DOI:
10.1039/C8QM00314A
(Review Article)
Mater. Chem. Front., 2018,
2, 2152-2174
Recent progress in macrocyclic amphiphiles and macrocyclic host-based supra-amphiphiles
Received
28th June 2018
, Accepted 19th September 2018
First published on 19th September 2018
Abstract
Macrocycle-based amphiphiles, including macrocyclic amphiphiles and macrocyclic host-based supra-amphiphiles, are a class of amphiphiles which are capable of self-assembling into multidimensional assemblies with defined nanostructures. By involving host–guest recognition, these amphiphiles can be tailored to fabricate new topological structures and fulfill multiple applications. Compared with covalent-bonded traditional amphiphiles and polymeric amphiphiles, the introduction of host–guest interactions facilitates the design, synthesis and controllability of these amphiphilic systems. Moreover, host–guest interactions usually possess stimuli-responsive properties, which further endow self-assemblies of macrocycle-based amphiphiles with fantastic functions. In this review, we summarize recent progress in macrocyclic amphiphiles and macrocyclic host-based supra-amphiphiles, with a focus on novel functions and applications of self-assemblies of these amphiphiles.
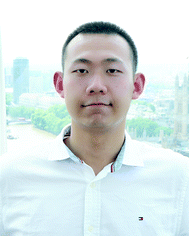
Huangtianzhi Zhu
| Huangtianzhi Zhu was born in China in 1993. He obtained BS degree from Zhejiang University in 2016, and then he joined Prof. Feihe Huang's group to pursue his PhD in chemistry. His current research is focused on pillararene-based functional materials. |
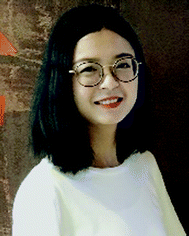
Liqing Shangguan
| Liqing Shangguan was born in Jiangxi, China in 1994. She got her BS from Sichuan University. Then she joined the laboratory of Prof. Feihe Huang at Zhejiang University in 2015 to pursue her PhD in chemistry. Her current research is focused on pillararene-based host–guest chemistry. |
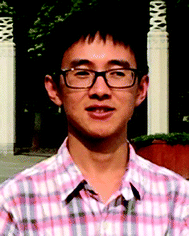
Bingbing Shi
| Bingbing Shi received his BS degree in chemistry from Lanzhou University. In 2014, he joined the laboratory of Prof. Feihe Huang at Zhejiang University to pursue his PhD in chemistry. His current research interests are focused on pillararene-based supramolecular functional materials. |
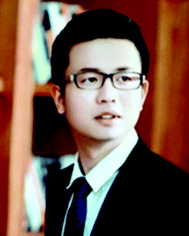
Guocan Yu
| Guocan Yu was born in Zhejiang, China in 1987. He received his PhD degree from Zhejiang University in 2015 under the direction of Prof. Feihe Huang. Then he joined Dr Xiaoyuan (Shawn) Chen's Laboratory of Molecular Imaging and Nanomedicine (LOMIN) in National Institutes of Health (NIH) as a postdoctoral research fellow. His current research interests are mainly focused on the development of supramolecular nanomedicines and smart nanomaterials for supramolecular theranostics. |
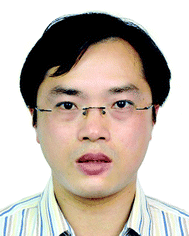
Feihe Huang
| Feihe Huang was born in China in 1973. He obtained his PhD from Virginia Tech under the guidance of Prof. Harry W. Gibson in March 2005. Then he joined Prof. Peter J. Stang's group at University of Utah as a postdoctor. He became a Professor of Chemistry at Zhejiang University in December 2005. His current research interests are supramolecular polymers, pillararene supramolecular chemistry, and nonporous adaptive crystals. The awards he received include the Chinese Chemical Society AkzoNobel Chemical Sciences Award and the Cram Lehn Pedersen Prize in Supramolecular Chemistry. He has published more than 230 supramolecular chemistry papers. His publications have been cited more than 18 622 times. His h-index is 72. He has served as a guest editor for Chem. Soc. Rev., Acc. Chem. Res., Chem. Rev., Adv. Mater., and Chem. Commun. He sits/sat on the advisory/editorial boards of Chem. Soc. Rev., Chem. Commun., Macromolecules, ACS Macro Lett., Polym. Chem., and Mater. Chem. Front. |
1. Introduction
Self-assembly systems, prepared from simple molecules or macromolecules connected by non-covalent interactions, can offer new functions wholly different from those of their individual parts.1 Self-assemblies exist widely in nature: cell membranes, folding proteins, DNA, microtubules, and so on.2 Among these bio-structures, biological membranes consisting of amphiphilic phospholipids show critical importance to living organisms. The amphiphilic properties of these molecules make them self-assemble into bilayers by turning the hydrophilic phosphate groups to the aqueous medium, and the hydrophobic alkyl chains toward the inside of the bilayers.3 The natural arrangements and self-assemblies of phospholipids have attracted a lot of attention from scientists focusing on amphiphiles. Conventionally, amphiphiles are a class of compounds containing both hydrophilic and hydrophobic groups connected by covalent bonds.4 In aqueous media, amphiphiles commonly self-assemble into well-defined structures, such as nanoparticles, micelles, nanosheets, nanorods, and vesicles, which can be applied in numerous fields.5 The morphologies and applications of self-assemblies formed by amphiphiles are determined not only by the interactions of hydrophilic–hydrophobic balance and steric hindrances but also by the external conditions (pH, temperature, concentration, or ionic strength).5g,6 In a biomimic manner, various amphiphiles have been rationally designed and synthesized to construct bioactive structures in order to achieve complex applications, typically nanoparticles and vesicles for drug/gene delivery in cancer theranostics.5c–f
The studies based on amphiphiles have been developed and extended to polymers,7 which are termed as polymeric amphiphiles. Compared with the self-assemblies formed by small amphiphilic molecules, the self-assemblies of polymeric amphiphiles have better sustainability and more controllable size due to the stronger hydrophilic–hydrophobic interactions and slower rate of dissociation and molecular exchange. These properties have endowed polymeric amphiphilic self-assemblies with unique applications, including bio-macromolecular delivery, catalysis, sensors, and templated synthesis of nanodevices.8 Nevertheless, the design, synthesis and separation of covalent-bonded polymeric amphiphiles are extremely difficult and time-consuming, greatly limiting their applications.
Supramolecular chemistry, arising from the discovery of crown ethers in the 1960s, has been awarded the Nobel Prize twice.9 It mainly studies complexes or assemblies formed by recognition between building blocks through non-covalent bonds, such as hydrogen bonding, hydrophilic–hydrophobic interactions, π–π stacking and electrostatic interactions.10 The dynamic and reversible nature of non-covalent interactions has attracted lots of attention from scientists in the fields of chemistry, materials science, biology and engineering. Enlightened by supramolecular chemistry, supramolecular amphiphiles, which are the combination of supramolecules and traditional amphiphiles, have been a research hotspot for decades. Among them, macrocycle-based amphiphiles exhibit distinctive properties not only in unique topological structures but also in self-assembly behaviours.11 Two main kinds of macrocycle-based amphiphiles are well-known:12 macrocyclic amphiphiles and macrocyclic host-based supra-amphiphiles (Fig. 1, here using pillar[5]arene as a model macrocycle). Macrocycle-based supra-amphiphiles are constructed on the basis of non-covalent interactions, in which the hydrophilic and hydrophobic parts are attached by various non-covalent interactions, avoiding complicated covalent synthesis or monomer modifications.13 A macrocyclic amphiphile contains both hydrophilic and hydrophobic groups in one macrocycle but on respective sides of the macrocycle. The cavities of macrocyclic amphiphiles can be used to recognize specific guests via inclusion complexations in order to fabricate new topological structures.14 Compared with traditional amphiphiles, various functional groups and multiple components endow macrocycle-based amphiphiles with excellent self-assembly ability in constructing multi-dimensional and hierarchical materials for different applications, typically for bio-related applications such as cargo delivery, cell imaging and controlled release. Some of the important examples in biochemistry are mentioned in the following parts. Furthermore, the reversible and dynamic characteristics of host–guest interactions endow supramolecular amphiphiles with awesome stimuli-responsiveness, which promotes their applications in sensing, transmission and other smart materials.15 Considering the advances of macrocycle-based amphiphiles, the related studies not only enrich the field of supramolecular chemistry but also benefit the development of functional self-assemblies and smart nanodevices.
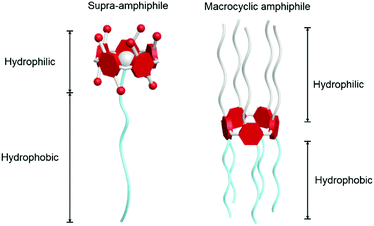 |
| Fig. 1 Cartoon illustrations: a macrocyclic host-based supra-amphiphile (left); a macrocyclic amphiphile (right). Pillar[5]arene derivatives are used here as examples. | |
As more and more scientists contribute to the expansion of macrocycle-based amphiphiles, though we have published reviews in this field,16 we herein summarize the results in the recent two years with a main focus on novel functions and applications of self-assemblies of these amphiphiles. The cited references are not limited to the recent two years, but the examples that are thoroughly discussed are recent progress and these examples are not overlapped with previous reviews we have published. The studies in the field of amphiphiles are commonly concerned with novel molecular structures, controllable self-assembled morphologies and behaviours, multiple stimuli-responsiveness, functional materials and biomedical applications. The following discussions about macrocycle-based amphiphiles are categorized by calixarenes, cyclodextrins, cucurbiturils and pillararenes since these macrocycles have been widely studied. The studies based on other macrocycles,17 albeit excellent, are not discussed in detail here.
2. Cyclodextrin-based macrocyclic amphiphiles and supra-amphiphiles
Cyclodextrins (CDs) were discovered in 1891 by Villiers and the first CD structure was reported by Schardinger in 1903, which was conceived as a toroidal shape containing D-glucose units connected by α-1,4-glucosidic linkers with a narrow rim having primary hydroxyl groups and a wide rim having secondary hydroxyl groups.18 The commonly discussed CDs are α-, β-, and γ-cyclodextrins consisting of six, seven and eight D-glucopyranose units, respectively. The detailed structures and sizes are listed in Fig. 2a. Due to the existence of the hydroxyl groups, the exterior of the CD cavity is highly polarized, whereas the interior of the cavity is non-polarized, allowing CDs to be feasible hosts for non-polarized guests in supramolecules.17a,19 In host–guest chemistry, non-covalent interactions involved in CD-based complexes are mainly van der Waals interactions and hydrophilic–hydrophobic interactions between the non-polarized inner cavity of CDs and the hydrophobic groups of the guests. The classic guests for CDs are shown in Fig. 2b. The binding affinities and selectivities between CDs and specific guests are determined by the cavity size of each CD.20 Notably, adamantine (ADA) derivatives are frequently used to fabricate β-CD-based host–guest systems, and the association constant (Ka) between ADA and β-CD is measured to be 4 × 104 M−1.21 Moreover, the complexation between CDs and guests can be adjusted by external stimuli, such as temperature, pH, redox, light and competitive molecules, which paves the way to construct stimuli-responsive materials. Taking advantage of their simple synthesis, high guest affinity and controllable complexation, supramolecular amphiphiles based on CDs have been well-established and applied in stimuli-responsive systems. Compared with artificial macrocyclic hosts, CDs can be obtained from natural products possessing excellent biocompatibility, low cytotoxicity and biodegradability, making them ideal building blocks for the fabrication of nanomedicines. Indeed, CD-based supramolecular amphiphiles have been widely used in biochemical systems including drug/gene delivery systems, bio-responsive systems and novel therapeutics.22
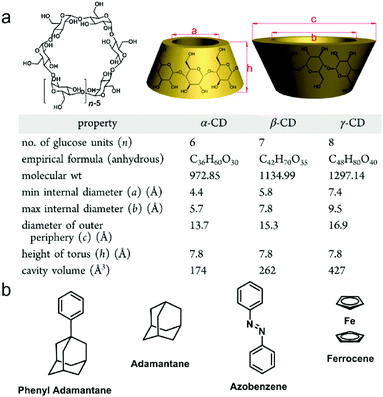 |
| Fig. 2 (a) Chemical structures and size data of typical cyclodextrins and (b) chemical structures of typical guest molecules for cyclodextrins. Adapted with permission from ref. 17a. Copyright 2015 American Chemical Society. | |
2.1 Cyclodextrin-based drug delivery systems
Various clinic drugs including paclitaxel (PTX), doxorubicin (DOX), phenytoin and aliskiren show outstanding effects in anti-cancer, anti-seizure and anti-hypertension therapies but suffer from poor water solubility, which limits their applications. In order to improve their pharmaceutical uses, new methods to enhance their solubility are required.22e,23 In early studies, CDs and their derivatives have been used as hosts to encapsulate drug molecules to form host–guest complexes.22a,b Compared with free drug molecules, the bioavailability and stability of the inclusion complexes were higher, and yet the solubility of the drugs had fairly limited improvement. Not only solubility, but also specificity should be considered, because chemotherapeutic drugs, especially anti-cancer drugs, usually have undesirable side effects (fatigue, nausea, etc.).24 To promote the targeted therapeutic capacity, nanocarrier approaches of drug delivery have been developed. Platforms such as liposomes, micelles, nanoparticles and dendrimers have been involved.25 In pursuit of effective, simple and biocompatible carriers for drug delivery, supramolecular amphiphiles have been taken into consideration. As expected, using self-assemblies of supramolecular amphiphiles exhibits excellence in favour of enhanced solubility of hydrophobic drugs, prolonged circulation time of drugs in the blood, and high tumor accumulation and controlled release of drugs.
Many scientists make contributions to this field. For example, Xie et al. fabricated supramolecular amphiphile-based binary vesicles by using β-CD and the PTX dimer (1), and the vesicles showed abilities of reversible morphology transformation and drug delivery (Fig. 3).26 From proton nuclear magnetic resonance (1H NMR), the host–guest recognition between β-CD and 1 was confirmed. The complexation stoichiometry ratio of β-CD and 1 was determined to be 2
:
1 by UV-vis spectroscopy. Due to the formation of a supra-amphiphile of the β-CD/1 inclusion complex, highly ordered morphologies of aggregations were observed by transmission electron microscopy (TEM), scanning electron microscopy (SEM) and atomic force microscopy (AFM). The β-CD/1 complex self-assembled into nanovesicles (NVs) with hydrophilic β-CD layers in an aqueous medium with an average diameter of 230 nm. Considering the α-amylase induced digestion of CDs and a competitive guest (adamantanamine hydrochloride, 2) caused disassembly of β-CD/1, the β-CD/1 NVs transformed into nanoparticles (NPs) formed by 1 upon addition of α-amylase or 2,27 arising from the disassociation of the host–guest complexation. Besides, these morphological transformations were reversible if excess β-CD were added again. Compound 1 in the NVs not only served as building blocks for constructing NVs but also acted as an anti-cancer therapeutic drug. Notably, the hollow cavities and the 1 layers of NVs could further encapsulate both hydrophilic and hydrophobic molecules to achieve synergistic efficacy, and the supra-amphiphilic nature of NVs benefited controlled release triggered by external stimuli (α-amylase or 2). After encapsulating DOX in β-CD/1 NVs (DOX@β-CD/1 NVs), the evaluation of the cellular uptake and the cytotoxicity of DOX@β-CD/1 NVs demonstrated the active endocytosis through the endo/lysosomal pathway and higher cytotoxicity compared with free NVs. In this work, the solubility of PTX was enhanced by CD-based recognition rather than covalent modification, and the PTX dimer was used as a building block to form vesicles. This work has opened a gate for the application of CD-based supra-amphiphiles in drug delivery.
