311G (d, p) basis set. Two model compounds with all alkyl side chains replaced by methyl groups were used to simplify the calculation. The calculated HOMO energy levels for DPP-TPA and SeDPP-TPA are −4.56 eV and −4.54 eV, and the LUMO energy levels are −2.39 eV and −2.44 eV, respectively. According to the calculated HOMO and LUMO energy levels, the calculated bandgap for each compound is 2.17 eV (DPP-TPA) and 2.10 eV (SeDPP-TPA). As illustrated in Fig. 1, for the LUMO orbitals, the π electron density mainly locates around the DPP unit. While for the HOMO orbitals, the π electron density distributes along the long molecular axes, indicating that the HOMO energy level is determined by the jointed donor and acceptor units in the molecules. And this is in good agreement with the UV-vis-NIR absorption measurement results (λmax = 630 nm for DPP-TPA, λmax = 648 nm for SeDPP-TPA). Theoretical calculations also show that after the selenophene unit substitution, ΔEST was reduced from 1.03 eV to 1.00 eV, indicating that SeDPP-TPA facilitates ISC and ROS generation. Furthermore, the 1O2 quantum yield of SeDPP-TPA is determined to be 40.2%, which is higher than that of DPP-TPA (33.6%).
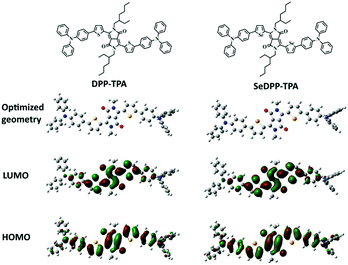 |
| Fig. 1 Minimized energy ground state geometry and the frontier molecular orbitals electron density distribution of DPP-TPA and SeDPP-TPA. | |
By nanoprecipitation, hydrophobic SeDPP-TPA is further prepared into water-dispersible NPs with indigo color (Fig. 2a). Fig. S1† shows the UV-vis absorption spectrum of SeDPP-TPA in dichloromethane, and the two peaks at 648 nm and 610 nm indicate that the conjugated structure has a good absorption in the near-infrared (NIR) interrogation window. Besides, the fluorescence quantum yield of SeDPP-TPA is calculated to be 0.23 by using rhodamine B (0.7 in ethanol) as a reference (Fig. S2†), and the low fluorescence quantum yield indicates that more non-radiative transitions would occur and may result in high photothermal conversion efficiency. After the formation of NPs, the absorption spectrum presents a bathochromic shift with peaks at 672 nm and 612 nm (Fig. 2b), which is caused by intermolecular interactions in the NPs.20 The SEM image shows that SeDPP-TPA NPs are uniform spheres with a size less than 100 nm (Fig. 2c), which is useful for the EPR effect in tumor treatment. Besides, SeDPP-TPA NPs showed no obvious changes under different biological conditions after standing for 48 h (Fig. S3†), indicating the good stability of SeDPP-TPA NPs. These results preliminarily indicate that SeDPP-TPA NPs have the potential application for NIR light-induced imaging and therapy (Table 1).
