The impact of ultraviolet B (UV-B) radiation in combination with different temperatures in the early life stage of zebrafish (Danio rerio)
Received
24th June 2017
, Accepted 31st October 2017
First published on 2nd November 2017
Abstract
Ultraviolet B (UV-B) radiation is an environmental stressor with detrimental effects on many aquatic organisms including fish. In addition, UV-B exposure combined with other environmental factors could have even more negative effects. The purpose of this study was to investigate the effect of UV-B radiation exposure on zebrafish embryos/larvae in terms of survival, developmental toxicity and the mRNA levels of the genes related to oxidative stress and innate immune response at different temperatures (24 °C, 28 °C and 30 °C). Zebrafish embryos were exposed to 3.3 W m−2 UV-B radiation and/or 24 °C, 28 °C (for the control) and 30 °C temperatures between 4 and 96 h post-fertilization. The mortality, hatching rate, malformations and heartbeat rate were evaluated. The results demonstrated that UV-B exposure or different temperatures (24 °C and 30 °C) induced developmental toxicity, including delayed hatching, increased the occurrence of malformations, and reduced the heartbeat rate and survival. The combined exposure to UV-B and different temperatures (24 °C and 30 °C) resulted in greater adverse effects on embryonic development. Furthermore, RT-PCR results showed that the mRNA levels of superoxide dismutase 1 (sod1), catalase 1 (cat1), heat shock protein 70 (hsp70), interleukin-1 beta (il-1β) and tumor necrosis factor alpha (tnfα) genes were significantly up-regulated in all of the treatment groups. These results revealed that the interaction between UV-B and temperature impaired the development of zebrafish embryos and disrupted their metabolism.
Introduction
Under natural conditions, aquatic organisms are often exposed to many abiotic stress factors simultaneously and it is well known that these factors have some structural and physiological impacts on living organisms. Among these factors, UV-B radiation is the most encountered one in the environment and it has detrimental effects on aquatic life. To date, the negative effects of UV-B radiation on various aquatic organisms such as fish, crab, sea urchin and daphnia have been reported.1 The increased malformation rate, reduced survival and hatching success of fish eggs, embryos and larvae induced by UV-B radiation have also been demonstrated.1,2 Moreover, it has been stated that the deleterious effects of UV-B radiation are mediated by the enhanced reactive oxygen species (ROS) level which leads to the damage of protein, lipid and DNA structures.1,3 Furthermore, the studies that rely on the interactive effects of UV-B with other abiotic stressors have indicated that abiotic stressors such as pollutants, low pH and low/high temperature can alter the deleterious effects of UV-B radiation on aquatic life.4–6 Among the abiotic stressors, water temperature is the main environmental driver that influences many metabolic, behavioral and physiological processes in aquatic life. For example, it has been shown to affect survival, growth, the immune system, larval size, yolk utilization efficiency, malformation rate and mortality in fish.5,7–9 Moreover, several studies have reported that a change in the temperature has a considerable influence on a variety of physiological stress responses in fish embryos.9–11 These studies have indicated that an elevated temperature leads to a higher metabolic rate, i.e. an increased oxygen consumption and consequently enhanced ROS levels.10,11 This increase can cause protein, lipid or DNA damage, which impairs animal performance.9–11 On the other hand, a decline in the temperature causes a lower metabolic rate, thus slower development. Due to a slow development, the exposure period of each developmental stage to other abiotic stressors increases and consequently their deleterious effects are greater.12
The additive and/interactive effects of temperature and UV-B radiation on frog embryo, juvenile and adult fish have been indicated in a few studies.12–15 However, up to now, there is no study on the additive and/interactive effects of these stressors on fish embryos. Therefore, this study has been conducted to explore the additive or synergistic effects between the temperature (24 °C and 30 °C) and UV-B radiation on the embryonic development of zebrafish. In the current study, a zebrafish embryo is preferred because of its small size, transparency, high fecundity, rapid embryogenesis and physiological similarity to mammals. Moreover, it is easy to maintain and handle. These properties make this embryo a useful research model for toxicity studies.16–19
Materials and methods
Fish maintenance and embryo collection
AB strain zebrafish were taken from the Fisheries Faculty of Ataturk University which were previously provided by Oregon State University (US) and maintained by the Aquatic Biotechnology Lab. Healthy 5 month old adult fish were selected and acclimatized separately in glass tanks at a constant temperature of 28 °C for at least 7 days with a 14
:
10 h light–dark photoperiod. The fish were fed with Artemia salina twice a day. A total of 10 female and 20 male zebrafish were maintained. Spawning was triggered in the morning when the light was turned on and completed within 30 min. At 4 hpf, embryos were examined under a stereomicroscope and those embryos that had developed normally and reached the blastula stage were selected for subsequent experiment. Embryos were placed at a low temperature (24 °C), normal temperature (28 °C) and a high temperature (30 °C) in Petri dishes containing an embryo medium (5 mM NaCl, 0.17 mM KCl, 0.33 mM CaCl2, 0.33 mM MgSO4, 0.01% methylene blue). Each medium was replaced every 24 h. The experiments were performed in accordance with the Code of Ethics of the World Medical Association (Declaration of Helsinki) for animal experiments and were approved by the local animal ethics committee at Ataturk University (Erzurum, Turkey).