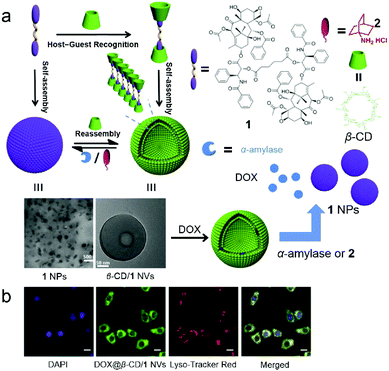 |
| Fig. 3 (a) Schematic representation of the formation of vesicles for controlled release. (b) Confocal laser scanning microscopy (CLSM) images of HepG2 cells incubated with DOX@β-CD/1 nanovesicles. Adapted with permission from ref. 26. Copyright 2017 American Chemical Society. | |
2.2 Cyclodextrin-based stimuli-responsive materials for detection and sensing
Stimuli-responsive supramolecular materials have been paid a lot of attention for decades, and their wide applications in sensors and smart functional self-assemblies have been well-established. Unlike traditional covalent-linked materials, supramolecular materials involving non-covalent interactions have better performances in rapid, reversible and specified responsiveness as well as in avoiding complicated covalent synthesis. External stimuli such as pH, light, temperature and even compounds have been used in these systems.28 Among these stimuli, additional compounds, typically biochemical compounds, are of special interest because biochemical compound-responsive systems with high sensitivity, fast results and specificity facilitate diagnostics, environment monitoring, drug discovery and food safety.29
Enzyme-responsive supra-amphiphiles are a class of non-covalent connected amphiphiles that undergo macroscopic transitions triggered by specific enzymes. Due to the key roles of enzymes in health and disease, enzyme-responsive materials are frequently exploited.30 Zhang et al. reported an amylase-responsive system by supramolecular recognition between β-CD and a naphthalene-containing guest (Fig. 4a).31 A covalent bola-type amphiphile (3) was synthesized, and 3 preferred to self-assemble in water by stacking its hydrophobic parts. When the concentration of 3 increased, the formation of aggregates was suggested by the red shift of the π–π* transition absorption band in UV-vis spectra (Fig. 3b). Meanwhile, the fluorescence of naphthalene was quenched because of aggregation. Interestingly, the fluorescence could be significantly enhanced by adding CDs, and the enhancements triggered by size-matched α- and β-CD were more effective than that of the overlarge γ-CD (Fig. 4c). The recovery of fluorescence was due to the increase of free naphthalene moieties from the nonemissive aggregated state by host–guest complexation. Due to the dynamic characteristic of supra-amphiphiles, the supra-amphiphilic 3/β-CD system exhibited amylase-responsive properties. β-CD was hydrolyzed along with the fluorescence quenching of the naphthalene fluorophore when exposed to amylase. The relevant mechanism was introduced as the disassociation of the 3/β-CD supra-amphiphile and the formation of the nonemissive aggregated state of 3 after adding the enzyme. This system had a potential application in quantitive determination of the activity of α-amylase.
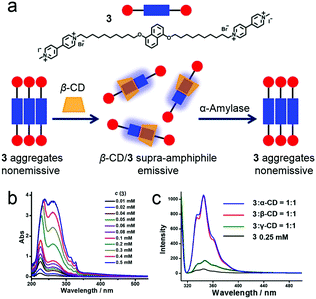 |
| Fig. 4 (a) Schematic representation of the enzyme-responsive supra-amphiphile. (b) UV-vis spectra of 3 with varying concentration. (c) Fluorescence spectra of 3 before and after adding cyclodextrins. Adapted with permission from ref. 31. Copyright 2016 American Chemical Society. | |
In addition to enzymes, carbohydrates are well-known molecules involved in cell adhesion and communication, embryo growth, regulation of protein functions, cancer metastasis and so on.32 Thus, there is an increasing demand for developing carbohydrate-responsive systems and correlative sensors. For recognizing and binding carbohydrates, boronic acids, which can covalently yet reversibly bind 1,2- or 1,3-diols, are considered as synthetic lectins. The design strategy includes a well-chosen “reporter”, such as alizarin red S (ARS), which displays a remarkable change in absorption or emission when it is connected or disconnected to boronic acids.
Recently, Ravoo et al. prepared a carbohydrate-responsive self-assembly based on the macrocyclic amphiphile β-CD and host–guest chemistry (Fig. 5a).33 According to previously studies, macrocyclic amphiphilic β-CD (5) self-assembled into bilayer vesicles (β-CDV) in an aqueous medium. Besides, the surfaces or the components of the vesicles could be decorated by host–guest recognition.34 Therefore, an adamantane-containing guest molecule (4) was chosen in order to form inclusion complexes with 5 and immobilize the boronic acid on the surface of β-CDV. Additionally, the strength of interaction between 4 and carbohydrate (D-fructose and D-glucose) increased with the increase of pH. These effects were caused by the enhanced nucleophilicity of the diol in base conditions and the high pKa of carbohydrates. The “reporter” in this system was ARS, whose fluorescence was dramatically enhanced when it was associated with boronic acid due to the suppression of intramolecular proton exchange. Therefore, the fluorescence of ARS increased in the presence of 4 (Fig. 5b). Interestingly, in the presence of β-CDV, the binding for 4 and ARS was reinforced, and stronger fluorescence of ARS was observed as a result of high local concentrations of ARS and 4 on the surfaces of β-CDV. In the titration of carbohydrates into a solution of 4/ARS coated β-CDV, the fluorescence of ARS decreased because the carbohydrates bound 4 and liberated ARS (Fig. 5c). By involving amphiphilic β-CD, self-assembled vesicles could be simply prepared. Moreover, the host–guest recognition enriched ARS on the vesicle surfaces, resulting in higher fluorescent intensity and sensitivity. These results are helpful for the design and development of carbohydrate sensors and even more sophisticated self-assembled materials using supramolecular amphiphiles.
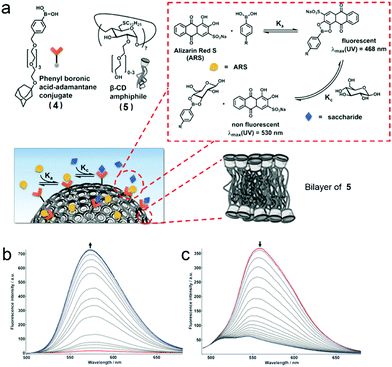 |
| Fig. 5 (a) Schematic representation of the formation of bilayer vesicles with sensing ability. (b) Fluorescence spectra of titration of Alizarin Red S (ARS) with 4. (c) Fluorescence titration of 4 with fructose in the presence of ARS and bilayers vesicles (β-CDV). Adapted with permission from ref. 33. Copyright 2017 John Wiley and Sons. | |
2.3 Cyclodextrin-based self-assemblies for photodynamic therapy and cell imaging
Photodynamic therapy (PDT) is a promising therapeutic modality against tumors with minimum invasiveness, low cost and satisfactory anti-tumor outcomes.35 Conventional therapeutics such as chemo- and radiation therapies always suffer from drug resistance, cumulative toxicity and inevitable side effects. However, these drawbacks can be avoided by PDT.36 During the PDT process, three key elements are involved: a photosensitizer (PS), light irradiation and produced reactive oxygen species (ROS). The main ability of PS is to transfer the energy of light irradiation to surrounding oxygen molecules in the tissue to produce ROS. Upon the stimulation of ROS, cell apoptosis and necrosis are triggered resulting in cell death.37 Therefore, scientists have been dedicated to the discovery of efficient PSs for a long time. Porphyrin derivatives such as 5-(4-hydroxyl phenyl)-10,15,20-triphenyl-porphyrin (TPP) are a class of novel PSs with unique abilities to generate ROS. Nevertheless, due to the severe π–π stacking between hydrophobic porphyrin rings, the fluorescence and the generation of ROS are completely inhibited.38 To solve the problem, although numerous methods have been applied to disperse the porphyrin moieties, for example porphyrin-coupled nanovesicles,39 there still remain challenges in designing and constructing stable, efficient and bioavailable porphyrin-containing systems.
The self-assemblies of supramolecular amphiphiles are considered as awesome nanocarriers for porphyrin derivatives because porphyrin units can be enriched in the hydrophobic regions of the self-assemblies without hampering photosensitivity. Enlightened by this principle, Huang et al. applied self-assemblies of supra-amphiphiles formed by polyethylene glycol (PEG) modified β-CD (6) and carborane derivatives (7) as nanocarriers for porphyrins in PDT (Fig. 6).40 Carborane, which has a spherical shape, ideal size and hydrophobic surface, was introduced into the system as an appropriate guest for β-CD with a high association constant. Two supra-amphiphiles, namely two complexes of 8 and 9 with 2
:
1 and 1
:
1 binding stoichiometries, respectively, were prepared, and both supra-amphiphiles self-assembled into NPs in an aqueous medium by turning hydrophilic PEG chains towards water. The NPs were capable of encapsulating TPP to boost the PDT efficiency by prohibiting TPP aggregation, and TPP@9 NPs showed excellent stability and water dispersibility. In vitro studies indicated TPP@9 NPs exhibit negligible cytotoxicity to normal human cells in the dark. Upon light irradiation, TPP@9 NPs showed higher phototoxicity against HepG2 and HeLa cells than free TPP at low drug concentration. Besides, confocal laser scanning microscopy (CLSM) indicated that the efficacy of cellular uptake of TPP@9 NPs was higher than free TPP aggregates, which was a distinct advantage of supra-amphiphiles. More importantly, the hydrophobic parts of self-assemblies provided proper cavities for hydrophobic TPP, and this prevented TPP aggregation. The self-assembly promoted PDT not only enriched the families of supra-amphiphiles but also exhibited new applications of CD-based supramolecular systems.
 |
| Fig. 6 Schematic representation of the formation of supra-amphiphiles and the mechanism of the PDT process. Adapted with permission from ref. 40. Copyright 2017 Royal Society of Chemistry. | |
Cell imaging is a versatile method to observe cell morphology and study biological processes, which requires the development of fluorescent materials. Ultra-small fluorescent nanostructures which have non-linear optical activity provide a broad range of applications such as imaging of biological matter.41 The main types of ultra-small non-linear optical materials are constructed by dendrimers and polymer dots through covalent synthesis.42 Supramolecular self-assembly offers an alternative approach in such an area with simple preparation and dynamic characteristics.43 Stuparu and co-workers reported precise and well-dispersed fluorescent dots prepared by cyclodextrin-based self-assembly and applied them in cell imaging (Fig. 7a).44 The host–guest recognition between hydrophilic γ-CD and hydrophobic dimethylaniline-containing corannulene (10) was used to fabricate supra-amphiphiles. The dimethylaniline group on 10 promoted intramolecular charge transfer (ICT), which enhanced the optical activity of corannulene, and the hydrophobic corannulene could be encapsulated by γ-CD via hydrophobic interactions. Thus, green luminescent ultra-small dots (2.5–5 nm) formed by the supra-amphiphilic γ-CD/10 complex were observed, and these luminescent dots displayed an acid-responsive property. When 10 was protonated, the dimethylaniline groups became cationic and hydrophilic, and the amphiphilicity of the complex was retarded, resulting in the destruction of ultra-small dots. The optical studies revealed that a two-photon up-conversion process participated in the emission mechanism (Fig. 7b). For achieving bio-application, γ-CD/10 dots were further applied in cell imaging. The fluorescence of MCF-7 cells incubated with γ-CD/10 dots was recorded using two-photon microscopy, and the resultant images demonstrated the good intracellular distribution of the dots in the cytoplasm (Fig. 7c). The donor–acceptor moiety in the guest molecule ensured bright luminescence and two-photon activity, and the CD-based host–guest interactions rendered the nanostructures water dispersibility and biocompatibility. This work established a novel supra-amphiphile as a two-photon bio-imaging agent which showed the wide application of cyclodextrin-based supramolecular chemistry in the biological field.
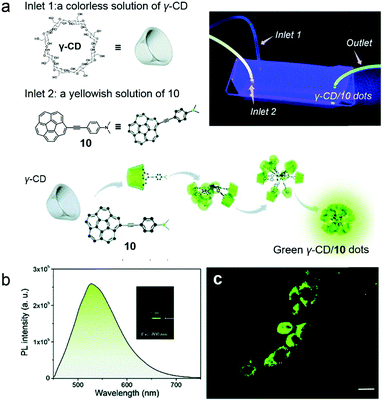 |
| Fig. 7 (a) Schematic representation of the formation of green emissive dots. The inset photograph is the fluidic device during performance and illuminated with 365 nm UV irradiation. (b) Two-photon excited emission of γ-CD/10 dots in water. (c) Two-photon microscopy images of the MCF-7 cell line incubated with γ-CD/10 dots. Adapted with permission from ref. 44. Copyright 2017 Royal Society of Chemistry. | |
3. Calix[n]arene-based macrocyclic amphiphiles and supra-amphiphiles
Calix[n]arenes are macrocyclic hosts which were developed during the 20th century.45 Their cyclic structures consist of phenol units and methylene bridges. Commonly, the methylene-linkers at meta-positions endowed calixarenes with basket-like conformations and well-defined cavities, which have abilities to encapsulate proper guests.45c,e,46 The modification of calixarenes has been the focus for many decades in calixarene chemistry, because both the upper and lower rims of calixarenes can be functionalized. The functional groups in modified calixarenes are selected for enhancing their solubility in different media, promoting their affinities to target guests, fulfilling complicated applications, or even self-assembling to regular polyhedra.47 Apart from basic calix[n]arenes, their analogues including oxacalixarenes, thiacalixarenes, azacalixarenes and resorcinarenes have been well studied. Due to the wide range of the calixarene families, calix[n]arenes are considered as the third generation of macrocyclic hosts after crown ethers and CDs.
In an early report, Regen et al. prepared calix[6]arene-based vesicles by adding a tetrahydrofuran solution of bare calix[6]arene into water.48 From then on, the fabrication of amphiphiles using calix[n]arenes has received a lot of attention because the rigid and conic structures of calixarenes not only ameliorate the stabilities of self-assemblies but also benefit the formation of high-curvature aggregations of amphiphiles. Therefore, various kinds of self-assemblies including vesicles, nanosheets, nanoparticles and nanotubes involving calixarene-based supramolecular amphiphiles have been developed.49 Among these amphiphiles, water-soluble p-sulfonatocalix[n]arenes (SCXn, n = 4–8) are particularly versatile building blocks in constructing supra-amphiphiles owing to their electron-rich cavities and negative charges (Fig. 8).50 Endowed with high solubility, guest/cation affinity and biological compatibility, sulfonatocalix[n]arenes have been utilized in various fields.51 Besides, the self-assembled morphologies could be altered by changing the number of phenol units and external stimuli.
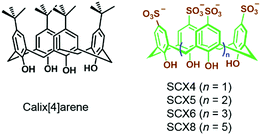 |
| Fig. 8 Chemical structures of calix[4]arene and water-soluble p-sulfonatocalix[n]arenes. | |
3.1 Sulfonatocalix[n]arene-based supramolecular amphiphiles with tunable self-assembly morphologies
Biczók et al. reported a supra-amphiphilic sulfonatocalix[6]arene (SCX6) and 1-methyl-3-tetradecyl-imidazolium bromide (C14mim+Br−) complex which could self-assemble into NPs in an aqueous medium, and the diameters of NPs increased with NaCl concentration.52 Besides, the NPs transformed into supramolecular micelles (SMs) at high NaCl concentration. In order to figure out how the macrocyclic size influenced the self-assembly morphologies, Biczók further investigated the self-assembly behaviours of SCXn (n = 4, 8) and C14mim+Br− (11) complexes (Fig. 9).53 SCX4 and 11 self-assembled into NPs or coagulation species in different SCX4/11 molar ratios. In contrast to SCX6/11 NPs, the NPs formed by the SCX4/11 complex were barely influenced by the changes of the NaCl concentration. When SCX8 was used instead of SCX4, the self-assembled NPs displayed tunable morphologies with different SCX8/11 molar ratios and NaCl concentrations. For example, once the molar ratio dropped below 6.8 (such as 3.9), the NPs transformed into SMs with the increase of the NaCl concentration. Deeper understanding of the transformation was achieved by isothermal titration calorimetry (ITC). It was found that SCX4 promoted the formation of NPs at a suitable SCXn/11 molar ratio, whereas the self-assembly of SCX8/11 into NPs was dominated by an endothermic process, and the transformation from NPs to SMs was an exothermic process. The enthalpy of SCX8/11 NPs increased in the presence of NaCl, making the transformation to SMs more favourable. This work not only described the effect of the macrocyclic size on self-assembly, but also introduced a supra-amphiphilic system with controllable morphologies, which have potential applications in stimuli-responsive systems and smart materials.
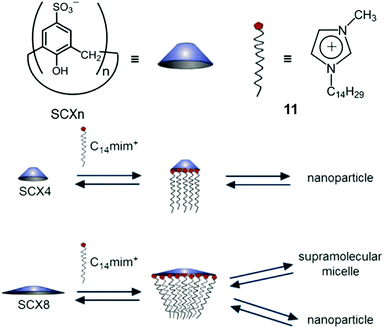 |
| Fig. 9 Schematic representation of different self-assemblies formed by different p-sulfonatocalix[n]arenes (SCXn, n = 4 or 8). Adapted with permission from ref. 53. Copyright 2017 American Chemical Society. | |
3.2 Sulfonatocalix[n]arene-based supramolecular amphiphiles with photo-responsiveness
Apart from the morphological transformation, SCXn have been applied in stimuli-responsive systems by using their host–guest interactions. Light is considered as a clean, efficient and convenient stimulus to regulate molecular structures, aggregation behaviours and other properties.54 In the field of photo-responsive systems, light-triggered chemical reactions attract lots of attention because of the low cost and rapidity. Photolysis, which refers to the compound being able to decompose under light irradiation, has been applied in the fabrication of degradable or stimuli-responsive materials.55,56 By employing supramolecular amphiphiles in photolyzable systems, novel photo-responsive materials with multiple functions and structures could be obtained.