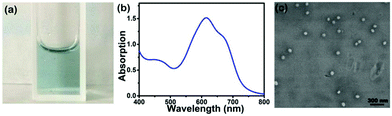 |
| Fig. 2 (a) Photograph of SeDPP-TPA NPs in PBS (pH 7.4, 40 μg mL−1). (b) UV-vis absorption spectrum of SeDPP-TPA NPs in PBS (pH 7.4). (c) SEM image of SeDPP-TPA NPs. | |
Table 1 Optical absorptions and theoretical calculated results of DPP-TPA and SeDPP-TPA
Compound |
λ
max (nm) |
HOMO (eV) |
LUMO (eV) |
ΔEcalg (eV) |
E
s1 (eV) |
E
T1 (eV) |
ΔEST (eV) |
DPP-TPA |
630 |
−4.56 |
−2.39 |
2.17 |
1.99 |
0.96 |
1.03 |
SeDPP-TPA |
648 |
−4.54 |
−2.44 |
2.10 |
1.93 |
0.93 |
1.00 |
The in vitro1O2 generation, photoacoustic (PA) properties and photothermal conversion efficiency of SeDPP-TPA NPs are subsequently examined, respectively. Firstly, the 1O2 generation ability is measured by using 1,3-diphenylisobenzofuran (DPBF) as a probe, the absorption of which at 418 nm decreases upon reaction with 1O2.21 As shown in Fig. 3a, the absorbance at 418 nm rapidly decreases and completely disappears within 8 seconds under laser irradiation (660 nm, 1.0 W cm−2), and the singlet oxygen quantum yield is calculated to be ∼40%, indicating that SeDPP-TPA has excellent 1O2 generation ability. Besides, there is almost no degradation of SeDPP-TPA itself under the irradiation, indicating the good photostability of SeDPP-TPA. Fig. 3b shows the PA properties of SeDPP-TPA NPs, and the PA intensity increases obviously with the increase of SeDPP-TPA NP concentration, exhibiting excellent concentration dependence. When the concentration is 40 μg mL−1, the PA image presents the strongest signal. Moreover, the PA intensity and concentration of the NPs present a linear relationship with R2 equal to 0.9931. A 660 nm laser is also used as the light source to measure the photothermal conversion efficiency of SeDPP-TPA NPs in PBS. As shown in Fig. 3c, with the increase of NP concentration, the temperature rises gradually under laser irradiation (1.0 W cm−2), indicating the significant concentration effect on photothermal conversion. When the concentration of SeDPP-TPA NPs is 40 μg mL−1, the temperature quickly elevates by about 16 °C within 60 seconds and the temperature elevation (ΔT) can finally reach up to around 40 °C within 10 minutes, demonstrating the excellent photothermal conversion effect of SeDPP-TPA NPs in the dispersion system. Fig. 3d shows that the temperature rises obviously as the laser power density increases, which presents obvious laser power intensity dependence. The photothermal conversion efficiency of SeDPP-TPA NPs is calculated to be 37.9%, which is also higher than the previously reported photothermal agents.22 These results above present the multifunctions of SeDPP-TPA NPs as a promising theranostic agent for PAI, PTT and PDT for cancer treatment.
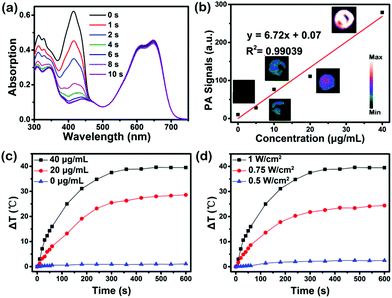 |
| Fig. 3 (a) Absorbance decrease of DPBF (c = 10−5 mol L−1) mixed with SeDPP-TPA (c = 10−5 mol L−1) in DCM under laser irradiation over time (660 nm, 1.0 W cm−2). (b) The relationship between the PA intensity and SeDPP-TPA NP concentration, and PA images in PBS (pH 7.4). (c) Photothermal conversion of SeDPP-TPA NPs at different concentrations in PBS (pH 7.4) under laser irradiation (660 nm, 1.0 W cm−2). (d) Photothermal conversion of SeDPP-TPA NPs in PBS (pH 7.4) with different power densities. | |
Cellular investigation