Treatments
The UV-B treatment groups were exposed to visible light plus UV-B, while control groups received visible light only. The fish were exposed to UV-B radiation for 3 h each day by using a UV-B lamp (white light was supplemented with UV-B Philips tubes (TL100W/12) filtered with 0.13 mm thick cellulose diacetate) at an irradiance of 3.3 W m−2, which generated the total daily dose of 1188 J m−2. The spectral irradiance was determined with an Ultraviolet Meter Model 3D (Solar Light Co, Glenside, PA, USA). These treatments were carried out simultaneously at three temperatures; low temperature (24 °C), normal temperature (28 °C) and high temperature (30 °C) to explore the potential synergistic effect of different combinations of the two abiotic stressors. In each experimental group 150 embryos were used and each treatment was performed in triplicate.
Mortality, hatching, heart rate and malformation
The total percentage of mortality and teratogenic responses including hatching, malformation and heartbeat were observed and recorded at 24, 48, 72, and 96 hpf by using a standard stereomicroscope (Imager. A2 Zeiss, Germany), and the dead embryos were removed from the medium as soon as possible. Mortality was defined as coagulation, missing heartbeat, failure to develop a somite, and a non-detached tail. The hatching rate was calculated by the rate of successful hatching embryos divided by the total number of embryos in each replicate. Malformations including pericardial edema, tail deformity, yolk sac edema, and spinal curvature were observed under 24, 48, 72, and 96 hpf after exposure to UV-B and/or different temperatures. The malformation rate was determined from the percentage of the total number of malformed larvae (including one of the above malformations) to the total number of larvae hatched during the test. For heartbeat counting, 24 embryos were randomly selected (for each treatment) and counted for 10 s per embryo after being stabilized at room temperature for 10 min until the embryonic heartbeat reached the steady state.
RNA isolation and reverse transcription real-time PCR
Total RNA was extracted from zebrafish larvae (60 larvae in each sample) using an RNeasy mini kit for animal tissue (Qiagen, Basel, Switzerland) according to the manufacturer's instructions. A DNase treatment (Qiagen) was used to remove sample contamination by genomic DNA, and 30 μL of water (RNase/DNase free) was used for the elution of RNA. The concentration and the ratio of 260/280 of total RNA were determined using a Nanodrop ND-1000 spectrophotometer. The cDNA synthesis was performed by using the RT2 First Strand cDNA Synthesis Kit (Qiagen, Basel, Switzerland). A Rotor-gene Q instrument was used to perform Real-time PCR by using SYBR Green (RT2 SYBR Green master mix, Qiagen, Cat. No: 330500) mixed with cDNA and gene-specific primer pairs (Table 1). The PCRs were repeated three times. The relative expression levels of both the reference gene and genes of interest were calculated by using the ΔΔCt method and reported as a fold change over the control. Analysis of the relative gene expression was done according to the Qiagen Data Analysis Center. β-Actin was used as a housekeeping gene and suitable negative controls were maintained for all the primers.