Liu et al. fabricated a photolyzable supra-amphiphilic system using SCX4 and an anthracene derivative (12).57 SCXn are frequently used to associate cation guests via π–π interactions and electrostatic interactions. SCX4 and 12 formed a 1
:
1 supra-amphiphile and self-assembled into NPs with an average diameter of 266 nm (Fig. 10a). By forming a host–guest complex with SCX4, the fluorescence of 12 was enhanced due to the inhibition of photoinduced electron transfer (PET).58 It's known that photolyzable 9-alkoxy-substituted anthracene can be activated by UV light followed by reacting with oxygen to generate anthraquinone and alkanol.59 To estimate the photolysis efficiency, the absorption of 12 was measured, and only a small amount of 12 degraded under irradiation with UV light at 365 nm for 30 min. However, the absorption of 12 in SCX4/12 significantly decreased within the same irradiation time, demonstrating the self-assembly of SCX4/12 promoted the photolysis of 12. Additionally, the photolysis of SCX4/12 self-assembly occurred much faster in oxygen atmosphere than in argon (Fig. 10b). SCX4/12 NPs disassembled after UV irradition, which was ascribed to the formation of anthraquinone precipitation and hydrophilic alkanol. This photolysis process was also achieved by visible light in the presence of Eosin Y (ESY) as an auxiliary photosensitizer (Fig. 10c). The possible reason for the photolysis enhancement was assumed as the self-assembly of supra-amphiphile SCX4/12 not only providing a hydrophobic core to elongate the lifetime of singlet oxygen but also promoting the contact between 12 and singlet oxygen in confined NPs. Moreover, the designed supra-amphiphile system also inhibited the fluorescence quenching of 12, which facilitated the photosensitization. This self-assembly promoted photolysis offers a promising strategy in the field of photo-responsive systems and the photodegradation of pollutants.
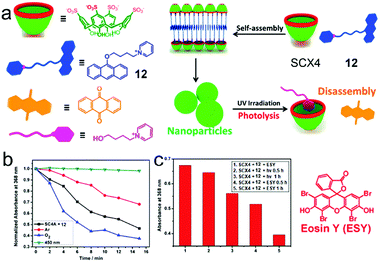 |
| Fig. 10 (a) Schematic representation of the photolyzable supra-amphiphilic system. (b) The absorbance of the SCX4/12 assembly at 368 nm upon irradiation for different amounts of time. (c) The absorbance of the SCX4/12 assembly at 368 nm in the absence and presence of eosin upon irradiation at 520 nm for different amounts of time. Adapted with permission from ref. 57. Copyright 2017 American Chemical Society. | |
3.3 Calix[n]arene-based macrocyclic amphiphiles for optical materials
With the advantage that both the upper and lower rims of calixarenes can be functionalized, calixarenes with both hydrophilic and hydrophobic groups located on respective sides, which are termed as macrocyclic amphiphilic calixarenes, are promising to synthesize. Due to the surfactant nature of these modified calixarenes, they conveniently self-assemble into various nanostructures such as vesicles and nanoparticles. Moreover, the high guest affinity of calixarenes endows the self-assemblies with multiple functions.
To achieve tunable photoluminescent materials, light-harvesting systems are exploited for generating energy migration-involving multiple fluorescent emissions.60 Because traditional covalent bond linked donor/acceptor luminophores suffer from sophisticated synthesis or limited controllability, the design of photoluminescent materials based on supramolecular self-assembly is attractive. Non-covalent interactions not only organize donor/acceptor luminophores in specific binding environments but also allow the systems to be controllable.61 In this regard, Guo et al. reported macrocyclic amphiphile-based broad-spectrum tunable photoluminescent materials (Fig. 11a).62 Both of the two amphiphilic calixarenes (13 and 14) prepared by them self-assembled into vesicular aggregates in water, and 14 assemblies were more stable. Compared with free guest 15, the complex displayed enhanced fluorescence, which was ascribed to the host–guest complexation-induced restriction of the intramolecular rotation of the fluorophore. The strong fluorescence allowed 15 to be a donor candidate in fabricating donor/acceptor luminophores. Considering the spectrum overlap, a hydrophobic benzothiadiazole derivative (16) was chosen as an acceptor, and the acceptors were confined in the hydrophobic layers of the self-assemblies, which endowed the system with the Förster resonance energy transfer (FRET) phenomenon from 15 to 16. Notably, in phosphate buffered saline (PBS), the energy transfer efficiency (ΦET), the number of 15 chromophores in one light-harvesting unit (n) and the antenna effect of the FRET system showed better performance (ΦET = 97%, n = 105, antenna effect = 6.6, Fig. 11b) compared with the system in pure water. The explanation was that the self-assembly of ionic amphiphiles could be boosted by increasing the ionic strength, which further led to more compact aggregation and promoted the energy transfer efficiency. Interestingly, this light-harvesting system exhibited conveniently controllable emission color achieved by changing the donor/acceptor ratio. When the donor/acceptor ratio reached 25
:
1, pure white-light emission was observed (Fig. 11c). This tunable luminescent material was further applied in fluorescent ink. More importantly, by host–guest recognition and self-assembly of the macrocyclic amphiphile, the donors and acceptors were discretely but accurately located in the cavities of the hosts and the bilayers of self-assemblies, leading to simple and versatile preparation of light-harvesting systems.
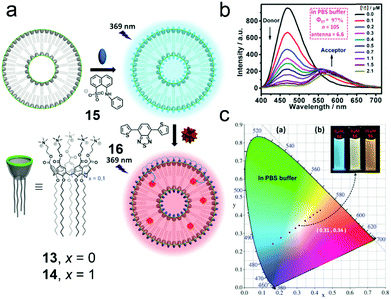 |
| Fig. 11 (a) Schematic representation of the formation of the broad-spectrum tunable photoluminescent materials. (b) Fluorescence spectra of the 14/15 assembly in PBS buffer with different concentrations of 16, λex = 369 nm. (c) The luminescent color changes of the 14/15 assembly with different concentrations of 16 in PBS buffer. Adapted with permission from ref. 62. Copyright 2016 John Wiley and Sons. | |
Additionally, macrocyclic amphiphilic calixarenes can also be used in the preparation of fluorescent NPs. One problem is that fluorophores aggregated in small structures usually produce broad and low emission due to aggregation-caused quenching (ACQ), which severely hampers the fluorescent intensity.63 However, using self-assemblies of amphiphilic calixarenes is a novel approach to solve the problem. Klymchenko et al. fabricated bright fluorescent NPs based on cross-linked calixarene micelles (Fig. 12a).64 An amphiphililc calixarene (17) bearing four alkyne groups was observed to self-assemble into 5 nm micelles by turning its hydrophilic rim towards water thus forming a cross-linkable surface for cyanine dyes (18 and 19). Compared with free dyes, the shell-cross-linked NPs had broadened and red-shifted emission bands and lower fluorescence quantum yields (QY) because of ACQ. Interestingly, the QY of 18 coated NPs changed versus the viscosity of the solvent (Fig. 12b). Solvents with large viscosity (such as glycerol and serum) decreased the internal rotation and mobility of cyanine molecules on the NP surface, which limited quenching. Therefore, 18 coated NPs showed 2-fold higher brightness than quantum dots (QD-585, Fig. 12c) when they were deposited on a glass surface in glycerol. The application of these NPs in bioimaging was explored. The NPs rapidly internalized into cells as stable and bright dots, and mainly distributed in endosomes and lysosomes, achieving excellent bioimaging contrast. This was the first observation of fluorescent NPs that light up in a viscous solvent. The self-assemblies of macrocyclic amphiphilic calixarenes were used as models for dyes, forming NPs with very small hydrodynamic diameters, high stability and superior brightness.
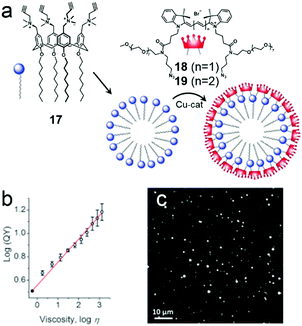 |
| Fig. 12 (a) Chemical structures of amphiphilic calixarene 17 and cyanine dyes (18 and 19) and a schematic representation of the formation of the micelles. (b) Dependence of the fluorescent quantum yield on viscosity. (c) Wide-field fluorescence image of 18 coated NPs deposited on glass. Adapted with permission from ref. 64. Copyright 2016 John Wiley and Sons. | |
Constructing and manipulating optical materials play an important role in the field of pharmacy or biology through phototheranostics. PDT as an emerging non-invasive and reliable therapeutic for cancers has been well established. However, the tumor selectivity and dark toxicity hinder its clinical translation. Therefore, finding “smart” photosensitizers (PSs) becomes a medical demand. Recently, Guo et al. termed a new concept of biomarker displacement activation (BDA) as a general strategy for in vivo targeted phototheranostics (Fig. 13a).65 BDA refers to a procedure in which an active PS (ON state) could be released from the cavity of the receptor when the inactive host–guest complex (OFF state) was exposed to the competitive biomarker. To validate the BDA strategy, an amphiphilic guanidinium-modified calix[5]arene (20) was prepared, which showed strong binding affinities to anionic PS (21) and ATP. Besides, the fluorescence and photoactivity of 21 were annihilated by host–guest complexation, and these properties could almost completely recover in the presence of 100 μM of ATP. Enlightened by these, 21 was preloaded in the NPs self-assembled by amphiphilic 20 and PEG-containing surfactant, and the non-emissive loaded NPs exhibited fluorescence in 4T1 breast cancer cells. The fluorescent intensity could be adjusted by using NaN3 (decrease intracellular ATP generation) or Ca2+ (increase intracellular ATP generation). Moreover, the PDT process could be achieved ascribed to the release of 21 in tumor cells. The BDA strategy has also been applied in selective tumor imaging and PDT in a tumor-bearing nude mouse (Fig. 13b). Compound 21 in loaded NPs was hardly released in normal organs due to the low ATP level and thus a high fluorescence intensity contrast of tumor to normal tissues was observed because of higher ATP expression in tumor cells. Apart from the tumor imaging, released 21 in tumor tissue was also used for PDT. Compared with 21 in the NPs, free 21 not only was enriched in tumor tissue but also emitted strong fluorescence in the abdomen of the mouse, which demonstrated the macrocyclic amphiphilic self-assembly was indispensable in “smart” PSs. The involvement of supramolecular self-assembly also had advantages in simple preparation and wide adaptability to different PSs.
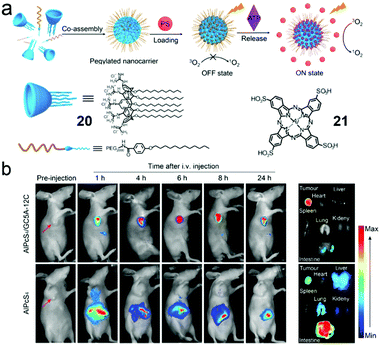 |
| Fig. 13 (a) Cartoon representation of the BDA strategy. (b) In vivo fluorescence imaging of the 4T1 tumor-bearing nude mice. Adapted with permission from ref. 65. Copyright 2018 American Chemical Society. | |
4. Cucurbit[n]uril-based macrocyclic amphiphiles and supra-amphiphiles
Cucurbit[n]urils (CB[n]s) are a huge family of macrocycles with glycoluril moieties linked by two methylene bridges, which were discovered in the 20th century (Fig. 14a).66 Afterward, numerous scientists have made contributions in CB[n] chemistry, and thus CB[n]s (n = 5–8 and even 15) have been successfully synthesized and isolated.67 In accordance with electrostatic potential surface modelling, the carbonyl groups on the respective sides of CB[n]s are negatively charged, which are favoured to bind cationic groups, and the hydrophobic cavity of CB[n]s also facilitates the guest affinity.68 Therefore, the CB[n]s commonly show very high association constants with positively charged and hydrophobic guests (Fig. 14b). Notably, the large cavity of CB[8] is accessible for two molecules together such as a methylviologen guest and a naphthalene guest via non-covalent interactions to form a 1
:
1
:
1 complex.69 Endowed with unique binding properties, CB[n]s have been widely used as water-soluble macrocycles to fabricate fantastic nanostructures, stimuli-responsive systems and drug delivery systems.70
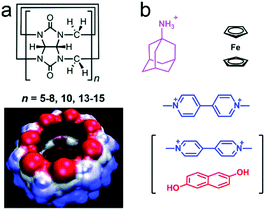 |
| Fig. 14 (a) Chemical structure of cucurbit[n]urils (CB[n]s) and the surface electrostatic potential of CB[7]. (b) Typical guests for CB[n]s. | |
4.1 Cucurbit[n]uril-based photo-responsive systems for controlled release
Photo-responsive systems have attractive potential in constructing molecular switches, drug delivery systems and smart materials due to their high sensitivity, low cost and easy operation.28m,71 As versatile hosts, attaching photo-responsive groups onto CB[n]-based supramolecules is a feasible method to prepare many controllable materials. Azobenzene is one of the most commonly used photo-responsive moieties.72 Yu et al. reported a CB[7]-based azobenzene-containing supra-amphiphile with a light-controlled release property (Fig. 15).73 The proper cavity size of CB[7] allowed itself to be an ideal host for trans azobenzene-containing guest 22, and the azobenzene unit could thread out of the cavity after photoisomerization. Based on this phenomenon, amphiphilic guest 23 was applied as a guest for CB[7] to fabricate a photo-responsive supra-amphiphile. NPs self-assembled from 23 transformed into vesicle-like aggregates in the presence of CB[7], and the vesicles changed into NPs again when the system was exposed to 365 nm UV light, ascribed to the disassociation of CB[7] and cis-23. Dependent on the photo-responsive assembly, calcein was used as a fluorescent indicator in controlled release. As expected, after light irradiation, the vesicles collapsed along with the release of calcein. In this work, the morphology of the self-assemblies could be reversibly controlled by light, leading to potential applications in smart nanomaterials, and it also demonstrated the basic design principle in CB[7]-based photo-responsive systems for drug release.
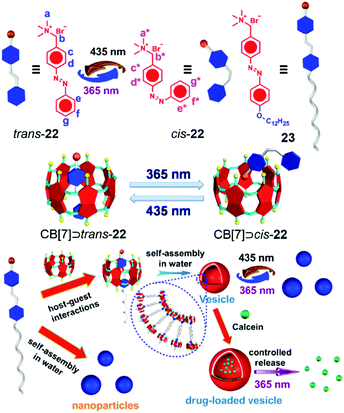 |
| Fig. 15 Schematic representation of the photo-responsive morphological transformation for controlled release. Adapted with permission from ref. 73. Copyright 2016 Elsevier. | |
The development of drug delivery systems with both stimuli-responsive and tumor-targeting abilities has attracted increasing attention.74 CB[8] is capable of forming a 1
:
1
:
1 heteroternary complex via hydrophobic and ion–dipole interactions, which is often regarded as a linker for hydrophilic and hydrophobic segments to construct supra-amphiphiles. Therefore, Liu et al. reported a supra-amphiphile with photo-responsiveness and targeting ability via CB[8], azobenzene (24) and methylviologen (25) derivatives (Fig. 16).75 CB[8] could encapsulate azobenzene and methylviologen at the same time to form a 1
:
1
:
1 complex,70k and thus highly stable giant supramolecular vesicles larger than 800 nm were prepared by self-assembly from the formed CB[8]-based supra-amphiphile. Notably, the trans-azobenzene moieties in the vesicles isomerized to cis-azobenzene groups, which led to the promoted protrusions of the vesicles and drug release. Moreover, the maleimide groups on the surfaces could be functionalized with iRGD, hyaluronic acid (HA) or BSA via “click reactions” which enhanced the specificity and endocytosis efficiency for tumor cells. The DOX-loaded vesicles exhibited slight suppression to cell viability in the dark, whereas increased cytotoxicity was observed under UV light, demonstrating the light-controlled drug release. The CB[8]-based ternary complexation promoted the formation of supramolecular vesicles with light-responsive properties, and the surface modification enhanced specificity and endocytosis efficiency to tumor cells, which not only broadened the application of photo-responsive systems but also offered a new surface-modified strategy to validate various functions.
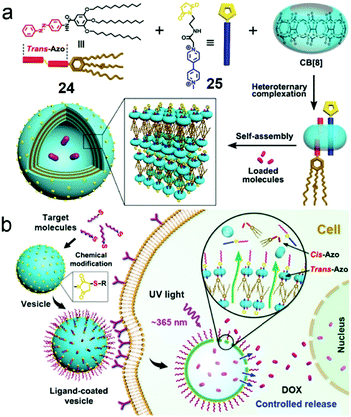 |
| Fig. 16 (a) Schematic representation of the formation of vesicles self-assembled by the CB[8]/24/25 supra-amphiphile. (b) Cartoon representation of the endocytosis and light-controlled release. Adapted with permission from ref. 75. Copyright 2016 American Chemical Society. | |
Apart from the photo-induced isomerization of azobenzene, the thiol–ene “click reaction” is also a feasible way to construct photo-responsive systems. Kim et al. used mono-allyloxylated CB[7] (AO1CB[7]) to build photo-responsive vesicles for drug release (Fig. 17).76 AO1CB[7] performed as an unconventional amphiphile in water, which self-assembled into vesicles with diameters of nearly 960 nm. Intermolecular interactions through the monoallyoxy groups among AO1CB[7] molecules were observed, indicating the monoallyoxy group was crucial to its amphiphilicity and the alternation of the substitution group might change the self-assembly morphology. Therefore, a biogenic thiol, glutathione (GSH), was used to modify AO1CB[7] vesicles. As expected, the vesicles transformed into irregular aggregates due to the formation of hydrophilic GSH-CB[7]. To explore the bio-application of the vesicles, DOX-loaded vesicles were used for controllable drug release. CLSM images demonstrated that DOX mainly located in cytosol and had no obvious release from the vesicles in HeLa cells. The encapsulated DOX could be released accompanied by the collapse of the vesicles under UV irradiation. The unconventional amphiphilicity of AO1CB[7] is quite unique, and its self-assemblies bearing susceptibility to endogenous GSH are simple but reliable drug carriers in controlled release systems.