HeLa cell arrays are employed to investigate the capability of cellular uptake and the phototherapy effect of SeDPP-TPA NPs in vitro. Fig. 4a shows the fluorescence imaging of SeDPP-TPA NPs (20 μg mL−1, 100 μL) in cells after incubation for 24 hours. The bright red fluorescence represents SeDPP-TPA NPs, which indicates the abundant uptake of the NPs by the HeLa cells. Then, 2′,7′-dichlorofluorescein diacetate (DCFH-DA, without being fluorescent itself, but can generate fluorescent 2′,7′-dichlorofluorescein (DCF) upon reacting with ROS)23 is used to detect the intracellular ROS generation of SeDPP-TPA NPs in the cells. As shown in Fig. 4b, after incubating the HeLa cells with SeDPP-TPA NPs and DCFH-DA, under laser irradiation (660 nm, 1.0 W cm−2) for 5 minutes, strong green fluorescence is observed (excited at 488 nm), which comes from the production of DCF in the cells. This phenomenon further confirms the excellent ROS generation capability of SeDPP-TPA NPs in vitro. The 3-(4,5-dimethylthiazol-2-yl)-2,5-diphenyl-tetrazolium bromide (MTT) assay was executed to investigate the therapy effect of SeDPP-TPA NPs in vitro.24 As shown in Fig. 4c, with the laser irradiation (660 nm, 1.0 W cm−2), the cell viability obviously decreases as the concentration increases, and the half-maximal inhibitory concentration (IC50, 12 μg mL−1) is lower than DPP-TPA under the same conditions (12.5 μg mL−1), which implies the better phototherapy efficacy of SeDPP-TPA NPs in tumor cells. However, the cells incubated with the SeDPP-TPA NPs but without laser irradiation present nearly 100% viability, indicating the excellent biocompatibility of SeDPP-TPA NPs. These in vitro results confirm that SeDPP-TPA NPs present desirable biocompatibility in biological tissue and efficient phototoxicity in tumor cells.
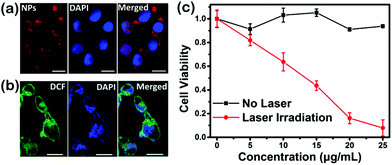 |
| Fig. 4 (a) Fluorescence images of HeLa cells incubated with SeDPP-TPA NPs (excited at 635 nm). (b) Fluorescence images of HeLa cells incubated with SeDPP-TPA NPs and DCFH-DA after laser irradiation (excited at 488 nm). (c) Cell viabilities at different concentrations of SeDPP-TPA NPs with or without laser irradiation (660 nm, 1.0 W cm−2). Scale bar = 20 μm. | |
In vivo imaging
Fig. 5a shows the PA images of the tumor sites at different times after the injection of SeDPP-TPA NPs. Clearly, the PA intensity increases rapidly after injection and maintains a rather high level from 1 to 6 hours, suggesting excellent PAI ability as well as passive tumor targeting of the NPs by the EPR effect in vivo. Fig. 5b shows the mean PA intensity against time; it shows that the PA intensity increases rapidly from 1 to 2 hours after injection and it remains at the maximum level between 2 and 4 hours. While after 8 hours of injection, the PA signal lowers to the original state, suggesting that SeDPP-TPA NPs have been metabolized. Fig. 5c shows two three-dimensional (3D) PA images at 0 and 4 hours after the injection of SeDPP-TPA NPs, respectively. The huge contrast can be visualized, which also demonstrates that SeDPP-TPA NPs are distributed throughout the tumor sites. According to the results of PAI, infrared thermal imaging is further investigated after 4 hours of the injection of SeDPP-TPA NPs into the mice with laser irradiation (660 nm, 1.0 W cm−2). As shown in Fig. 5d, the temperature of the tumor sites after SeDPP-TPA NP injection increases rapidly and reaches a plateau of 61 °C after 6 minutes, which can effectively ablate the tumor. However, the temperature of the PBS injection group shows no obvious change. These results confirm the excellent PAI and infrared thermal imaging properties, showing the tumor diagnosing ability of SeDPP-TPA NPs.