Table 1 Primer sequences of this study
Genes |
Forward primer (5′–3′) |
Reverse primer (5′–3′) |
Ref. |
sod1
|
GTCCGCACTTCAACCCTCA |
TCCTCATTGCCACCCTTCC |
48
|
cat1
|
CAAGGTCTGGTCCCATAAA |
TGACTGGTAGTTGGAGGTAA |
48
|
hsp70
|
GGAAAAGAGGGAAGCTTTGG |
ACGTTCCATGTTTCCAGACC |
49
|
il-1β
|
CATTTGCAGGCCGTCACA |
GGACATGCTGAAGCGCACTT |
50
|
tnfα
|
GGCTGGAAAACAACGAGATCA |
AAGATCAAAGACGGCTCCAA |
51
|
β-act
|
TGCTGTTTTCCCCTCCATTG |
TTCTGTCCCATGCCAACCA |
50
|
Statistical analysis
The results of mortality, malformation, hatching heart rates were presented as mean ± standard error (SEM). On the other hand, the gene expression results were demonstrated as mean + standard deviation (SDM). To evaluate statistically the difference in the mentioned rates among the studied groups and to determine the interaction between UV-B and temperature, two-way ANOVA followed by Tukey's multiple comparison test was performed using GraphPad Prism software Prism version 6.00 for Windows, (GraphPad Software, La Jolla California USA). On the other hand, to assess the statistical significance of the fold change of the studied gene expressions between the treated and control groups, Student's t-test was used provided by the Qiagen Gene Globe Data Analysis Center. In all cases, values were considered statistically significant at p < 0.05. *represents p < 0.05, **p < 0.01, ***p < 0.001 and ****p < 0.0001.
Results
Developmental toxicity
Two-way ANOVA analysis of mortality, hatching, malformation and heart rate demonstrated that separate and combined UV-B and temperature treatments had a statistically significant effect on these parameters. However, the interaction term of treatments was found to be not statistically significant indicating that the effects of the treatments were independent except for the hatching rate at 48 and 72 hpf.
Fig. 1 demonstrates the effects of UV-B and different temperature (24, 28 and 30 °C) treatments on the mortality rate in zebrafish embryos at 24, 48, 72 and 96 hpf. As can be seen from this figure, embryos from the control group developed normally and showed an overall mortality of less than 3%. A significantly increased mortality rate in the 24 °C, 24 °C + UV-B, 30 °C + UV-B treated groups was obtained at 24, 48, 72 and 96 hpf with respect to the control group. However, the most profound increase was observed in the 24 °C + UV-B group. At 96 hpf, the mortality rate of this group was 21% ± 2.8. The time dependent analysis of each stressor indicated that the embryos, which survived after 72 h of exposure, did not show any further mortality.
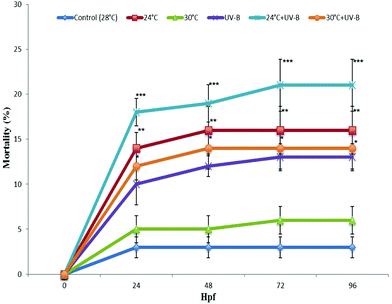 |
| Fig. 1 Mortality rate (%) of zebrafish embryos/larvae exposed to UV-B and/or different temperatures. Mortality was recorded at 24, 48, 72 and 96 h post fertilization. Data are presented as mean ± standard error (SEM) of three independent replicates. Asterisks (*, **, *** and ****) indicate a significant change with p < 0.05, p < 0.01, p < 0.001 and p < 0.0001, respectively. | |
The effects of both stress factors on the hatching rates of the control and treated groups at different times are shown in Fig. 2. With respect to the control group, UV-B and/temperature exposure decreased the hatching rate at 48 hpf, 72 hpf and 96 hpf. These decreases were found to be statistically significant for all the treated groups at 48 hpf. However, the significantly decreased rate was observed only for UV-B, 24 °C, 24 °C + UV-B groups at 72 hpf and 24 °C and 24 °C + UV-B groups at 96 hpf. Overall, the most significant and profound decrease was observed in 24 °C + UV-B treatment.