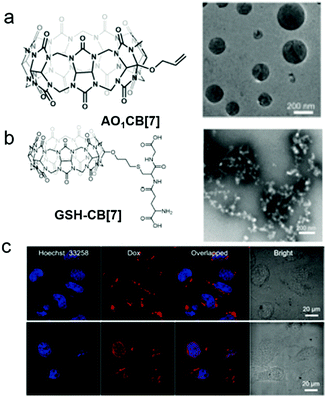 |
| Fig. 17 Chemical structures and TEM images of self-assemblies of (a) AO1CB[7] and (b) GSH-CB[7]. (c) CLSM images of DOX@AO1CB-[7] vesicle treated HeLa cells without (top) and with (bottom) irradiation by a laser (λ = 720 nm). Adapted with permission from ref. 76. Copyright 2018 John Wiley and Sons. | |
4.2 Cucurbit[n]uril-induced formation of FRET-enabled vesicles
FRET as a widely studied energy transfer process has been achieved by using macromolecules or molecular self-assemblies bearing advantages in nanometer size and dynamic nature.77 Self-assemblies based on CB[n]s are not only convenient to fabricate but also able to reduce the distances between fluorophores required by the FRET mechanism.78 Therefore, FRET-enabled vesicles self-assembled by a CB[7]-based supra-amphiphile were constructed by Das et al. (Fig. 18).79 The self-assembly behaviour of an amphiphilic guest 26 containing a naphthalene group was firstly studied and the results suggested the morphologies changed from micelles to vesicles and then to rods with the increase of the concentration. In the presence of CB[7], the supra-amphiphile self-assembled into vesicles and their hydrophobic pockets existed between the bilayers of the vesicles which were capable of entrapping hydrophobic dye. Thus the CB[7]/26 vesicles were applied in FRET system with an efficiency of 81.5% by entrapping anthracene. Moreover, the FRET system showed stimuli-responsiveness to a competitive guest (adamantyl amine) and high temperature, which caused the disruption of the self-assemblies.
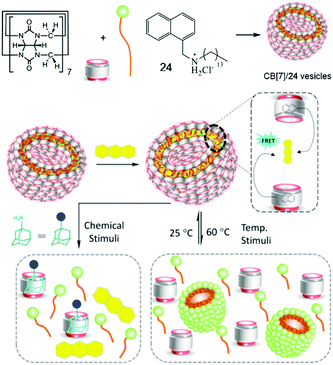 |
| Fig. 18 Schematic representation of the formation of a FRET enabled self-assembly and its stimuli-responsive properties. Adapted with permission from ref. 79. Copyright 2017 American Chemical Society. | |
4.3 Cucurbit[n]uril-based bola-type supra-amphiphiles for supramolecular radicals
Supramolecular radicals, which refer to the radicals stabilized or generated by non-covalent interactions such as host–guest interactions, often show different properties from common supramolecules.80 Benefiting from the dynamic and reversible nature of the supramolecular interactions, supramolecular radicals can be applied in various fields with switchable and adaptive capabilities.81 Zhang et al. elegantly reported a bola-type supramolecular radical used for selective photothermal therapy of bacteria based on host–guest interactions of CB[7] (Fig. 19).82 A perylene diimide guest bearing two ammonium groups (27) exhibited π–π stacking which could quench the radical anion. Besides, the phenyl groups on 27 could show nonspecific membranolysis. By introduction of CB[7], the π–π stacking and membrane permeability were both inhibited by forming the bola-type supra-amphiphile. Besides, negatively charged bacteria surfaces could absorb the positively charged complex, leading to the in situ reduction of perylene diimide to its radical anion by hydrogenases on the surfaces of anaerobic bacteria. The photothermal therapy of anaerobic bacteria was achieved by the perylene diimide radical anion under NIR irradiation. Interestingly, due to the fewer hydrogenases on the surfaces of aerobic bacteria, perylene diimide was hardly reduced, which caused a limited photothermal effect. Therefore, the selective photothermal therapy for anaerobic bacteria was fulfilled by the supramolecular radicals, which had great potential for regulating microbial balance.
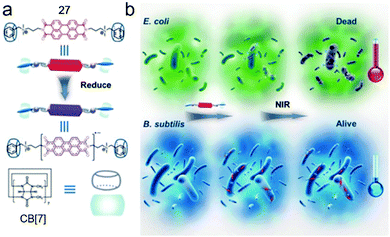 |
| Fig. 19 (a) Chemical structures and formation of the supramolecular radical of CB[7]/27. (b) Cartoon representation of the selective photothermal therapy for anaerobic bacteria. Adapted with permission from ref. 82. Copyright 2017 John Wiley and Sons. | |
Apart from the biological application, the supramolecular radical was also utilized to enhance the Fenton oxidation efficiency (Fig. 20).83 Fenton oxidation is a decomposition reaction of aromatic compounds triggered by H2O2 and Fe3+ through a radical cation intermediate pathway.84 The activity of the radical cation directly influences the rate of the decomposition. As an electrostatically negative host, CB[7] is considered to accelerate the Fenton oxidation by activating the electron-deficient radical. A dipyrrole guest (28) was chosen as a model dye to estimate the enhanced oxidation efficiency. The oxidation process was monitored by UV spectroscopy, and the peak related to 28 decreased much faster in the presence of CB[7], indicating the acceleration of the oxidation reaction. The kinetic study demonstrated the supramolecular complexation decreased the apparent activation energy. Moreover, by calculating the spin-density distribution, the complexation increased the degree of localization of single electrons, rendering the carbon–carbon double bond on pyrole easier to be cleaved. By involving the supra-molecular interactions, the oxidation efficiency of aromatic compounds was highly enhanced, which opened up a new approach for the oxidation of organic pollutants.
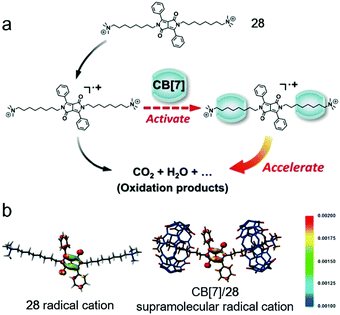 |
| Fig. 20 (a) Schematic representation of CB[7] promoted Fenton oxidation. (b) Spin-density distribution of the 28 radical cation (left) and CB[7]/28 supramolecular radical cation (right). Adapted with permission from ref. 83. Copyright 2016 John Wiley and Sons. | |
5. Pillar[n]arene-based macrocyclic amphiphiles and supra-amphiphiles
Pillar[n]arenes (PA[n]s) are novel macrocyclic hosts reported in 2008.85 Compared with meta-bridged calixarenes, the 1,4-dialkoxybenzene units are connected by methylene bridges in para-positions (Fig. 21). PA[n]s have several distinguishing features, such as symmetric and rigid pillar-shaped architectures, and electron-rich and hydrophobic cavities. These features promote π-electron density in the cavities, which makes PA[n]s prefer encapsulating electron-deficient or neutral guests such as alkyl chains, diamines, ammonium salts, pyridinium salts and viologen moieties.86 The modification of PA[n]s can be achieved easily by converting dialkoxy substitutions to reactive moieties such as the phenol group, ester group, halide moiety, and alkyne group.87 Notably, PA[n]s bearing ten triethylene glycol groups have the lower critical solution temperature (LCST) behaviour in an aqueous medium.12 Phenylethynyl containing conjugated PA[n]s show thermo-responsive blue-green emission.88 Ester groups on PA[n]s can be hydrolysed to carboxyl anions by proper methods, and thus anionic water-soluble carboxylate-containing pillararenes (WP[n]s) can be synthesized, which have strong affinities to cation guests in water.89 More importantly, two different substitutions can be attached to the different rims of pillararenes to make non-symmetrical pillararenes, which was reported by Huang and co-workers, leading to novel building blocks in fabricating macrocyclic amphiphiles.87d Therefore, PA[n]s, especially WP[n]s, have been widely used in supramolecular chemistry, and their applications in molecular recognition, self-assemblies, supramolecular polymers and stimuli-responsive systems have been well investigated.90
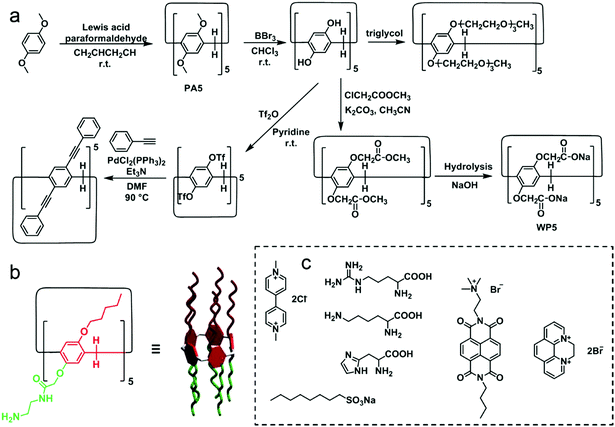 |
| Fig. 21 (a) Synthetic procedures and chemical structures of typical pillar[5]arene derivatives. (b) Chemical structure of a reported non-symmetrical pillararene. (c) Typical guests for water-soluble pillararenes. | |
5.1 Pillar[n]arene-based stimuli-responsive supra-amphiphiles in controlled release and ion sensing
WP[n]s have pH-responsive characteristics. For example, water-soluble pillar[5]arene (WP5) with carboxylate groups is soluble in water in neutral or base conditions. However, carboxylic acid moieties form along with the precipitation of pillar[5]arene in acid conditions.91 Besides, the chelation between the carboxylate anion and a metal cation (such as the zinc cation) also hampers the solubility of WP5. These properties are commonly used in stimuli-responsive supra-amphiphiles. Based on these points, Du et al. reported a controlled release system based on a supra-amphiphile that responded to five stimuli, including GSH, the zinc cation, pH, CO2 and hexanediamine (HDA).92 Benzimidazolium containing amphiphile 26 could be associated by WP6, and the supra-amphiphile WP6/26 self-assembled into vesicles in water (Fig. 22). A water-soluble dye, Ru(bipy)3Cl2, was chosen as a model cargo in the vesicles to estimate the controlled release ability. The redox-responsiveness was fulfilled by GSH, which cleaved the disulfide linker in the guest molecule, resulting in the loss of amphiphilicity of the host–guest complex and leading to the disruption of vesicles and cargo release. Cation-responsiveness was achieved by adjusting the pH to 5.0 or adding zinc cations to decrease the negative charges on WP6 and weaken the electrostatic interaction, leading to the guest threading out of the cavity. The disassociation could also be observed by bubbling with CO2. As a competitive guest, HDA was able to destroy the supra-amphiphile and release the cargo. Due to the overexpression of GSH and higher acidity in tumor cells, DOX-loaded WP6/29 vesicles were applied in cancer therapy. Indeed, the encapsulated DOX was successfully released and entered the cell nuclei to induce cell apoptosis. This is the first report that the self-assembled behaviour of the “smart” supra-amphiphile can be altered by five stimuli, and the stimuli involved are related to the tumor microenvironments, which makes the stimuli-responsive supra-amphiphile vesicles have promising applications in tumor therapy with diverse requirements. The multi-responsive property is a crucial advantage of the pillararene-based supra-amphiphile.
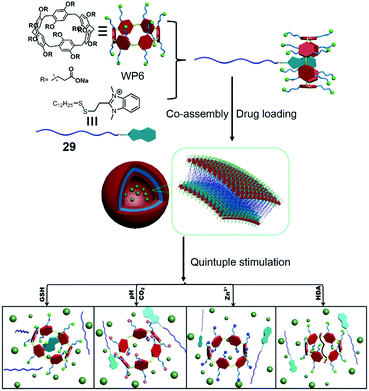 |
| Fig. 22 Chemical structure of a multi-responsive pillar[6]arene-based supra-amphiphile and a schematic representation of its controlled release process. Adapted with permission from ref. 92. Copyright 2017 John Wiley and Sons. | |
Molecule sensing is an important application of stimuli-responsive systems except for controlled release. Detection of heavy metal ions which cause severe pollution and toxicity to biological systems is very important.93 Many traditional metal ion sensors suffer from the requirements of organic solvents that restrict their environmental applications. This problem can be solved by using WP[n]-based supramolecular systems, which have been widely employed in ion detection and recognition.94 Recently, a Fe3+ ion sensor based on WP5 and its host–guest system was reported by Yin and co-workers (Fig. 23).95 Perylenediimide (PDI), a common fluorophore in fluorescent probes, was applied to detect Fe3+ ions. The host–guest system was constructed by a water-soluble ammonium modified PDI derivative 30 and WP5. The self-assembly morphology of the amphiphilic system changed from irregular aggregates to regular blocks along with a fluorescence “turn off” by PET in the presence of WP5. Notably, by testing the effects of various metal ions, only Fe3+ ions caused the fluorescence “turn on”. Further study showed that Fe3+ ions had strong interactions with WP5/30, and the interdiction of the PET process was the dominant reason for fluorescent recovery. The reversibility of this fluorescence sensor was measured by alternately adding Fe3+ ions and competitive chelator Na4P2O7; the sensor showed reversible fluorescent changes. The pillararene-based supra-amphiphile renders the system simple synthesis and reversibility. Moreover, this Fe3+ ion sensor exhibits a specific response to Fe3+ ions with a detection limit of 2.13 × 10−7 M, which demonstrates the promising applications of pillararenes in environmental or biological monitoring.
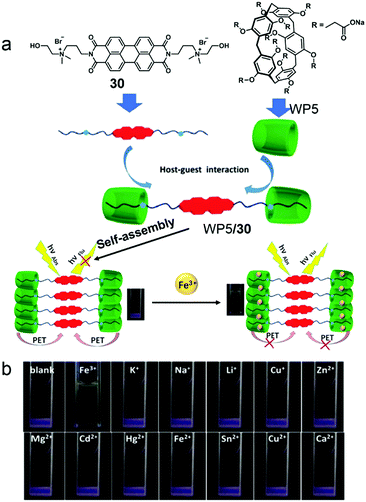 |
| Fig. 23 (a) Chemical structures of the host and guest compounds and a cartoon representation of the Fe3+ ion sensing mechanism. (b) Photographs of WP5/30 supra-amphiphile solutions after adding various metal ions under UV light. Adapted with permission from ref. 95. Copyright 2017 American Chemical Society. | |
5.3 Pillar[n]arene-based supra-amphiphiles for drug delivery
Supramolecular drug delivery systems for chemotherapy are a feasible approach to enhance the specificity and efficacy of therapeutic drugs. Compared with controlled release, living cell drug delivery is more complicated because of the multi-component bio-system. Although facing challenges, lots of drug delivery systems based on pillararenes have been reported typically by using supra-amphiphiles.96 As synthetic macrocycles, the biocompatibility of pillararenes is an important issue in living cell experiments. To solve this problem, Wang et al. synthesized biocompatible water-soluble phosphate pillar[5,6]arenes (WP5P and WP6P) and applied them in drug delivery (Fig. 24).97 To fabricate supra-amphiphilic systems, a functionalized pyridinium guest 31 was applied as a surfactant. The studies of host–guest recognition suggested that WP5P and WP6P had strong affinities to cationic guests, which were similar to the properties of WP5 and WP6. Surprisingly, supra-amphiphile WP5P/31 self-assembled into micelles in water, whereas WP6P/31 formed vesicles. The different morphologies were used as nanocarriers for different drugs. Hydrophobic drug DOX could be encapsulated by WP5P/31 micelles, and hydrophilic drug mitoxantrone (MTZ) preferred the hollow structures of WP6P/31 vesicles. Both of the drug-loaded supramolecular nanocarriers showed acid- and Zn2+-responses in vitro, leading to effective drug release. As expected, compared with the cells incubated with carboxylate-containing WP5 and WP6, the cell viabilities of WP5P and WP6P treated L929 cells were always higher, indicating the introduction of phosphate effectively ameliorated the biocompatibility. The drug delivery abilities of both supra-amphiphiles were estimated. The results confirmed the relative cell viability of normal cells was higher than that of the tumor cells resulting from the effective drug accumulation in tumor cells.