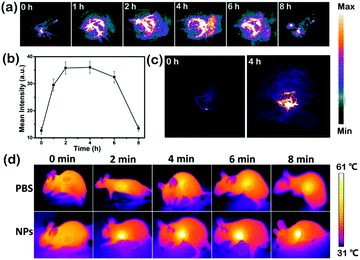 |
| Fig. 5 (a) PA images of the tumor sites at different times after the injection of SeDPP-TPA NPs (0.2 mg kg−1) in mice. (b) The relationship between the PA intensity of the tumor sites and time. (c) 3D PA images of the tumor sites at 0 and 4 hours. (d) Infrared thermal images of the tumor sites (white circle) under laser irradiation (660 nm, 1.0 W cm−2) at different times after injecting PBS or SeDPP-TPA NPs. | |
In vivo phototherapy
Motivated by the great ROS generation, dual-modal imaging and photothermal conversion performance of SeDPP-TPA NPs, in vivo phototherapy is further investigated. 18 HeLa tumor bearing mice were randomly divided into 3 groups for different treatments. As shown in Fig. 6a, the tumor volumes of the NP only group and the laser irradiation only group increase rapidly in the treatment period. In contrast, the tumors are eliminated with only one treatment in the NP injection and laser irradiation group (660 nm, 1.0 W cm−2), which demonstrates the excellent phototherapy effect of SeDPP-TPA NPs. Besides, there is no tumor recurrence during the whole observed period, indicating that SeDPP-TPA NPs can effectively inhibit the tumor growth. The body weight changes of mice are shown in Fig. 6b. The mice body weight presents a normal growth of all the three groups, which means that SeDPP-TPA NPs are biocompatible and have low toxicity in vivo. Fig. 6c shows the representative photographs of the mice from 3 groups after 14 days of treatment, respectively. Obviously, the tumor disappears after phototherapy, but the tumors of the other two mice were very huge, which further reflects the excellent phototherapy effect of SeDPP-TPA NPs in vivo. After 14 days of treatment, the mice are sacrificed, and the heart, liver, spleen, lung and kidney are removed for the pathomorphology analysis. As shown in Fig. 7, from the hematoxylin and eosin (H&E)25 stained tissue slices, no noticeable tissue damage or adverse effect can be observed in these major organs of the mice from all the three groups. The result further demonstrates that SeDPP-TPA NPs are biocompatible to normal organs. Therefore, these measurements in vivo demonstrate that SeDPP-TPA NPs can serve as an excellent therapeutic agent for dual-modal imaging guided tumor phototherapy without appreciable side effects.
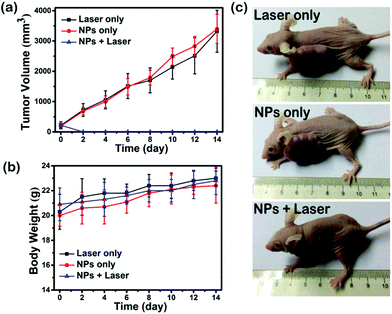 |
| Fig. 6 (a) The relationship between the tumor growth and time with different treatments. (b) The relationship between the body weight and time with different treatments. (c) Photographs of mice with different treatments after 14 days. | |
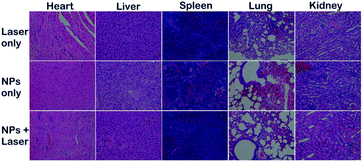 |
| Fig. 7 H&E stained images of the heart, liver, spleen, lung and kidney of mice after different treatments for 14 days. | |
Conclusions
In summary, a selenophene substituted DPP-based photosensitizer (SeDPP-TPA NPs) has been synthesized for photoacoustic and infrared thermal imaging guided photodynamic and photothermal therapy. The introduction of selenophene enables the photosensitizer to produce a high yield of 1O2 (ΦΔ = 40.2%) and to show a bathochromic shift in absorption (maximum peak at 648 nm). By nanoprecipitation, SeDPP-TPA NPs are formed with excellent water dispersibility and passive tumor targeting properties by the EPR effect. Besides, SeDPP-TPA NPs also exhibit high photothermal conversion efficiency (η = 37.9%) under laser irradiation. The in vitro half-maximal inhibitory concentration (IC50) of the treatment group is around 12 μg mL−1, indicating a more efficient phototherapy effect. And, SeDPP-TPA NPs can also act as a PAI contrast agent to make the therapy visualized in vivo. As a result, SeDPP-TPA NPs have great potential to act as a multifunctional theranostic agent for photoacoustic/infrared thermal imaging-guided phototherapy in clinic.