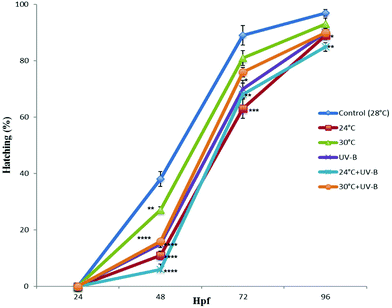 |
| Fig. 2 Hatching rate (%), between 24 and 96 hpf, of zebrafish embryos/larvae exposed to UV-B and/or different temperatures. The hatching rate was recorded at 24, 48, 72 and 96 h post fertilization. Data are presented as mean ± standard error (SEM) of three independent replicates. Asterisks (*, **, *** and ****) indicate a significant change with p < 0.05, p < 0.01, p < 0.001 and p < 0.0001, respectively. | |
Fig. 3a and b indicate the effects of UV-B and/or temperature on the malformation and heartbeat rate, respectively. Relative to the control, an increase in the malformation rates was obtained in all treated groups (11.2%
:
24 °C; 10.4%
:
UV-B; 16.1%
:
24 °C + UV-B and 12.4%
:
30 °C + UV-B). The increased malformation rates were significant for 24 °C, 24 °C + UV-B and 30 °C + UV-B groups. Moreover, the most profound changes in this rate were obtained for the 24 °C + UV-B group (Fig. 3a). To define the malformation rate, the observed malformations such as pericardial edema, tail deformity, yolk sac edema and spinal curvature, shown in Fig. 4, were totally counted. As can be seen from the heartbeat rate results, the heartbeat rates of the larvae from 24 °C, UV-B, 24 °C + UV-B, 30 °C + UV-B groups reduced by 5%, 5%, 10% and 5%, respectively, compared to the control group. This rate decreased in UV-B and/temperature treatments. However, 24 °C + UV-B treatment significantly decreased this rate (Fig. 3b).
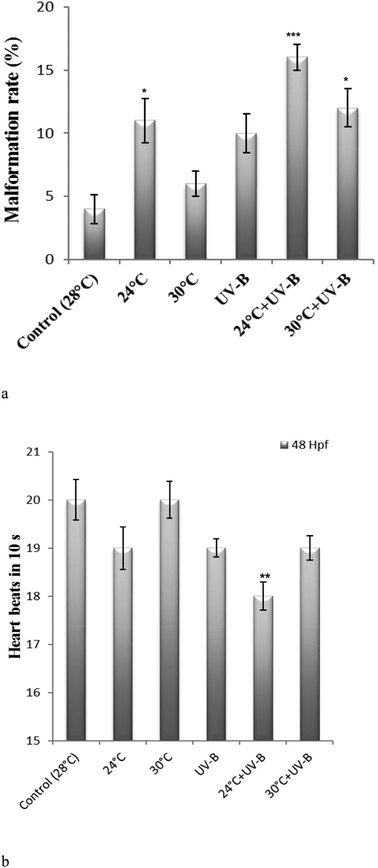 |
| Fig. 3 Malformation and the heartbeat rate in zebrafish embryos exposed to UV-B and/or different temperatures. Data are presented as mean ± standard error (SEM) of three independent replicates. Asterisks (*, **, *** and ****) indicate a significant change with p < 0.05, p < 0.01, p < 0.001 and p < 0.0001, respectively. | |
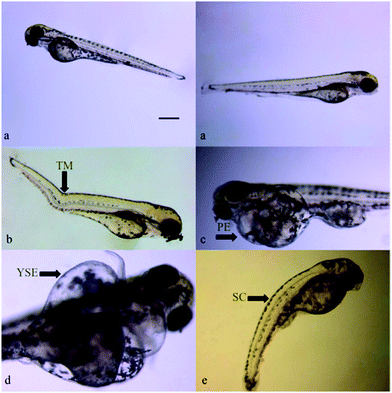 |
| Fig. 4 Malformations of zebrafish with different treatments (a) control, (b) tail malformation (TM), (c) pericardial edema (PE), (d) yolk sac edema (YSE), (e) spinal curvature (SC). Scale bar: 200 μm. | |
Expression of oxidative stress, hsp70 and immune response related genes
The mRNA expressions of oxidative stress and immune response related genes including sod1, cat1, hsp70, il-1β, and tnfα were analyzed in zebrafish larvae from the control and UV-B and/temperature treated groups for 96 hpf. β-Actin was used as a housekeeping gene for normalization (Fig. 5). UV-B and/or different temperature treatments led to a significant upregulation of sod1, cat1, hsp70, il-1β, and tnfα gene expression with respect to the control groups (Fig. 5a, b, c, d and e). However, the more profound upregulation of sod1, il-1β, and tnfα gene expressions was acquired for the 24 °C + UV-B treated group as compared with other groups. Moreover, the most increased expression of hsp70 and cat1 was observed in the 30 °C + UV-B group when compared with the control and other groups. These results were also supported by the heat map result based on an unsupervised hierarchical clustering of the studied genes of the control and the stress factor-treated groups (Fig. 6).