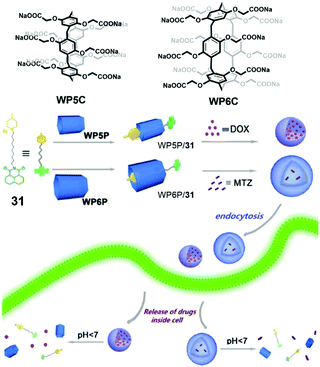 |
| Fig. 24 Chemical structures of WP5P, WP6P and pyridinium guest 31, and a cartoon representation of the self-assembling and controlled release processes. Adapted with permission from ref. 97. Copyright 2016 American Chemical Society. | |
Pei et al. reported a galactose-containing self-assembled nanocarrier which enhanced the cellular uptake of nanocarriers as well as the effect of DOX for HepG2 cells overexpressing asialoglycoprotein receptor (ASGP-R) (Fig. 25).98 Protonated tryptophan (Trp) unit functionalized PA5 (TP5) and a galactose-containing pyridinium guest (32) self-assembled into vesicles with galactose external layers in water, and the vesicles showed efficient encapsulation for DOX. The galactose on the vesicle surfaces acted as a targeting group to HepG2 cells via carbohydrate–protein interactions. Moreover, the protonated Trp units on TP5 had significant interactions with DNA, which promoted the formation of a TP5/DNA/DOX complex. The inhibition of DNA synthesis eventually caused enhanced toxicity for cell death. Compared with free DOX, the DOX loaded vesicles also showed enhanced toxicity to hepatoma cells and DOX-resistant hepatoma cells, indicating that the rational design of TP5/32 nanocarriers played indispensable roles in this highly efficient chemotherapy.
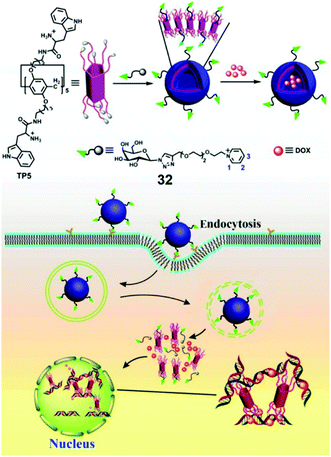 |
| Fig. 25 Chemical structures of TP5 and guest 32, and the cartoon representation of the mechanism of the enhanced anti-cancer effect of DOX loaded TP5/32 vesicles. Adapted with permission from ref. 98. Copyright 2016 American Chemical Society. | |
These two pioneering examples pointed out the advantages of pillararene-based self-assemblies in biochemical applications. The easy-to-functionalize characteristic of pillararenes endows the hosts with diversity and multiple merits such as biocompatibility and stimuli-responsiveness. The host–guest interactions benefit the introduction of functional guests, for example, targeting groups, diagnostic/imaging agents, and therapeutics. The reversibility and stimuli-responsiveness of the non-covalent interactions are usually used to control the self-assembly behaviour, which further controls their bio-applications.
5.3 Pillar[n]arene-based supra-amphiphiles for biological imaging
Fluorescent self-assembled materials have been a research hotspot in recent years because of their attractive performances in imaging, sensing, energy conversion and organic optical materials.99 In contrast with traditional fluorescent materials, supramolecular amphiphile involving self-assemblies have better flexibility and easier synthesis. Therefore, using host–guest recognitions and self-assemblies in constructing fluorescent materials is a novel approach. Among numerous fluorescent systems, near-infrared (NIR) emission is considered as an essential function because of its wide applications in biological imaging.41c,100 In order to broaden the biochemical applications of pillararenes, Huang et al. reported self-assembled NPs with NIR emission based on WP5 and a NIR fluorophore for cell imaging (Fig. 26).101 Cyanostilbene derivatives are known to absorb visible light and emit red to NIR fluorescence, and their emission could be enhanced by formation of an aggregated state. Therefore, cyanostilbene derivative 33 with aggregation-induced emission (AIE) and NIR emission was modified with a cationic group as a guest of WP5. The water solution of 33 had nearly no emission, and the aggregated nanoribbons showed a slight fluorescent enhancement. Notably, the formation of the host–guest complex significantly increased the NIR emission, which was explained by host–guest complexation enhanced aggregation. The morphology self-assembled by supra-amphiphilic WP5/33 was NPs, which showed pH-response due to the precipitation of WP5 in acid conditions. Considering that the excitation and emission wavelengths exhibited low photo-damage to biological samples, these NIR nanoparticles were further used in cell imaging. After incubating with WP5/33 nanoparticles, bright red fluorescence was observed in the cytoplasm of Hela and bEnd.3 cells. These results indicated the NIR nanoparticles were successfully applied in cell imaging and pillararene-based host–guest complexes could have enormous potential in functional fluorescent materials.
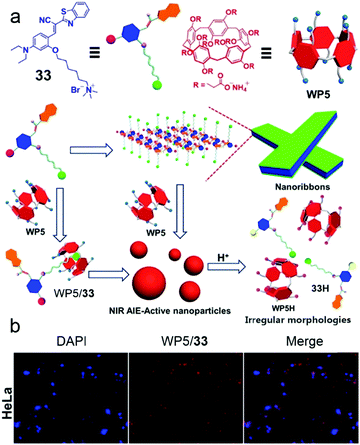 |
| Fig. 26 (a) Schematic representation of self-assembly processes of 33 and WP5/33. (b) CLSM images of HeLa cells demonstrating the cell image capability of the WP5/33 supra-amphiphile. Adapted with permission from ref. 101. Copyright 2016 American Chemical Society. | |
Photoacoustic imaging provides unique advantages in monitoring diseases by detection of ultrasonic waves generated by thermo-elastic expansion.102 It's capable of acquiring high-resolution images with deep depth. The contrast, which is urgently required for high-performance photoacoustic imaging, is usually determined by the optical-to-acoustic efficiency, for example, the conversion rate of absorbed laser energy to the thermoacoustic wave.103 Therefore, amplifying the photoacoustic signal is demanded in photoacoustic imaging. It's known that graphene oxide (GO) has been extended to biomedical applications but has rarely been used for photoacoustic imaging due to its structural defects introduced by the oxidative process and its poor absorption in the NIR region.104 To solve these problems, Chen et al. reported a supramolecular hybrid material for ultrasound and photoacoustic amplification based on a pillararene and GO (Fig. 27).105 A supra-amphiphile combining pillar[6]arene (CP6) with bicarbonate counterions and a pyrene-containing guest (34) was constructed, and its hydrophobic pyrene group could attach onto the surfaces of GO via π–π stacking. This hybrid material exhibited some different properties compared with native GO such as enhanced NIR absorption, which was favourable for the photothermal effect. Furthermore, the hybrid material was applied as a heating source to decompose the bicarbonate counterions to generate CO2 nanobubbles. These nanobubbles were capable of reflecting and scattering ultrasound, and increasing the vibration of the medium, rendering the hybrid material a contrast agent in ultrasonography and a nanoamplifier to enhance the photoacoustic signal. The hybrid material was also applied in in vivo experiments using tumor-bearing nude mice, and the photoacoustic signal of the tumors treated with the material increased significantly. In this work, the introduction of the supra-amphiphile changed the photothermal capacity of GO, which was indispensable in the enhancement of the photoacoustic signal. It also showed the diversity of pillararenes. As a good example of a pillararene-containing hybrid material, we believe there will be more emerging smart materials applied in biological or optical fields.
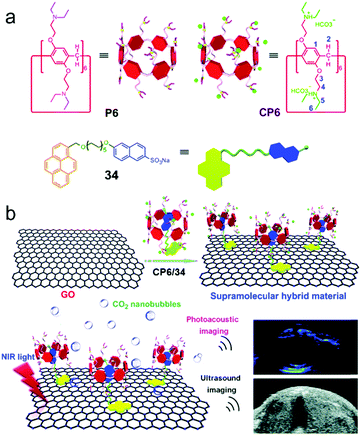 |
| Fig. 27 (a) Chemical structures of CP6 and 34. (b) Cartoon representation of the hybrid material and its photoacoustic amplification ability. Adapted with permission from ref. 105. Copyright 2018 Royal Society of Chemistry. | |
6. Summary and outlook
In this review, we summarize the recent progress in the field of macrocycle-based amphiphiles. Numerous self-assemblies or materials with interesting morphologies and functions have been developed from these amphiphiles. The introduction of macrocyclic hosts brings fantastic advantages to the functional materials constructed from macrocycle-based amphiphiles. First, the host or guest molecules are easy to be functionalized and synthesized, which further influences the functions of self-assemblies or materials to meet different requirements, such as targeting group modified surfaces of self-assemblies for enhanced cellular uptake and fluorescent group modified self-assemblies for sensing. Second, the morphologies of self-assemblies could be easily controlled. For example, macrocyclic amphiphiles are reliable building blocks to form vesicles, and the self-assembled vesicles can be further used as models for functional modifications. Third, the dynamic nature of non-covalent interactions endows macrocyclic host-based supramolecular amphiphiles with multiple stimuli-responsiveness. For example, the host–guest complexation can be adjusted by guest competition, heating, pH changing and other external stimuli, and the morphologies of self-assemblies or the functions of the systems can be easily tailored. By integrating these advantages, many complex systems with unique properties can be easily prepared. There is no doubt that macrocyclic host-based supramolecular amphiphiles have become a rising star in various fields.
Despite numerous detailed studies on macrocycle-based amphiphiles, challenges such as well-defined self-assembly structures, predictable self-assembly morphologies, and accurate building block arrangements in self-assemblies, are still remaining. Perhaps studies on how the building blocks arrange themselves and reliable mechanisms in the self-assembly process can help us to realize precise or predictable self-assembly. Besides, with the dynamic nature of macrocyclic host-based supramolecular amphiphiles, the self-assemblies are rendered stimuli-responsiveness. To broaden the applications of these stimuli-responsive systems, many attempts such as combining macrocyclic host-based supramolecular amphiphiles with polymers, inorganic materials and other hybrid materials have become a research hotspot recently. The development of novel and multi-functional materials with macrocycle-based amphiphiles might be a flourishing area in the future.
Conflicts of interest
There are no conflicts to declare.
Acknowledgements
The National Natural Science Foundation of China (91527301, 21434005) is acknowledged for financial support.
Notes and references
-
(a) S. Biswas, K. Kinbara, T. Niwa, H. Taguchi, N. Ishii, S. Watanabe, K. Miyata, K. Kataoka and T. Aida, Nat. Chem., 2013, 5, 613 CrossRef CAS PubMed;
(b) Q. Gan, Y. Ferrand, C. Bao, B. Kauffmann, A. Grélard, H. Jiang and I. Huc, Science, 2011, 331, 1172 CrossRef CAS PubMed;
(c) J. D. Hartgerink, E. Beniash and S. I. Stupp, Science, 2001, 294, 1684 CrossRef CAS PubMed;
(d) C. C. Lee, C. Grenier, E. W. Meijer and A. P. H. J. Schenning, Chem. Soc. Rev., 2009, 38, 671 RSC;
(e) W. Zhang, W. Jin, T. Fukushima, A. Saeki, S. Seki and T. Aida, Science, 2011, 334, 340 CrossRef CAS PubMed;
(f) Z. Huang, S.-K. Kang, M. Banno, T. Yamaguchi, D. Lee, C. Seok, E. Yashima and M. Lee, Science, 2012, 337, 1521 CrossRef CAS PubMed;
(g) F. Vera, J. L. Serrano and T. Sierra, Chem. Soc. Rev., 2009, 38, 781 RSC;
(h) P. Cordier, F. Tournilhac, C. Soulié-Ziakovic and L. Leibler, Nature, 2008, 451, 977 CrossRef CAS PubMed;
(i) T. Aida, E. W. Meijer and S. I. Stupp, Science, 2012, 335, 813 CrossRef CAS PubMed;
(j) F. Zeng and S. C. Zimmerman, Chem. Rev., 1997, 97, 1681 CrossRef CAS PubMed;
(k) R. Chakrabarty, P. S. Mukherjee and P. J. Stang, Chem. Rev., 2011, 111, 6810 CrossRef CAS PubMed;
(l) T. R. Cook and P. J. Stang, Chem. Rev., 2015, 115, 7001 CrossRef CAS PubMed.
-
(a) A. L. Horwich, G. W. Farr and W. A. Fenton, Chem. Rev., 2006, 106, 1917 CrossRef CAS PubMed;
(b) C. A. Royer, Chem. Rev., 2006, 106, 1769 CrossRef CAS PubMed;
(c) W. W. Grabow and L. Jaeger, Acc. Chem. Res., 2014, 47, 1871 CrossRef CAS PubMed;
(d) J. D. Flory, C. R. Simmons, S. Lin, T. Johnson, A. Andreoni, J. Zook, G. Ghirlanda, Y. Liu, H. Yan and P. Fromme, J. Am. Chem. Soc., 2014, 136, 8283 CrossRef CAS PubMed;
(e) C. Geary, S. Baudrey and L. Jaeger, Nucleic Acids Res., 2008, 36, 1138 CrossRef CAS PubMed;
(f) L. Jaeger and N. B. Leontis, Angew. Chem., Int. Ed., 2000, 14, 2521 CrossRef;
(g) A. Chworos, I. Severcan, A. Y. Koyfman, P. Weinkam, E. Oroudjev, H. G. Hansma and L. Jaeger, Science, 2004, 306, 2068 CrossRef CAS PubMed;
(h) J. Lee, W. Kladwang, M. Lee, D. Cantu, M. Azizyan, H. Kim, A. Limpaecher, S. Yoon, A. Treuille and R. Das, Proc. Natl. Acad. Sci. U. S. A., 2014, 111, 2122 CrossRef CAS PubMed;
(i) J. B. Lee, J. Hong, D. K. Bonner, Z. Poon and P. T. Hammond, Nat. Mater., 2012, 11, 316 CrossRef CAS PubMed.
-
(a) T. J. McIntosh and S. A. Simon, Annu. Rev. Biophys. Biomol. Struct., 1994, 23, 27 CrossRef CAS PubMed;
(b) S. Matile, A. V. Jentzsch, J. Montenegro and A. Fin, Chem. Soc. Rev., 2011, 40, 2453 RSC.
-
(a) J. N. Isrealachvili, D. J. Mitchell and B. W. Ninham, J. Chem. Soc., Faraday Trans. 2, 1976, 72, 1525 RSC;
(b) S. Zhang, R.-O. Moussodia, H.-J. Sun, P. Leowanawat, A. Muncan, C. D. Nusbaum, K. M. Chelling, P. A. Heiney, M. L. Klein, S. Andre, R. Roy, H.-J. Gabius and V. Percec, Angew. Chem., Int. Ed., 2014, 53, 10899 CrossRef CAS PubMed;
(c) A. Sorrenti, O. Illa and R. M. Ortuño, Chem. Soc. Rev., 2013, 42, 8200 RSC.
-
(a) H. Ringsdorf, B. Schlarb and J. Venzmer, Angew. Chem., Int. Ed., 1988, 27, 113 CrossRef;
(b) M. Wang, K. Alberti, S. Sun, C. L. Arellano and Q. Xu, Angew. Chem., Int. Ed., 2014, 53, 2893 CrossRef CAS PubMed;
(c) L.-P. Lv, K. Landfester and D. Crespy, Chem. Mater., 2014, 26, 3351 CrossRef CAS;
(d) E. Soussan, S. Cassel, M. Blanzat and I. Rico-Lattes, Angew. Chem., Int. Ed., 2009, 48, 274 CrossRef CAS PubMed;
(e) J. Yao, M. Yang and Y. Duan, Chem. Rev., 2014, 114, 6130 CrossRef CAS PubMed;
(f) E. G. Kelley, J. N. L. Albert, M. O. Sullivan and T. H. Epps III, Chem. Soc. Rev., 2013, 42, 7057 RSC;
(g) H. Zhu, L. Shangguan, D. Xia, J. H. Mondal and B. Shi, Nanoscale, 2017, 9, 8913 RSC.
- S. Fleming and R. V. Ulijn, Chem. Soc. Rev., 2014, 43, 8150 RSC.
-
(a) M. Moffitt, K. Khougaz and A. Eisenberg, Acc. Chem. Res., 1996, 29, 95 CrossRef CAS;
(b) Y. Mai and A. Eisenberg, Chem. Soc. Rev., 2012, 41, 5969 RSC;
(c)
P. Alexandridis and B. Lindman, Amphiphilic Block Copolymers: Self-Assembly and Applications, Elsevier, Amsterdam, 2000 Search PubMed.
-
(a) X. Wang, H. Chen, K. Zhang, M. Ma, F. Li, D. Zeng, S. Zheng, Y. Chen, L. Jiang, H. Xu and J. Shi, Small, 2014, 10, 1403 CrossRef CAS PubMed;
(b) J. Nicolas, S. Mura, D. Brambilla, N. Mackiewicz and P. Couvreur, Chem. Soc. Rev., 2013, 42, 1147 RSC;
(c) F. Yuen and K. C. Tam, Soft Matter, 2010, 6, 4613 RSC;
(d) C. Alvarez-Lorenzoa and A. Concheiro, Chem. Commun., 2014, 50, 7743 RSC.
-
(a) J.-M. Lehn, Angew. Chem., Int. Ed., 1988, 27, 89 CrossRef;
(b) D. J. Cram, Angew. Chem., Int. Ed., 1988, 27, 1009 CrossRef;
(c) C. J. Pedersen, Angew. Chem., Int. Ed., 1988, 27, 1021 CrossRef;
(d) J.-P. Sauvage, Angew. Chem., Int. Ed., 2017, 56, 11080 CrossRef CAS PubMed;
(e) J. F. Stoddart, Angew. Chem., Int. Ed., 2017, 56, 11094 CrossRef CAS PubMed;
(f) B. L. Feringa, Angew. Chem., Int. Ed., 2017, 56, 11060 CrossRef CAS PubMed.