Conflicts of interest
There are no conflicts to declare.
Acknowledgements
The work was supported by the NNSF of China (61525402, 61775095, 61604071, 61371066), the Key University Science Research Project of Jiangsu Province (15KJA430006), and the Natural Science Foundation of Jiangsu Province (BK20161012), Jiangsu Provincial Key Research and Development plan (BE2017741).
Notes and references
-
(a) L. Y. Feng, L. Wu and X. G. Qu, Adv. Mater., 2013, 25, 168 CrossRef CAS PubMed;
(b) D. Dolmans, D. Fukumura and R. K. Jain, Nat. Rev. Cancer, 2003, 3, 380 CrossRef CAS PubMed;
(c) X. H. Huang, I. H. El-Sayed, W. Qian and M. A. El-Sayed, J. Am. Chem. Soc., 2006, 128, 2115 CrossRef CAS PubMed.
-
(a) P. Agostinis, K. Berg, K. A. Cengel, T. H. Foster, A. W. Girotti, S. O. Gollnick, S. M. Hahn, M. R. Hamblin, A. Juzeniene, D. Kessel, M. Korbelik, J. Moan, P. Mroz, D. Nowis, J. Piette, B. C. Wilson and J. Golab, Ca-Cancer J. Clin., 2011, 61, 250 CrossRef PubMed;
(b) A. P. Castano, P. Mroz and M. R. Hamblin, Nat. Rev. Cancer, 2006, 6, 535 CrossRef CAS PubMed.
-
(a) P. K. Jain, X. H. Huang, I. H. El-Sayed and M. A. El-Sayed, Acc. Chem. Res., 2008, 41, 1578 CrossRef CAS PubMed;
(b) S. Lal, S. E. Clare and N. J. Halas, Acc. Chem. Res., 2008, 41, 1842 CrossRef CAS PubMed;
(c) F. P. Gao, Y. X. Lin, L. L. Li, Y. Liu, U. Mayerhoffer, P. Spenst, J. G. Su, J. Y. Li, F. Wurthner and H. Wang, Biomaterials, 2014, 35, 1004 CrossRef CAS PubMed.
-
(a) J. Lin, S. J. Wang, P. Huang, Z. Wang, S. H. Chen, G. Niu, W. W. Li, J. He, D. X. Cui, G. M. Lu, X. Y. Chen and Z. H. Nie, ACS Nano, 2013, 7, 5320 CrossRef CAS PubMed;
(b) J. Wang, G. Z. Zhu, M. X. You, E. Q. Song, M. I. Shukoor, K. J. Zhang, M. B. Altman, Y. Chen, Z. Zhu, C. Z. Huang and W. H. Tan, ACS Nano, 2012, 6, 5070 CrossRef CAS PubMed;
(c) W. S. Kuo, Y. T. Chang, K. C. Cho, K. C. Chiu, C. H. Lien, C. S. Yeh and S. J. Chen, Biomaterials, 2012, 33, 3270 CrossRef CAS PubMed.
-
(a) J. J. Peng, L. Z. Zhao, X. J. Zhu, Y. Sun, W. Feng, Y. H. Gao, L. Y. Wang and F. Y. Li, Biomaterials, 2013, 34, 7905 CrossRef CAS PubMed;
(b) B. K. Wang, J. H. Wang, Q. Liu, H. Huang, M. Chen, K. Y. Li, C. Z. Li, X. F. Yu and P. K. Chu, Biomaterials, 2014, 35, 1954 CrossRef CAS PubMed;
(c) G. Terentyuk, E. Panfilova, V. Khanadeev, D. Chumakov, E. Genina, A. Bashkatov, V. Tuchin, A. Bucharskaya, G. Maslyakova, N. Khlebtsov and B. Khlebtsov, Nano Res., 2014, 7, 325 CrossRef CAS.