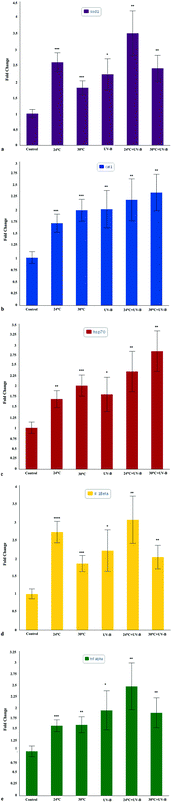 |
| Fig. 5 Gene expression (fold change) of oxidative stress (sod1 and cat1), hsp70 and immune related genes (il-1β and tnfα) of zebrafish larvae after exposure to UV-B and/or different temperatures. Data are presented as mean ± standard deviation (SDM) of three independent replicates. Asterisks (*, **, *** and ****) indicate a significant change with p < 0.05, p < 0.01, p < 0.001 and p < 0.0001, respectively. | |
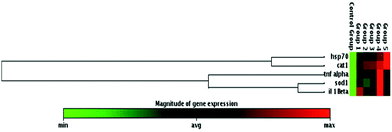 |
| Fig. 6 The clustergram presenting non-supervised hierarchical clustering of the entire dataset to display a heat map with dendrograms indicating co-regulated genes across groups. Group 1: 24 °C; Group 2: 30 °C; Group 3: UV-B; Group 4: 24 °C + UV-B; Group 5: 30 °C + UV-B. Red indicates high expression, while green represents low expression. | |
Discussion
Numerous abiotic stress factors influence the physiological, molecular and cellular mechanism of fish. Many studies related to the effects of abiotic stress factors on aquatic life, generally investigated only single stressor exposure. However, in nature, living organisms simultaneously expose many stress factors.4,20,21 Therefore, to obtain the real scenario about the effects of these stressors on organisms, their interactive and/or additive effects should be explored. In this context, the current study was conducted to elucidate the synergistic and/or additive effects of two common abiotic stress factors, UV-B and different temperatures on zebrafish embryos by evaluating their developmental toxicity and mortality. To further understand their mechanisms of toxicity, the expressions of oxidative stress and immune related genes were also investigated.
To assess the developmental toxicity of UV-B and temperature, the mortality, hatching, malformation and the heartbeat rates were measured since these parameters enable the elucidation of the biological response of zebrafish at an early life stage to stress factors. The decreased heartbeat, hatching rates, increased malformation, and the mortality rates demonstrated that UV-B and/temperature exposure is toxic to the development of zebrafish embryos, by delaying hatching processes, leading to a series of malformations, followed by mortality. In agreement with our findings, previous studies indicated that UV-B and/temperature exposure affected the growth, survival, mortality, hatching rate and enzyme activity of aquatic organisms.4,15,22 Moreover, Dong et al.,23 Azevedo et al.,21 Pype et al.,24 and Hallare et al.25 reported that UV-B radiation or different temperature treatment increased the malformations, the mortalities of eggs, embryos and larvae and reduced hatching success. The decreased hatching rate induced by UV-B and/temperature indicated the inhibition/retardation of these processes, which might be a result of the inhibition of mitosis or the suppression of embryogenesis or the inability of the emerging larvae to break the eggshell. Furthermore, the reduced hatching success can be due to the inhibition of the hatching enzyme, chorinase and zebrafish hatching enzyme 1 activity induced by the UV-B and/temperature-mediated OH˙ production.9,26 Another reason for delayed hatching may be due to the observed developmental malformations such as pericardial edema, tail deformity, yolk sac edema and spinal curvature.27 Similarly, pericardial edema in zebrafish embryos induced by different stress factors including UV-B and high or low temperature was demonstrated in previous studies.23,28 This defect may be caused by heart abnormalities which may be induced by the insufficient synthesis of cardiac troponin T since the role of inadequate synthesis of this contractile protein in the pericardial edema was previously suggested by Dambal et al.29 In addition to pericardial edema, the decreased heartbeat rate by UV-B and temperature exposure may indicate heart abnormalities.30 UV-B decreased heartbeat rate in zebrafish embryos was reported by Wu et al.,31 which is in agreement with our findings. Similarly, the decreased heartbeat rate in zebrafish embryos was also indicated by Hallare and his colleagues.25 The decreased heartbeat rate induced by temperature may be related to the alteration in the metabolism of zebrafish embryos since zebrafish is an ectothermic organism and temperature is an essential factor influencing all kinds of metabolic processes in ectothermic organisms.9,14