-
(a) S. C. Zimmerman, M. S. Wendland, N. A. Rakow, I. Zharov and K. S. Suslick, Nature, 2002, 418, 399 CrossRef CAS PubMed;
(b) F. Huang, F. R. Fronczek and H. W. Gibson, J. Am. Chem. Soc., 2003, 125, 9272 CrossRef CAS PubMed;
(c) E. R. Kay, D. A. Leigh and F. Zerbetto, Angew. Chem., Int. Ed., 2007, 46, 72 CrossRef CAS PubMed;
(d) F. Rodler, J. Linders, T. Fenske, T. Rehm, C. Mayer and C. Schmuck, Angew. Chem., Int. Ed., 2010, 49, 8747 CrossRef CAS PubMed;
(e) F. Giacalone and N. Martin, Adv. Mater., 2010, 22, 4220 CrossRef CAS PubMed;
(f) H. Zhu, B. Shi, K. Chen, P. Wei, D. Xia, J. H. Mondal and F. Huang, Org. Lett., 2016, 18, 5054 CrossRef CAS PubMed.
-
(a) T. R. Cook, Y.-R. Zheng and P. J. Stang, Chem. Rev., 2013, 113, 734 CrossRef CAS PubMed;
(b) Y.-W. Yang, Y.-L. Sun and N. Song, Acc. Chem. Res., 2014, 47, 1950 CrossRef CAS PubMed;
(c) D.-W. Zhang, X. Zhao and Z.-T. Li, Acc. Chem. Res., 2014, 47, 1961 CrossRef CAS PubMed;
(d) M. Zhang, X. Yan, F. Huang, Z. Niu and H. W. Gibson, Acc. Chem. Res., 2014, 47, 1995 CrossRef CAS PubMed;
(e) H. Yang, B. Yuan, X. Zhang and O. A. Scherman, Acc. Chem. Res., 2014, 47, 2106 CrossRef CAS PubMed;
(f) J. Hu and S. Liu, Acc. Chem. Res., 2014, 47, 2084 CrossRef CAS PubMed.
- X. Chi, G. Yu, L. Shao, J. Chen and F. Huang, J. Am. Chem. Soc., 2016, 138, 3168 CrossRef CAS PubMed.
- Y. Yao, X. Chi, Y. Zhou and F. Huang, Chem. Sci., 2014, 5, 2778 RSC.
- Y. Zhou, K. Jie and F. Huang, Org. Chem. Front., 2017, 4, 2387 RSC.
- J.-F. Chen, Q. Lin, Y.-M. Zhang, H. Yao and T.-B. Wei, Chem. Commun., 2017, 53, 13296 RSC.
-
(a) G. Yu, K. Jie and F. Huang, Chem. Rev., 2015, 115, 7240 CrossRef CAS PubMed;
(b) K. Jie, Y. Zhou, Y. Yao and F. Huang, Chem. Soc. Rev., 2015, 44, 3568 RSC.
-
(a) P. Xin, S. Tan, Y. Sun, Q. Ren, W. Dong, J. Guo, T. Jiang and C.-P. Chen, Chem. Commun., 2017, 53, 5322 RSC;
(b) E. Amirthalingam, M. Rodrigues, L. Casal-Dujat, A. C. Calpena, D. B. Amabilino, D. Ramos-López and L. Pérez-García, J. Colloid Interface Sci., 2015, 437, 132 CrossRef CAS PubMed;
(c) K. Helttunen, A. Galán, P. Ballester, J. Bergenholtz and M. Nissinen, J. Colloid Interface Sci., 2016, 464, 59 CrossRef CAS PubMed.
-
(a) J. Szejtli, Chem. Rev., 1998, 98, 1743 CrossRef CAS PubMed;
(b) G. Wenz, B.-H. Han and A. Müller, Chem. Rev., 2006, 106, 782 CrossRef CAS PubMed;
(c) A. Harada, Acc. Chem. Res., 2001, 34, 456 CrossRef CAS PubMed;
(d) K. A. Connors, Chem. Rev., 1997, 97, 1325 CrossRef CAS PubMed;
(e) K. Uekama, F. Hirayama and T. Irie, Chem. Rev., 1998, 98, 2045 CrossRef CAS PubMed;
(f) A. Douhal, Chem. Rev., 2004, 104, 1955 CrossRef CAS PubMed;
(g) G. Crini, Chem. Rev., 2014, 114, 10940 CrossRef CAS PubMed.
-
(a) L. Wang, L. Li, Y. Fan and H. Wang, Adv. Mater., 2013, 25, 3888 CrossRef CAS PubMed;
(b) S. A. Nepogodiev and J. F. Stoddart, Chem. Rev., 1998, 98, 1959 CrossRef CAS PubMed;
(c) A. Harada, A. Hashidzume, H. Yamaguchi and Y. Takashima, Chem. Rev., 2009, 109, 5974 CrossRef CAS PubMed;
(d) Q.-D. Hu, G.-P. Tang and P. K. Chu, Acc. Chem. Res., 2014, 47, 2017 CrossRef CAS PubMed;
(e) M. W. Ambrogio, C. R. Thomas, Y. L. Zhao, J. I. Zink and J. F. Stoddartt, Acc. Chem. Res., 2011, 44, 903 CrossRef CAS PubMed;
(f) H. Wang, S. T. Wang, H. Su, K. J. Chen, A. L. Armijo, W. Y. Lin, Y. J. Wang, J. Sun, K. Kamei, J. Czernin, C. G. Radu and H. R. Tseng, Angew. Chem., Int. Ed., 2009, 48, 4344 CrossRef CAS PubMed;
(g) Q. Hu, W. Li, X. Hu, Q. Hu, J. Shen, X. Jin, J. Zhou, G.-P. Tang and P. K. Chu, Biomaterials, 2012, 33, 6580 CrossRef CAS PubMed;
(h) R. Dong, L. Zhou, J. Wu, C. Tu, Y. Su, B. Zhu, H. Gu, D. Yan and X. Zhu, Chem. Commun., 2011, 47, 5473 RSC;
(i) Y. Zhao, F. Sakai, L. Su, Y. Liu, K. Wei, G. Chen and M. Jiang, Adv. Mater., 2013, 25, 5215 CrossRef CAS PubMed;
(j) K. Wei, J. Li, G. Chen and M. Jiang, ACS Macro Lett., 2013, 2, 278 CrossRef CAS;
(k) H. Kitagishi, M. Tamaki, T. Ueda, S. Hirota, T. Ohta, Y. Naruta and K. Kano, J. Am. Chem. Soc., 2010, 132, 16730 CrossRef CAS PubMed;
(l) F. Sakai, G.-S. Chen and M. Jiang, Polym. Chem., 2012, 3, 954 RSC;
(m) A. J. Valente and O. Söderman, Adv. Colloid Interface Sci., 2014, 205, 156 CrossRef CAS PubMed;
(n) M. Messner, S. V. Kurkov, P. Jansook and T. Loftsson, Int. J. Pharm., 2010, 387, 199 CrossRef CAS PubMed.
-
(a) X. Huan, D. Wang, R. Dong, C. Tu, B. Zhu, D. Yan and X. Zhu, Macromolecules, 2012, 45, 5941 CrossRef CAS;
(b) A. Harada, R. Kobayashi, Y. Takashima, A. Hashidzume and H. Yamaguchi, Nat. Chem., 2011, 3, 34 CrossRef CAS PubMed;
(c) Y. Liu, G.-S. Chen, Y. Chen, F. Ding and J. Chen, Org. Biomol. Chem., 2005, 3, 2519 RSC;
(d) C. Fleischmann and H. Ritter, Macromol. Rapid Commun., 2013, 34, 1085 CrossRef CAS PubMed;
(e) J. J. Gassensmith, J. Y. Kim, J. M. Holcroft, O. K. Farha, J. F. Stoddart, J. T. Hupp and N. C. Jeong, J. Am. Chem. Soc., 2014, 136, 8277 CrossRef CAS PubMed;
(f) E. M. Del Valle, Process Biochem., 2004, 39, 1033 CrossRef;
(g) J. Zhang and P.-X. Ma, Adv. Drug Delivery Rev., 2013, 65, 1215 CrossRef CAS PubMed.
-
(a) G.-S. Chen and M. Jiang, Chem. Soc. Rev., 2011, 40, 2254 RSC;
(b) K. N. Houk, A. G. Leach, S. P. Kim and X. Zhang, Angew. Chem., Int. Ed., 2003, 42, 4872 CrossRef CAS PubMed.
-
(a) A. Okamatsu, K. Motoyama, R. Onodera, T. Higashi, T. Koshigoe, Y. Shimada, K. Hattori, T. Takeuchi and H. Arima, Bioconjugate Chem., 2013, 24, 724 CrossRef CAS PubMed;
(b) E. M. A. Moncayo, N. Guilloteau, C. Bienvenu, J. L. J. Blanco, C. D. Giorgio, P. Vierling, J. M. Benito, C. O. Mellet and J. M. G. Fernàndez, New J. Chem., 2014, 38, 5215 RSC;
(c) T. Harada, L. Giorgio, T. J. Harris, D. T. Pham, H. T. Ngo, E. F. Need, B. J. Coventry, S. F. Lincoln, C. J. Easton, G. Buchanan and T. W. Kee, Mol. Pharmaceutics, 2013, 10, 4481 CrossRef CAS PubMed;
(d) M. Ma, S. Xu, P. Xing, S. Li, X. Chu and A. Hao, Colloid Polym. Sci., 2015, 293, 891 CrossRef CAS;
(e) R. Tong and J. Cheng, Angew. Chem., 2008, 120, 4908 CrossRef;
(f) M. Hörning, M. Nakahata, P. Linke, A. Yamamoto, M. Veschgini, S. Kaufmann, Y. Takashima, A. Harada and M. Tanaka, Sci. Rep., 2017, 7, 7660 CrossRef PubMed.
-
(a) X. Hu and X. Jing, Expert Opin. Drug Delivery, 2009, 6, 1079 CrossRef CAS PubMed;
(b) X. Hu, J. Li, W. Lin, Y. Huang, X. Jing and Z. Xie, RSC Adv., 2014, 4, 38405 RSC;
(c) R. Lin, A. G. Cheetham, P. Zhang, Y. A. Lin and H. Cui, Chem. Commun., 2013, 49, 4968 RSC.
-
(a) D. Peer, J. M. Karp, S. Hong, O. C. Farokhzad, R. Margalit and R. Langer, Nat. Nanotechnol., 2007, 2, 751 CrossRef CAS PubMed;
(b) F. Danhier, O. Feron and V. Preat, J. Controlled Release, 2010, 148, 135 CrossRef CAS PubMed;
(c) T. M. Zagar, D. M. Cardinale and L. B. Marks, Nat. Rev. Clin. Oncol., 2015, 13, 172 CrossRef PubMed.
-
(a) V. P. Torchilin, Nat. Rev. Drug Discovery, 2005, 4, 145 CrossRef CAS PubMed;
(b) F. E. Alemdaroglu, N. C. Alemdaroglu, P. Langguth and A. Herrmann, Adv. Mater., 2008, 20, 899 CrossRef CAS;
(c) C. C. Lee, J. A. MacKay, J. M. Frechet and F. C. Szoka, Nat. Biotechnol., 2005, 23, 1517 CrossRef CAS PubMed;
(d) G. F. Paciotti, D. G. I. Kingston and L. Tamarkin, Drug Dev. Res., 2006, 67, 47 CrossRef CAS;
(e) M. Joglekar and B. G. Trewyn, Biotechnol. J., 2013, 8, 931 CrossRef CAS PubMed.
- Q. Pei, X. Hu, L. Wang, S. Liu, X. Jing and Z. Xie, ACS Appl. Mater. Interfaces, 2017, 9, 26740 CrossRef CAS PubMed.
- Q. Pei, X. Hu, S. Liu, Y. Li, Z. Xie and X. Jing, J. Controlled Release, 2017, 254, 23 CrossRef CAS PubMed.
-
(a) Z. Zhang, D. S. Kim, C.-Y. Lin, H. Zhang, A. D. Lammer, V. M. Lynch, I. Popov, O. Š. Miljanić, E. V. Anslyn and J. L. Sessler, J. Am. Chem. Soc., 2015, 137, 7769 CrossRef CAS PubMed;
(b) P. Wang, Y. Yao and M. Xue, Chem. Commun., 2014, 50, 5064 RSC;
(c) X. Ma and Y. Zhao, Chem. Rev., 2015, 115, 7794 CrossRef CAS PubMed;
(d) X. Wu, Y. Li, C. Lin, X. Hu and L. Wang, Chem. Commun., 2015, 51, 6832 RSC;
(e) C. D. Jones and J. W. Steed, Chem. Soc. Rev., 2016, 45, 6546 RSC;
(f) N. Tuccitto, G. Sfrazzetto, T. Giuseppe, C. M. A. Gangemi, F. P. Ballistreri, R. M. Toscano, G. A. Tomaselli, A. Pappalardo and G. Marletta, Chem. Commun., 2016, 52, 11681 RSC;
(g) X. Ji, S. Dong, P. Wei, D. Xia and F. Huang, Adv. Mater., 2013, 25, 5725 CrossRef CAS PubMed;
(h) M. Li, M. R. Stojković, M. Ehlers, E. Zellermann, I. Piantanida and C. Schmuck, Angew. Chem., Int. Ed., 2016, 55, 13015 CrossRef CAS PubMed;
(i) J. Bi, X. Zeng, D. Tian and H. Li, Org. Lett., 2016, 18, 1092 CrossRef CAS PubMed;
(j) Y. Tu, F. Peng, X. Sui, Y. Men, P. B. White, J. C. M. van Hest and D. A. Wilson, Nat. Chem., 2016, 9, 480 CrossRef PubMed;
(k) X. Yan, J.-F. Xu, T. R. Cook, F. Huang, Q.-Z. Yang, C.-H. Tung and P. J. Stang, Proc. Natl. Acad. Sci. U. S. A., 2014, 111, 8717 CrossRef CAS PubMed;
(l) N. Koumura, R. W. J. Zijlstra, R. A. van Delden, N. Harada and B. L. Feringa, Nature, 1999, 401, 152 CrossRef CAS PubMed;
(m) G. Yu, C. Han, Z. Zhang, J. Chen, X. Yan, B. Zheng, S. Liu and F. Huang, J. Am. Chem. Soc., 2012, 134, 8711 CrossRef CAS PubMed;
(n) N. H. Evans and P. D. Beer, Angew. Chem., Int. Ed., 2014, 53, 11716 CrossRef CAS PubMed;
(o) D. Cao, M. Amelia, L. M. Klivansky, G. Koshkakaryan, S. I. Khan, M. Semeraro, S. Silvi, M. Venturi, A. Credi and Y. Liu, J. Am. Chem. Soc., 2010, 132, 1110 CrossRef CAS PubMed;
(p) Y. Chang, K. Yang, P. Wei, S. Huang, Y. Pei, W. Zhao and Z. Pei, Angew. Chem., Int. Ed., 2014, 53, 13126 CrossRef CAS PubMed.
- E. Morales-Narváez, L. Baptista-Pires, A. Zamora-Gálvez and A. Merkoçi, Adv. Mater., 2017, 29, 1604905 CrossRef PubMed.
- R. V. Ulijn, J. Mater. Chem., 2006, 16, 2217 RSC.
- Y. Kang, Z. Cai, X. Tang, K. Liu, G. Wang and X. Zhang, ACS Appl. Mater. Interfaces, 2016, 8, 4927 CrossRef CAS PubMed.
-
(a) M. S. Timmer, B. L. Stocker and P. H. Seeberger, Curr. Opin. Chem. Biol., 2007, 11, 59 CrossRef CAS PubMed;
(b) G. Alvarez-Manilla, N. L. Warren, T. Abney, J. Atwood, III, P. Azadi, W. S. York, M. Pierce and R. Orlando, Glycobiology, 2007, 17, 677 CrossRef CAS PubMed;
(c) A. Varki, Nature, 2007, 446, 1023 CrossRef CAS PubMed;
(d) R. Kannagi, M. Izawa, T. Koike, K. Miyazaki and N. Kimura, Cancer Sci., 2004, 95, 377 CrossRef CAS PubMed;
(e) R. C. Casey, T. R. Oegema Jr., K. M. Skubitz, S. E. Pambuccian, S. M. Grindle and A. P. N. Skubitz, Clin. Exp. Metastasis, 2003, 20, 143 CrossRef CAS PubMed;
(f) J. B. Catterall, L. M. H. Jones and G. A. Turner, Clin. Exp. Metastasis, 1999, 17, 583 CrossRef CAS PubMed;
(g) R. Takano, E. Muchmore and J. W-Dennis, Glycobiology, 1994, 4, 665 CrossRef CAS PubMed;
(h) E. Pasmatzi, G. Badavanis, A. Monastirli, S. Georgiou, A. Sagriotis, Th. Sakkis, S. Mantagos, J. Varakis, G. Stamatiou and D. Tsambaos, Anat. Embryol., 2005, 209, 207 CrossRef CAS PubMed.
- S. Himmelein and B. J. Ravoo, Chem. – Eur. J., 2017, 23, 6034 CrossRef CAS PubMed.