-
(a) J. Z. Zhao, W. H. Wu, J. F. Sun and S. Guo, Chem. Soc. Rev., 2013, 42, 5323 RSC;
(b) A. Gorman, J. Killoran, C. O'Shea, T. Kenna, W. M. Gallagher and D. F. O'Shea, J. Am. Chem. Soc., 2004, 126, 10619 CrossRef CAS PubMed.
-
(a) P. Johari and V. B. Shenoy, ACS Nano, 2012, 6, 5449 CrossRef CAS PubMed;
(b) G. L. Gibson, T. M. McCormick and D. S. Seferos, J. Am. Chem. Soc., 2012, 134, 539 CrossRef CAS PubMed;
(c) A. J. Kronemeijer, E. Gili, M. Shahid, J. Rivnay, A. Salleo, M. Heeney and H. Sirringhaus, Adv. Mater., 2012, 24, 1558 CrossRef CAS PubMed.
-
(a) R. Acharya, S. Cekli, C. J. Zeman, R. M. Altamimi and K. S. Schanze, J. Phys. Chem. Lett., 2016, 7, 693 CrossRef CAS PubMed;
(b) C. Bleiholder, D. B. Werz, H. Koppel and R. Gleiter, J. Am. Chem. Soc., 2006, 128, 2666 CrossRef CAS PubMed;
(c) C. M. Robertson, A. A. Leitch, K. Cvrkalj, R. W. Reed, D. J. T. Myles, P. A. Dube and R. T. Oakley, J. Am. Chem. Soc., 2008, 130, 8414 CrossRef CAS PubMed.
-
(a) C. Graf, A. Assoud, O. Mayasree and H. Kleinke, Molecules, 2009, 14, 3115 CrossRef CAS PubMed;
(b) H. F. Ren, Z. H. Huang, H. Yang, H. P. Xu and X. Zhang, ChemPhysChem, 2015, 16, 523 CrossRef CAS PubMed.
- D. Wu, L. Chen, N. Kwon and J. Yoon, Chem, 2016, 1, 674 CAS.
-
(a) M. Grzybowski and D. T. Gryko, Adv. Opt. Mater., 2015, 3, 280 CrossRef CAS;
(b) M. Kaur and D. H. Choi, Chem. Soc. Rev., 2015, 44, 58 RSC;
(c) S. Qu and H. Tian, Chem. Commun., 2012, 48, 3039 RSC.
-
(a) Y. Cai, Q. Y. Tang, X. J. Wu, W. L. Si, Q. Zhang, W. Huang and X. C. Dong, ACS Appl. Mater. Interfaces, 2016, 8, 10737 CrossRef CAS PubMed;
(b) Y. Cai, Q. Tang, X. Wu, W. Si, W. Huang, Q. Zhang and X. Dong, ChemistrySelect, 2016, 1, 3071 CrossRef CAS.
- Y. Cai, P. Liang, Q. Tang, X. Yang, W. Si, W. Huang, Q. Zhang and X. Dong, ACS Nano, 2017, 11, 1054 CrossRef CAS PubMed.
- S. H. Zeng, L. X. Yin, X. Y. Jiang, Y. Q. Li and K. C. Li, Dyes Pigm., 2012, 95, 229 CrossRef CAS.
- B. K. An, S. K. Kwon, S. D. Jung and S. Y. Park, J. Am. Chem. Soc., 2002, 124, 14410 CrossRef CAS PubMed.