UV-B and/temperature increased the malformation and mortality rate and delayed hatching might be related to the increased oxidative stress since this stress firstly occurred in the case of environmental stressor exposure.32,33 Therefore, to understand the association between UV-B and/temperature exposure and antioxidant defense response, the expressions of sod1 and cat1 were examined. The upregulation of these genes in UV-B, temperature and UV-B and temperature treated groups indicated that the antioxidant defense response was enhanced to protect the cell against the harmful effects of oxidative stress induced by UV-B exposure and/or different temperatures. Similar to our findings, the induction of sod1 due to UV-B exposure was previously demonstrated in developing zebrafish.34 The genes responsible for the production of SOD and CAT are differently regulated during the early life stage in aquatic organisms. For example, Dale et al.35 observed different regulations of antioxidant related genes during the early stages of zebrafish development. In addition to the antioxidant defense response to environmental stressors, heat shock proteins are involved in cellular defense mechanisms under a variety of stressful conditions such as temperature, heavy metals, pesticides, and UV radiation.36 Previous studies suggested that HSPs play significant roles during normal embryo development in zebrafish embryos.37 HSPs act as molecular chaperons during embryonic development because they are involved in cell movement, proliferation and morphogenesis. In our study we observed that exposure to UV-B and/or different temperatures increased the mRNA level of hsp70 in zebrafish larvae. Similar results have been found in zebrafish where UV-B exposure3 or low and high temperature38 induced the expression of hsp70.
It is well known that the growth, development and survival features of organisms partly depend on the capacity of their immune system. Since fish development occurs in an open environment, the fish embryos face many stressors during their development. Therefore, their immune system is really sensitive to these stress factors. A study on the innate immune system function of zebrafish provides a clarification of the environmental stress factor-induced alterations in the immune system. Many studies indicated that UV-B radiation, and low and high temperatures negatively affect the immune function of fish embryos.39–43 For example Jokinen et al.14 stated that low or high temperatures altered the immunological responsiveness in Atlantic salmon. Banerjee and Leptin44 found that UV radiation causes some changes in immune related gene expressions in zebrafish. Similar to previous findings in the present study we found that UV-B exposure and/or different temperatures induced immune related gene expressions. It was reported that some environmental factors such as UV-B and temperature could induce or inhibit the mRNA expression of innate immune-related cytokines in zebrafish embryos.44,45 The alteration of these expressions may lead to inflammation in zebrafish larvae due to the increased number of leucocytes. The main cytokines such as il-1β and tnfα secreted by immune cells are vital in modulating the amplitude of an immune response. Banerjee and Leptin44 revealed that the mRNA levels of il-1β and tnfα increased significantly in the larval zebrafish after exposure to 12 mj cm−2, 24 mj cm−2 and 48 mj cm−2 UV-B. In agreement with these data, in the present study, we found that the mRNA levels of il-1β and tnfα were enhanced in the larval zebrafish after the exposure to UV-B and/or different temperatures, reflecting that UV-B and temperature exhibited their effects on the immune system response during the early development of zebrafish.