-
(a) B. J. Ravoo and R. Darcy, Angew. Chem., Int. Ed., 2000, 39, 4324 CrossRef CAS;
(b) F. Versluis, J. Voskuhl, J. Vos, H. Friedrich, B. J. Ravoo, P. H. H. Bomans, M. C. A. Stuart, N. A. J. M. Sommerdijk and A. Kros, Soft Matter, 2014, 10, 9746 RSC.
-
(a) D. E. J. G. J. Dolmans, D. Fukumura and R. K. Jain, Nat. Rev. Cancer, 2003, 3, 380 CrossRef CAS PubMed;
(b) D. K. Chatterjee, L. S. Fong and Y. Zhang, Adv. Drug Delivery Rev., 2008, 60, 1627 CrossRef CAS PubMed;
(c) L. Cheng, C. Wang, L. Feng, K. Yang and Z. Liu, Chem. Rev., 2014, 114, 10869 CrossRef CAS PubMed.
-
(a) G. Szakács, J. K. Paterson, J. A. Ludwig, C. Booth-Genthe and M. M. Gottesman, Nat. Rev. Drug Discovery, 2006, 5, 219 CrossRef PubMed;
(b) C. Bock and T. Lengauer, Nat. Rev. Cancer, 2012, 12, 494 CrossRef CAS PubMed.
-
(a) M. Ethirajan, Y. Chen, P. Joshi and R. K. Pandey, Chem. Soc. Rev., 2011, 40, 34 RSC;
(b) Y. Cheng, A. C. Samia, J. D. Meyers, I. Panagopoulos, B. Fei and C. Burda, J. Am. Chem. Soc., 2008, 130, 10643 CrossRef CAS PubMed;
(c) Z. Z. Yu, Q. Q. Sun, W. Pan, N. Li and B. Tang, ACS Nano, 2015, 9, 11064 CrossRef CAS PubMed.
-
(a) A. Ormond and H. Freeman, Materials, 2013, 6, 817 CrossRef CAS PubMed;
(b) R. R. Allison and C. H. Sibata, Photodiagn. Photodyn. Ther., 2010, 7, 61 CrossRef CAS PubMed;
(c) J. F. Lovell, T. W. B. Liu, J. Chen and G. Zheng, Chem. Rev., 2010, 110, 2839 CrossRef CAS PubMed;
(d) C.-K. Lim, J. Heo, S. Shin, K. Jeong, Y. H. Seo, W. D. Jang, C. R. Park, S. Y. Park, S. Kim and I. C. Kwon, Cancer Lett., 2013, 334, 176 CrossRef CAS PubMed;
(e) A. Z. Wang, R. Langer and O.
C. Farokhzad, Annu. Rev. Med., 2012, 63, 185 CrossRef CAS PubMed.
- J. F. Lovell, C. S. Jin, E. Huynh, H. L. Jin, C. Kim, J. L. Rubinstein, W. C. W. Chan, W. Cao, L. V. Wang and G. Zheng, Nat. Mater., 2011, 10, 324 CrossRef CAS PubMed.
- H. Xiong, D. Zhou, X. Zheng, Y. Qi, Y. Wang, X. Jing and Y. Huang, Chem. Commun., 2017, 53, 3422 RSC.
-
(a) X. Michalet, F. F. Pinaud, L. A. Bentolila, J. M. Tsay, S. Doose, J. J. Li, G. Sundaresan, A. M. Wu, S. S. Gambhir and S. Weiss, Science, 2005, 307, 538 CrossRef CAS PubMed;
(b) I. L. Medintz, H. T. Uyeda, E. R. Goldman and H. Mattoussi, Nat. Mater., 2005, 4, 435 CrossRef CAS PubMed;
(c) R. Weissleder and M. J. Pittet, Nature, 2008, 452, 580 CrossRef CAS PubMed;
(d) M. Dahan, S. Levi, C. Luccardini, P. Rostaing, B. Riveau and A. Triller, Science, 2003, 302, 442 CrossRef CAS PubMed;
(e) F. Pinaud, S. Clarke, A. Sittner and M. Dahan, Nat. Methods, 2010, 7, 275 CrossRef CAS PubMed;
(f) S. N. Baker and G. A. Baker, Angew. Chem., Int. Ed., 2010, 49, 6726 CrossRef CAS PubMed.
-
(a) O. Mongin, T. R. Krishna, M. H. V. Werts, A.-M. Caminade, J.-P. Majoral and M. Blanchard-Desce, Chem. Commun., 2006, 915 RSC;
(b) O. Mongin, A. Pla-Quintana, F. Terenziani, D. Drouin, C. Le Droumaguet, A.-M. Caminade, J.-P. Majoral and M. Blanchard-Desce, New J. Chem., 2007, 31, 1354 RSC;
(c) C. Wu and D. T. Chiu, Angew. Chem., Int. Ed., 2013, 52, 3086 CrossRef CAS PubMed;
(d) J. Pecher and S. Mecking, Chem. Rev., 2010, 110, 6260 CrossRef CAS PubMed.
-
(a) F. J. M. Hoeben, P. Jonkheijm, E. W. Meijer and A. P. H. J. Schenning, Chem. Rev., 2005, 105, 1491 CrossRef CAS PubMed;
(b) T. F. A. De Greef, M. M. J. Smulders, M. Wolffs, A. P. H. J. Schenning, R. P. Sijbesma and E. W. Meijer, Chem. Rev., 2009, 109, 5687 CrossRef CAS PubMed.
- S. Sreejith, N. V. Menon, Y. Wang, H. Joshi, S. Liu, K. C. Chong, Y. Kang, H. Sun and M. C. Stuparu, Mater. Chem. Front., 2017, 1, 831 RSC.
-
(a) R. Muthukrishnan and C. D. Gutsche, J. Org. Chem., 1979, 44, 3962 CrossRef CAS;
(b) C. D. Gutsche, B. Dhawan, K. H. No and R. Muthukrishnan, J. Am. Chem. Soc., 1981, 103, 3782 CrossRef CAS;
(c) M. C. Calama, P. Timmerman and D. N. Reinhoudt, Angew. Chem., Int. Ed., 2000, 39, 755 CrossRef CAS;
(d) D.-S. Guo and Y. Liu, Acc. Chem. Res., 2014, 47, 1925 CrossRef CAS PubMed;
(e) R. Kumar, Y. O. Lee, V. Bhalla, M. Kumar and J. S. Kim, Chem. Soc. Rev., 2014, 43, 4824 RSC;
(f) S. Sameni, C. Jeunesse, D. Matt and J. Harrowfield, Chem. Soc. Rev., 2009, 38, 2117 RSC;
(g) D.-S. Guo and Y. Liu, Chem. Soc. Rev., 2012, 41, 5907 RSC.
-
(a) L. Baldini, A. Casnati, F. Sansone and R. Ungaro, Chem. Soc. Rev., 2007, 36, 254 RSC;
(b) A. Casnati, F. Sansone and R. Ungaro, Acc. Chem. Res., 2003, 36, 246 CrossRef CAS PubMed.
-
(a) A. Dondoni and A. Marra, Chem. Rev., 2010, 110, 4949 CrossRef CAS PubMed;
(b) J. S. Kim and D. T. Quang, Chem. Rev., 2007, 107, 3780 CrossRef CAS PubMed;
(c) S. Mann, Nat. Mater., 2009, 8, 781 CrossRef CAS PubMed;
(d) S. Fujii, S. Yamada, S. Matsumoto, G. Kubo, K. Yoshida, E. Tabata, R. Miyake, Y. Sanada, I. Akiba, T. Okobira, N. Yagi, E. Mylonas, N. Ohta, H. Sekiguchi and K. Sakurai, Sci. Rep., 2017, 7, 44494 CrossRef CAS PubMed.
- M. A. Markowitz, R. Bielski and S. L. Regen, Langmuir, 1989, 5, 276 CrossRef CAS.
-
(a) M. Strobel, K. Kita-Tokarczyk, A. Taubert, C. Vebert, P. A. Heiney, M. Chami and W. Meier, Adv. Funct. Mater., 2006, 16, 252 CrossRef CAS;
(b) O. M. Martin and S. Mecozzi, Tetrahedron, 2007, 63, 5539 CrossRef CAS;
(c) E. J. Cho, J. K. Kang, W. S. Han and J. H. Jung, Langmuir, 2008, 24, 5229 CrossRef CAS PubMed;
(d) G. Gattuso, A. Notti, A. Pappalardo, S. Pappalardo, M. F. Parisi and F. Puntoriero, Tetrahedron Lett., 2013, 54, 188 CrossRef CAS;
(e) T. Auletta, B. Dordi, A. Mulder, A. Sartori, S. Onclin, C. M. Bruinink, M. Péter, C. A. Nijhuis, H. Beijleveld, H. Schönherr, G. J. Vancso, A. Casnati, R. Ungaro, B. J. Ravoo, J. Huskens and D. N. Reinhoudt, Angew. Chem., Int. Ed., 2004, 43, 369 CrossRef CAS PubMed;
(f) M. Makha, I. R. McKinnon and C. L. Raston, J. Chem. Soc., Perkin Trans. 2, 2002, 1801 RSC;
(g) C. Geraci, G. M. L. Consoli, G. Granata, E. Galante, A. Palmigiano, M. Pappalardo, S. D. D. Puma and A. Spadaro, Bioconjugate Chem., 2013, 24, 1710 CrossRef CAS PubMed;
(h) E. James, P. K. Eggers, A. R. Harvey, S. A. Dunlop, M. Fitzgerald, K. A. Stubbs and C. L. Raston, Org. Biomol. Chem., 2013, 11, 6108 RSC.
- M. Komiyama, K. Isaka and S. Shinkai, Chem. Lett., 1991, 937 CrossRef CAS.
-
(a) D.-S. Guo, T.-X. Zhang, Y.-X. Wang and Y. Liu, Chem. Commun., 2013, 49, 6779 RSC;
(b) S. Shinkai, K. Araki, T. Matsuda, N. Nishiyama, H. Ikeda, I. Takasu and M. Iwamoto, J. Am. Chem. Soc., 1990, 112, 9053 CrossRef CAS;
(c) E. da Silva, A. N. Lazar and A. W. Coleman, J. Drug Del. Sci. Tech., 2004, 14, 3 CrossRef CAS;
(d) E. K. Stephens, Y. Tauran, A. W. Coleman and M. Fitzgerald, Chem. Commun., 2015, 51, 851 RSC.
- V. Wintgens, Z. Miskolczy, J.-M. Guigner, C. Amiel, J. G. Harangozó and L. Biczók, Langmuir, 2015, 31, 6655 CrossRef CAS PubMed.
- J. G. Harangozó, V. Wintgens, Z. Miskolczy, J.-M. Guigner, C. Amiel and L. Biczók, Langmuir, 2016, 32, 10651 CrossRef PubMed.
-
(a) M. Burnworth, L. Tang, J. R. Kumpfer, A. J. Duncan, F. L. Beyer, G. L. Fiore, S. J. Rowan and C. Weder, Nature, 2011, 472, 334 CrossRef CAS PubMed;
(b) X. Ma and H. Tian, Chem. Soc. Rev., 2010, 39, 70 RSC;
(c) M. L. Pellizzaro, K. A. Houton and A. Wilson, Chem. Sci., 2013, 4, 1825 RSC.
-
(a) A. M. Kloxin, A. M. Kasko, C. N. Salinas and K. S. Anseth, Science, 2009, 324, 59 CrossRef CAS PubMed;
(b) M. U. de la Orden, J. M. Montes, J. M. Urreaga, A. Bento, M. R. Ribeir, E. Perez and M. L. Cerrada, Polym. Degrad. Stab., 2015, 111, 78 CrossRef CAS.
-
(a) L. Sun, Y. Yang, C. M. Dong and Y. Wei, Small, 2011, 7, 401 CrossRef CAS PubMed;
(b) C.-C. Chen, W.-H. Ma and J.-C. Zhao, Chem. Soc. Rev., 2010, 39, 4206 RSC;
(c) W. Zhao, W.-H. Ma, C.-C. Chen, J.-C. Zhao and Z.-G. Shuai, J. Am. Chem. Soc., 2004, 126, 4782 CrossRef CAS PubMed.
- Y.-X. Wang, Y.-M. Zhang and Y. Liu, J. Am. Chem. Soc., 2015, 137, 4543 CrossRef CAS PubMed.
- T. Zhang, S. Sun, F. Liu, Y. Pang, J. Fan and X. Peng, Phys. Chem. Chem. Phys., 2011, 13, 9789 RSC.
-
(a) E. J. Bowen, Discuss. Faraday Soc., 1953, 14, 143 RSC;
(b) S. Kohtani, M. Tomohiro, K. Tokumura and R. Nakagaki, Appl. Catal., B, 2005, 58, 265 CrossRef CAS;
(c) W. E. Barnett and L. L. Needham, J. Org. Chem., 1971, 36, 4134 CrossRef CAS;
(d) M. F. Powell, J. Org. Chem., 1987, 52, 56 CrossRef CAS;
(e) K. Wang, D.-S. Guo, X. Wang and Y. Liu, ACS Nano, 2011, 5, 2880 CrossRef CAS PubMed.
-
(a) J. Andres, R. D. Hersch, J. E. Moser and A. S. Chauvin, Adv. Funct. Mater., 2014, 24, 5029 CrossRef CAS;
(b) L. A. J. Chrisstoffels, A. Adronov and M. J. Fréchet, Angew. Chem., Int. Ed., 2000, 39, 2163 CrossRef CAS;
(c) A. Ajayaghosh, V. K. Praveen, C. Vijayakumar and S. J. George, Angew. Chem., Int. Ed., 2007, 46, 6260 CrossRef CAS PubMed;
(d) K. V. Rao, K. K. R. Datta, M. Eswaramoorthy and S. J. George, Adv. Mater., 2013, 25, 1713 CrossRef CAS PubMed.
-
(a) I. Yildiz, S. Impellizzeri, E. Deniz, B. McCaughan, J. F. Callan and F. M. Raymo, J. Am. Chem. Soc., 2011, 133, 871 CrossRef CAS PubMed;
(b) P. G. Adams, A. M. Collins, T. Sahin, V. Subramanian, V. S. Urban, P. Vairaprakash, Y. Tian, D. G. Evans, A. P. Shreve and G. A. Montañ, Nano Lett., 2015, 15, 2422 CrossRef CAS PubMed;
(c) B. Radaram, J. Potvin and M. Levine, Chem. Commun., 2013, 49, 8259 RSC.
- Z. Xu, S. Peng, Y.-Y. Wang, J.-K. Zhang, A. I. Lazar and D.-S. Guo, Adv. Mater., 2016, 28, 7666 CrossRef CAS PubMed.
-
(a) A. Reisch and A. S. Klymchenko, Small, 2016, 12, 1968 CrossRef CAS PubMed;
(b) Y.-N. Hong, J. W. Y. Lam and B.-Z. Tang, Chem. Soc. Rev., 2011, 40, 5361 RSC;
(c) G. Scheibe, L. Kandler and H. Ecker, Naturwissenschaften, 1937, 25, 75 CrossRef CAS.
- I. Shulov, R. V. Rodik, Y. Arntz, A. Reisch, V. I. Kalchenko and A. S. Klymchenko, Angew. Chem., Int. Ed., 2016, 55, 15884 CrossRef CAS PubMed.
- J. Gao, J. Li, W.-C. Geng, F.-Y. Chen, X. Duan, Z. Zheng, D. Ding and D.-S. Guo, J. Am. Chem. Soc., 2018, 140, 4945 CrossRef CAS PubMed.
-
(a) R. Behrend, E. Meyer and F. I. Rusche, Liebigs Ann. Chem., 1905, 339, 1 CrossRef;
(b) J. Lagona, P. Mukhopadhyay, S. Chakrabarti and L. Isaacs, Angew. Chem., Int. Ed., 2005, 44, 4844 CrossRef CAS PubMed;
(c) W. Liu, S. K. Samanta, B. D. Smith and L. Isaacs, Chem. Soc. Rev., 2017, 46, 2391 RSC;
(d) W. L. Mock, Top. Curr. Chem., 1995, 175, 1 CrossRef CAS;
(e) K. Kim, N. Selvapalam, Y. H. Ko, K. M. Park, D. Kim and J. Kim, Chem. Soc. Rev., 2007, 36, 267 RSC;
(f) J. W. Lee, S. Samal, N. Selvapalam, H.-J. Kim and K. Kim, Acc. Chem. Res., 2003, 36, 621 CrossRef CAS PubMed;
(g) E. Masson, X. Ling, R. Joseph, L. Kyeremeh-Mensah and X. Lu, RSC Adv., 2012, 2, 1213 RSC;
(h) S. J. Barrow, S. Kasera, M. J. Rowland, J. del Barrio and O. A. Scherman, Chem. Rev., 2015, 115, 12320 CrossRef CAS PubMed.