- X. Song, R. Zhang, C. Liang, Q. Chen, H. Gong and Z. Liu, Biomaterials, 2015, 57, 84 CrossRef CAS PubMed.
-
(a) K. Pu, A. J. Shuhendler, J. V. Jokerst, J. Mei, S. S. Gambhir, Z. Bao and J. Rao, Nat. Nanotechnol., 2014, 9, 233 CrossRef CAS PubMed;
(b) Q. Fan, K. Cheng, Z. Yang, R. Zhang, M. Yang, X. Hu, X. Ma, L. Bu, X. Lu, X. Xiong, W. Huang, H. Zhao and Z. Cheng, Adv. Mater., 2015, 27, 843 CrossRef CAS PubMed;
(c) D. Zhang, Y.-X. Zhao, Z.-Y. Qiao, U. Mayerhöffer, P. Spenst, X.-J. Li, F. Würthner and H. Wang, Bioconjugate Chem., 2014, 25, 2021 CrossRef CAS PubMed.
-
(a) J. Mou, T. Lin, F. Huang, H. Chen and J. Shi, Biomaterials, 2016, 84, 13 CrossRef CAS PubMed;
(b) Y. Cai, W. Si, Q. Tang, P. Liang, C. Zhang, P. Chen, Q. Zhang, W. Huang and X. Dong, Nano Res., 2017, 10, 794 CrossRef CAS.
-
(a) M. Shahid, T. McCarthy-Ward, J. Labram, S. Rossbauer, E. B. Domingo, S. E. Watkins, N. Stingelin, T. D. Anthopoulos and M. Heeney, Chem. Sci., 2012, 3, 181 RSC;
(b) S.-Y. Liu, M.-M. Shi, J.-C. Huang, Z.-N. Jin, X.-L. Hu, J.-Y. Pan, H.-Y. Li, A. K. Y. Jen and H.-Z. Chen, J. Mater. Chem. A, 2013, 1, 2795 RSC.
- K. K. Ng and G. Zheng, Chem. Rev., 2015, 115, 11012 CrossRef CAS PubMed.
- H. Shi, W. Sun, C. Liu, G. Gu, B. Ma, W. Si, N. Fu, Q. Zhang, W. Huang and X. Dong, J. Mater. Chem. B, 2016, 4, 113 RSC.
-
(a) Q. W. Tian, F. R. Jiang, R. J. Zou, Q. Liu, Z. G. Chen, M. F. Zhu, S. P. Yang, J. L. Wang, J. H. Wang and J. Q. Hu, ACS Nano, 2011, 5, 9761 CrossRef CAS PubMed;
(b) Y. Cao, H. F. Dong, Z. Yang, X. M. Zhong, Y. Chen, W. H. Dai and X. J. Zhang, ACS Appl. Mater. Interfaces, 2017, 9, 159 CrossRef CAS PubMed;
(c) Y. P. Yan, Q. Yang, J. Wang, H. Y. Jin, J. Wang, H. Yang, Z. G. Zhou, Q. W. Tian and S. P. Yang, J. Mater. Chem. B, 2017, 5, 382 RSC.
- L. Xia, X. Kong, X. Liu, L. Tu, Y. Zhang, Y. Chang, K. Liu, D. Shen, H. Zhao and H. Zhang, Biomaterials, 2014, 35, 4146 CrossRef CAS PubMed.
- H. Fan, G. Yan, Z. Zhao, X. Hu, W. Zhang, H. Liu, X. Fu, T. Fu, X. B. Zhang and W. Tan, Angew. Chem., Int. Ed., 2016, 55, 5477 CrossRef CAS PubMed.
- S. Su, Y. Ding, Y. Li, Y. Wu and G. Nie, Biomaterials, 2016, 80, 169 CrossRef CAS PubMed.
Footnote |
† Electronic supplementary information (ESI) available: Supplementary figures. See DOI: 10.1039/c7qo00755h |
|
This journal is © the Partner Organisations 2018 |