In summary, the obtained results indicated that both low and high temperatures enhanced the harmful effects of UV-B on zebrafish development. In particular, low temperature profoundly increased the sensitivity of zebrafish larvae to UV-B exposure. This profound effect occurred due to two reasons. One reason is that low temperature induces a slow development of fish and therefore fish are exposed to UV-B radiation for a long duration.4 Another reason is a reduction in the enzyme-mediated repair of UV-B damaged DNA due to the retardation of all biochemical reactions at low temperatures.46,47
Conclusions
The present study revealed that UV-B exposure in combination with different temperatures caused developmental toxicity in zebrafish embryos–larvae, indicated by increased mortality and malformation, decreased heartbeat rate, and delayed hatching period. Furthermore, the expression of genes that are related to oxidative stress and the innate immune system, including sod1, cat1, hsp70, il-1β, and tnfα, were significantly affected by UV-B exposure and/or different temperatures. In summary, the present work suggested that 24 °C and 30 °C temperatures have additive effects on UV-B induced alterations in the development of zebrafish embryos. Further studies are necessary to better understand and elucidate the mechanisms of toxicity of temperature and UV-B exposure in fish embryos at the biochemical and molecular level.
Conflicts of interest
The authors declare no conflict of interest.
Acknowledgements
The authors would like to thank Saltuk Buğrahan Ceyhun from Fisheries Faculty, Ataturk University for providing zebrafish.
References
- H. U. Dahms and J. S. Lee, Aquat. Toxicol., 2010, 97, 3–14 CrossRef CAS PubMed.
- A. R. Almeida, T. S. Andrade, V. Burkina, G. Fedorova, S. Loureiro, A. M. V. M. Soares and I. Domingues, Ecotoxicol. Environ. Saf., 2015, 122, 145–152 CrossRef CAS PubMed.
- R. C. Rola, L. F. Marins, L. E. M. Nery, C. E. da Rosa and J. Z. Sandrini, Fish Physiol. Biochem., 2014, 40, 1817–1825 CrossRef CAS PubMed.
- E. G. Kazerouni, C. E. Franklin and F. Seebacher, Funct. Ecol., 2016, 30, 584–595 CrossRef.
- T. T. Bizuayehu, S. D. Johansen, V. Puvanendran, H. Toften and I. Babiak, BMC Genomics, 2015, 16 DOI:10.1186/s12864-015-1503-7.
- H. Wang, X. W. Zhu, H. Z. Wang, J. Qiang, P. Xu and R. W. Li, Aquacult. Res., 2014, 45, 259–269 CrossRef CAS.
- S. N. Politis, F. T. Dahlke, I. A. E. Butts, M. A. Peck and E. A. Trippel, J. Exp. Mar. Biol. Ecol., 2014, 459, 70–79 CrossRef.
- E. Realis-Doyelle, A. Pasquet, D. De Charleroy, P. Fontaine and F. Teletchea, PLoS One, 2016, 11 DOI:10.1371/journal.pone.0155487.
- R. Osterauer and H. R. Kohler, Aquat. Toxicol., 2008, 86, 485–494 CrossRef CAS PubMed.
- A. V. Nytro, E. Vikingstad, A. Foss, T. A. Hangstad, P. Reynolds, G. Eliassen, T. A. Elvegard, I. B. Falk-Petersen and A. K. Imsland, Aquaculture, 2014, 434, 296–302 CrossRef.
- W. R. Barrionuevo and W. W. Burggren, Am. J. Physiol. Reg. Integr. Comp. Physiol, 1999, 276, R505–R513 CAS.
- V. Uitregt, O. Vincent, R. S. Wilson and C. E. Franklin, Glob. Change Biol., 2007, 13, 1114–1121 CrossRef.
- R. L. Cramp, S. Reid, F. Seebacher and C. E. Franklin, Biol. Lett., 2014, 10, 20140449 CrossRef PubMed.
- I. E. Jokinen, H. M. Salo, E. Markkula, K. Rikalainen, M. T. Arts and H. I. Browman, Fish Shellfish Immunol., 2011, 30, 102–108 CrossRef CAS PubMed.
- F. Seebacher, E. G. Kazerouni and C. E. Franklin, Biol. Lett., 2016, 12 DOI:10.1098/rsbl.2016.0258.