-
(a) D. Whang, Y.-M. Jeon, J. Heo and K. Kim, J. Am. Chem. Soc., 1996, 118, 11333 CrossRef CAS;
(b) D. Whang and K. Kim, J. Am. Chem. Soc., 1997, 119, 451 CrossRef CAS;
(c) E. Lee, J. Heo and K. Kim, Angew. Chem., Int. Ed., 2000, 39, 2699 CrossRef CAS;
(d) A. I. Day, R. J. Blanch, A. P. Arnold, S. Lorenzo, G. R. Lewis and I. Dance, Angew. Chem., Int. Ed., 2002, 41, 275 CrossRef CAS;
(e) X.-J. Cheng, L.-L. Liang, K. Chen, N.-N. Ji, X. Xiao, J.-X. Zhang, Y.-Q. Zhang, S.-F. Xue, Q.-J. Zhu, X.-L. Ni and Z. Tao, Angew. Chem., Int. Ed., 2013, 52, 7252 CrossRef CAS PubMed.
- J. Murray, K. Kim, T. Ogoshi, W. Yao and B. C. Gibb, Chem. Soc. Rev., 2017, 46, 2479 RSC.
- H.-J. Kim, J. Heo, W. S. Jeon, E. Lee, J. Kim, S. Sakamoto, K. Yamaguchi and K. Kim, Angew. Chem., Int. Ed., 2001, 40, 1526 CrossRef CAS PubMed.
-
(a) D. Ma, B. Zhang, U. Hoffmann, M. G. Sundrup, M. Eikermann and L. Isaacs, Angew. Chem., Int. Ed., 2012, 51, 11358 CrossRef CAS PubMed;
(b) D. Ma, G. Hettiarachchi, D. Nguyen, B. Zhang, J. B. Wittenberg, P. Y. Zavalij, V. Briken and L. Isaacs, Nat. Chem., 2012, 4, 503 CrossRef CAS PubMed;
(c) N. Dong, S.-F. Xue, Q.-J. Zhu, Z. Tao, Y. Zhao and L.-X. Yang, Supramol. Chem., 2008, 20, 659 CrossRef CAS;
(d) W. Li, A. T. Bockus, B. Vinciguerra, L. Isaacs and A. R. Urbach, Chem. Commun., 2016, 52, 8537 RSC;
(e) B. Gong, B.-K. Choi, J.-Y. Kim, D. Shetty, Y. H. Ko, N. Selvapalam, N. K. Lee and K. Kim, J. Am. Chem. Soc., 2015, 137, 8908 CrossRef CAS PubMed;
(f) H. Chen, J. Y. W. Chan, S. Li, J.-J. Liu, I. Wyman, S. M. Y. Lee, D. H. Macartney and R. Wang, RSC Adv., 2015, 5, 63745 RSC;
(g) C. Kim, S. S. Agasti, Z. Zhu, L. Isaacs and V. M. Rotello, Nat. Chem., 2010, 2, 962 CrossRef CAS PubMed;
(h) G. Y. Tonga, Y. Jeong, B. Duncan, T. Mizuhara, R. Mout, R. Das, S. T. Kim, Y.-C. Yeh, B. Yan, S. Hou and V. M. Rotello, Nat. Chem., 2015, 7, 597 CrossRef CAS PubMed;
(i) M. J. Webber, E. A. Appel, B. Vinciguerra, A. B. Cortinas, L. S. Thapa, S. Jhunjhunwala, L. Isaacs, R. Langer and D. G. Anderson, Proc. Natl. Acad. Sci. U. S. A., 2016, 113, 14189 CrossRef CAS PubMed;
(j) A. T. Bockus, L. C. Smith, A. G. Grice, O. A. Ali, C. C. Young, M. W. Obley, A. Leek, J. L. Roberts, B. Vinciguerra, L. Isaacs and A. R. Urbach, J. Am. Chem. Soc., 2016, 138, 16549 CrossRef CAS PubMed;
(k) C. Stoffelen, J. Voskuhl, P. Jonkheijm and J. Huskens, Angew. Chem., Int. Ed., 2014, 53, 3400 CrossRef CAS PubMed.
-
(a) J. L. Sessler, B. Wang and A. Harriman, J. Am. Chem. Soc., 1993, 115, 10418 CrossRef CAS;
(b) Y. Liu, C. Yu, H. Jin, B. Jiang, X. Zhu, Y. Zhou, Z. Lu and D. Yan, J. Am. Chem. Soc., 2013, 135, 4765 CrossRef CAS PubMed;
(c) J. Yang, G. Yu, D. Xia and F. Huang, Chem. Commun., 2014, 50, 3993 RSC;
(d) D. Xia, G. Yu, J. Li and F. Huang, Chem. Commun., 2014, 50, 3606 RSC;
(e) J. C. Barnes, M. Frasconi, R. M. Young, N. H. Khdary, W.-G. Liu, S. M. Dyar, P. R. McGonigal, I. C. Gibbs-Hall, C. S. Diercks, A. A. Sarjeant, C. L. Stern, W. A. Goddard, III, M. R. Wasielewski and J. F. Stoddart, J. Am. Chem. Soc., 2014, 136, 10569 CrossRef CAS PubMed;
(f) Y. Wang, J.-F. Xu, Y.-Z. Chen, L.-Y. Niu, L.-Z. Wu, C.-H. Tung and Q.-Z. Yang, Chem. Commun., 2014, 50, 7001 RSC;
(g) H.-L. Sun, Y. Chen, J. Zhao and Y. Liu, Angew. Chem., Int. Ed., 2015, 54, 9376 CrossRef CAS PubMed;
(h) X. Chi, X. Ji, D. Xia and F. Huang, J. Am. Chem. Soc., 2015, 137, 1440 CrossRef CAS PubMed;
(i) D. Xia, P. Wei, B. Shi and F. Huang, Chem. Commun., 2016, 52, 513 RSC;
(j) F. B. Schwarz, T. Heinrich, A. Lippitz, W. E. S. Unger and C. A. Schalley, Chem. Commun., 2016, 52, 14458 RSC.
-
(a) A. Natansohn and P. Rochon, Chem. Rev., 2002, 102, 4139 CrossRef CAS PubMed;
(b) B. H. M. Dhammika and S. C. Burdette, Chem. Soc. Rev., 2012, 41, 1809 RSC;
(c) A. A. Beharry and G. A. Woolley, Chem. Soc. Rev., 2011, 40, 4422 RSC;
(d) P. Ceroni, A. Credi and M. Venturi, Chem. Soc. Rev., 2014, 43, 4068 RSC;
(e) D.-H. Qu, Q.-C. Wang, Q.-W. Zhang, X. Ma and H. Tian, Chem. Rev., 2015, 115, 7543 CrossRef CAS PubMed.
- L. Shao, B. Hua, J. Sun, Q. Li, J. Yang and G. Yu, Tetrahedron Lett., 2017, 58, 1863 CrossRef CAS.
- S. Mura, J. Nicolas and P. Couvreur, Nat. Mater., 2013, 12, 991 CrossRef CAS PubMed.
- C. Hu, N. Ma, F. Li, Y. Fang, Y. Liu, L. Zhao, S. Qiao, X. Li, X. Jiang, T. Li, F. Shen, Y. Huang, Q. Luo and J. Liu, ACS Appl. Mater. Interfaces, 2018, 10, 4603 CrossRef CAS PubMed.
- K. M. Park, K. Baek, Y. H. Ko, A. Shrinidhi, J. Murray, W. H. Jang, K. H. Kim, J.-S. Lee, J. Yoo, S. Kim and K. Kim, Angew. Chem., Int. Ed., 2018, 57, 3132 CrossRef CAS PubMed.
-
(a) J. M. Glasscock, Y. Zhu, P. Chowdhury, J. Tang and F. Gai, Biochemistry, 2008, 47, 11070 CrossRef CAS PubMed;
(b) A. Aneja, N. Mathur, P. K. Bhatnagar and P. Mathur, J. Biol. Phys., 2008, 34, 487 CrossRef CAS PubMed;
(c) A. Coskun and E. U. Akkaya, J. Am. Chem. Soc., 2006, 128, 14474 CrossRef CAS PubMed;
(d) J. Kim, D. T. McQuade, A. Rose, Z. Zhu and T. W. Swager, J. Am. Chem. Soc., 2001, 123, 11488 CrossRef CAS PubMed;
(e) E. A. Jares-Erijman and T. M. Jovin, Nat. Biotechnol., 2003, 21, 1387 CrossRef CAS PubMed.
- J. J. Reczek, A. A. Kennedy, B. T. Halbert and A. R. Urbach, J. Am. Chem. Soc., 2009, 131, 2408 CrossRef CAS PubMed.
- S. Kushwaha, A. Maity, M. Gangopadhyay, S. Ravindranathan, P. R. Rajamohanan and A. Das, Langmuir, 2017, 33, 10989 CrossRef CAS PubMed.
- Q. Song, F. Li, Z. Wang and X. Zhang, Chem. Sci., 2015, 6, 3342 RSC.
-
(a) A. M. Brouwer, C. Frochot, F. G. Gatti, D. A. Leigh, L. Mottier, F. Paolucci, S. Roffia and G. W. H. Wurpel, Science, 2001, 291, 2124 CrossRef CAS PubMed;
(b) J.-M. Lehn, Chem. Soc. Rev., 2007, 36, 151 RSC.
- Y. Yang, P. He, Y. Wang, H. Bai, S. Wang, J.-F. Xu and X. Zhang, Angew. Chem., Int. Ed., 2017, 56, 16239 CrossRef CAS PubMed.
- Y. Jiao, W.-L. Li, J.-F. Xu, G. Wang, J. Li, Z. Wang and X. Zhang, Angew. Chem., Int. Ed., 2016, 55, 8933 CrossRef CAS PubMed.
-
(a) H. J. H. Fenton, J. Chem. Soc., Trans., 1894, 65, 899 RSC;
(b) X. Zhou, J. Lan, G. Liu, K. Deng, Y. Yang, G. Nie, J. Yu and L. Zhi, Angew. Chem., Int. Ed., 2012, 51, 178 CrossRef CAS PubMed.
- T. Ogoshi, S. Kanai, S. Fujinami, T. A. Yamagishi and Y. Nakamoto, J. Am. Chem. Soc., 2008, 130, 5022 CrossRef CAS PubMed.
-
(a) C. Li, Q. Xu, J. Li, F. Yao and X. Jia, Org. Biomol. Chem., 2010, 8, 1568 RSC;
(b) T. Ogoshi, K. Demachi, K. Kitajima and T. A. Yamagishi, Chem. Commun., 2011, 47, 10290 RSC;
(c) C. Li, K. Han, J. Li, H. Zhang, J. Ma, X. Shu, Z. Chen, L. Weng and X. Jia, Org. Lett., 2012, 14, 42 CrossRef CAS PubMed;
(d) H. Deng, X. Shu, X. Hu, J. Li, X. Jia and C. Li, Tetrahedron Lett., 2012, 53, 4609 CrossRef CAS;
(e) Y. Guan, M. Ni, X. Hu, T. Xiao, S. Xiong, C. Lin and L. Wang, Chem. Commun., 2012, 48, 8529 RSC.
-
(a) N. L. Strutt, H. Zhang, S. T. Schneebeli and J. F. Stoddart, Acc. Chem. Res., 2014, 47, 2631 CrossRef CAS PubMed;
(b) M. Xue, Y. Yang, X. Chi, Z. Zhang and F. Huang, Acc. Chem. Res., 2012, 45, 1294 CrossRef CAS PubMed;
(c) H. Ju, F. Zhu, X. Hao, Z. Wu and F. Huang, Macromol. Rapid Commun., 2017, 38, 1700232 CrossRef PubMed;
(d) Y. Yao, M. Xue, J. Chen, M. Zhang and F. Huang, J. Am. Chem. Soc., 2012, 134, 15712 CrossRef CAS PubMed;
(e) H. Zhu, B. Shi, L. Gao, Y. Liu, P.-R. Liu, L. Shangguan, Z. Mao and F. Huang, Polym. Chem., 2017, 8, 7108 RSC;
(f) Y. Ma, X. Chi, X. Yan, J. Liu, Y. Yao, W. Chen, F. Huang and J. Hou, Org. Lett., 2012, 14, 1532 CrossRef CAS PubMed;
(g) Y. Liu, B. Lou, L. Shangguan, J. Cai, H. Zhu and B. Shi, Macromolecules, 2018, 51, 1351 CrossRef CAS.
- T. Ogoshi, K. Umeda, T. Yamagishi and Y. Nakamoto, Chem. Commun., 2009, 4874 RSC.
-
(a) T. Ogoshi, M. Hashizume, T. A. Yamagishi and Y. Nakamoto, Chem. Commun., 2010, 46, 3708 RSC;
(b) G. Yu, M. Xue, Z. Zhang, J. Li, C. Han and F. Huang, J. Am. Chem. Soc., 2012, 134, 13248 CrossRef CAS PubMed;
(c) S. Dasgupta and P. S. Mukherjee, Org. Biomol. Chem., 2017, 15, 762 RSC.
-
(a) K. Yang, Y. Pei, J. Wen and Z. Pei, Chem. Commun., 2016, 52, 9316 RSC;
(b) L. Shangguan, Q. Chen, B. Shi and F. Huang, Chem. Commun., 2017, 53, 9749 RSC;
(c) Z. Li, J. Yang and F. Huang, Chin. J. Chem., 2018, 36, 59 CrossRef CAS;
(d) Z. Li, J. Yang, G. Yu, J. He, Z. Abliz and F. Huang, Org. Lett., 2014, 16, 2066 CrossRef CAS PubMed;
(e) Y. Ma, M. Xue, Z. Zhang, X. Chi and F. Huang, Tetrahedron, 2013, 69, 4532 CrossRef CAS;
(f) H. Zhu, J. Liu, B. Shi, H. Wang, Z. Mao, T. Shan and F. Huang, Mater. Chem. Front., 2018, 2, 1475 RSC.
- X.-F. Ji, D.-Y. Xia, X. Yan, H. Wang and F.-H. Huang, Acta Polym. Sin., 2017, 9–18 CAS.
- L. Jiang, X. Huang, D. Chen, H. Yan, X. Li and X. Du, Angew. Chem., Int. Ed., 2017, 56, 2655 CrossRef CAS PubMed.
- R. R. Avirah, K. Jyothish and D. Ramaiah, Org. Lett., 2007, 9, 121 CrossRef CAS PubMed.
- F. Zhang, J. Ma, Y. Sun, I. Boussouar, D. Tian, H. Li and L. Jiang, Chem. Sci., 2016, 7, 3227 RSC.
- Q. Yao, B. Lü, C. Ji, Y. Cai and M. Yin, ACS Appl. Mater. Interfaces, 2017, 9, 36320 CrossRef CAS PubMed.
- Q. Duan, Y. Cao, Y. Li, X.-Y. Hu, T. Xiao, C. Lin, Y. Pan and L. Wang, J. Am. Chem. Soc., 2013, 135, 10542 CrossRef CAS PubMed.
- X.-Y. Hu, X. Liu, W. Zhang, S. Qin, C. Yao, Y. Li, D. Cao, L. Peng and L. Wang, Chem. Mater., 2016, 28, 3778 CrossRef CAS.
- K. Yang, Y. Chang, J. Wen, Y. Lu, Y. Pei, S. Cao, F. Wang and Z. Pei, Chem. Mater., 2016, 28, 1990 CrossRef CAS.
-
(a) Y. S. Zhao, H. Fu, A. Peng, Y. Ma, Q. Liao and J. Yao, Acc. Chem. Res., 2010, 43, 409 CrossRef CAS PubMed;
(b) H. Zheng, Y. Li, H. Liu, X. Yin and Y. Li, Chem. Soc. Rev., 2011, 40, 4506 RSC;
(c) B. He, J. Dai, D. Zherebetskyy, T.-L. Chen, B.-A. Zhang, S. J. Teat, Q. Zhang, L. Wang and Y. Liu, Chem. Sci., 2015, 6, 3180 RSC.
- Y. Liu, M. Chen, T. Cao, Y. Sun, C. Li, Q. Liu, T. Yang, L. Yao, W. Feng and F. Li, J. Am. Chem. Soc., 2013, 135, 9869 CrossRef CAS PubMed.
- B. Shi, K. Jie, Y. Zhou, J. Zhou, D. Xia and F. Huang, J. Am. Chem. Soc., 2016, 138, 80 CrossRef CAS PubMed.
-
(a) H.-F. Zhang, K. Maslov, G. Stoica and L. V. Wang, Nat. Biotechnol., 2006, 24, 848 CrossRef CAS PubMed;
(b) H. Li, P. Zhang, L. P. Smaga, R. A. Hoffman and J. Chan, J. Am. Chem. Soc., 2015, 137, 15628 CrossRef CAS PubMed.
- Y. Shi, H. Qin, S. Yang and D. Xing, Nano Res., 2016, 9, 3644 CrossRef CAS.
- J. T. Robinson, S. M. Tabakman, Y. Liang, H. Wang, H. S. Casalongue, D. Vinh and H. Dai, J. Am. Chem. Soc., 2011, 133, 6825 CrossRef CAS PubMed.
- G. Yu, J. Yang, X. Fu, Z. Wang, L. Shao, Z. Mao, Y. Liu, Z. Yang, F. Zhang, W. Fan, J. Song, Z. Zhou, C. Gao, F. Huang and X. Chen, Mater. Horiz., 2018, 5, 429 RSC.
|
This journal is © the Partner Organisations 2018 |