- Y. X. Jin, Z. Z. Liu, F. Liu, Y. Ye, T. Peng and Z. W. Fu, Neurotoxicol. Teratol., 2015, 48, 9–17 CrossRef CAS PubMed.
- Y. X. Jin, R. J. Chen, W. P. Liu and Z. W. Fu, Fish Shellfish Immunol., 2010, 28, 854–861 CrossRef CAS PubMed.
- H. M. Yang, Y. M. Ham, W. J. Yoon, S. W. Roh, Y. J. Jeon, T. Oda, S. M. Kang, M. C. Kang, E. A. Kim, D. Kim and K. N. Kim, J. Photochem. Photobiol., B, 2012, 114, 126–131 CrossRef CAS PubMed.
- F. R. Abe, J. N. Mendonca, L. A. B. Moraes, G. A. R. de Oliveira, C. Gravato, A. M. V. M. Soares and D. P. de Oliveira, Chemosphere, 2017, 178, 282–290 CrossRef CAS PubMed.
- V. Scheil and H. R. Kohler, Arch. Environ. Contam. Toxicol., 2009, 56, 238–243 CrossRef CAS PubMed.
- S. L. Azevedo, F. Ribeiro, K. Jurkschat, A. M. V. M. Soares and S. Loureiro, Environ. Toxicol. Chem., 2016, 35, 458–467 CrossRef CAS PubMed.
- P. Kern, R. L. Cramp and C. E. Franklin, J. Exp. Biol., 2014, 217, 1246–1252 CrossRef PubMed.
- Q. Dong, K. Svoboda, T. R. Tiersch and W. T. Monroe, J. Photochem. Photobiol., B, 2007, 88, 137–146 CrossRef CAS PubMed.
- C. Pype, E. Verbueken, M. A. Saad, C. R. Casteleyn, C. J. Van Ginneken, D. Knapen and S. J. Van Cruchten, Reprod. Toxicol., 2015, 56, 56–63 CrossRef CAS PubMed.
- A. V. Hallare, M. Schirling, T. Luckenbach, H. R. Kohler and R. Triebskorn, J. Therm. Biol., 2005, 30, 7–17 CrossRef CAS.
- L. C. Felix, E. J. Folkerts, Y. He and G. G. Goss, Environ. Sci.: Nano, 2017, 4, 658–669 RSC.
- B. Sreedevi, G. Suvarchala and G. H. Philip, Indian J. Sci. Res., 2014, 5, 1 CAS.
- T. S. Andrade, J. F. Henriques, A. R. Almeida, A. M. V. M. Soares, S. Scholz and I. Domingues, Environ. Toxicol. Chem., 2017, 36, 682–690 CrossRef CAS PubMed.
- V. Y. Dambal, K. P. Selvan, C. Lite, S. Barathi and W. Santosh, Ecotoxicol. Environ. Saf., 2017, 141, 113–118 CrossRef CAS PubMed.
- G. Suvarchala and G. H. Philip, Environ. Sci. Pollut. Res., 2016, 23, 15515–15523 CrossRef CAS PubMed.
- N. L. Wu, T. A. Lee, S. F. Wang, H. J. Li, H. T. Chen, T. C. Chien, C. C. Huang and C. F. Hung, Exp. Dermatol., 2016, 25, 983–990 CrossRef CAS PubMed.
- D. Livingstone, Mar. Pollut. Bull., 2001, 42, 656–666 CrossRef CAS PubMed.
- P. A. Dennery, Birth Defects Res., Part C, 2007, 81, 155–162 CrossRef CAS PubMed.
- L. Behrendt, M. E. Jönsson, J. V. Goldstone and J. J. Stegeman, Aquat. Toxicol., 2010, 98, 74–82 CrossRef CAS PubMed.
- K. Dale, J. D. Rasinger, K. L. Thorstensen, S. Penglase and S. Ellingsen, Food Chem. Toxicol., 2017, 101, 84–93 CrossRef CAS PubMed.
- R. O. Kim, J. S. Rhee, E. J. Won, K. W. Lee, C. M. Kang, Y. M. Lee and J. S. Lee, Aquat. Toxicol., 2011, 101, 529–539 CrossRef CAS PubMed.
- J. Dorts, E. Falisse, E. Schoofs, E. Flamion, P. Kestemont and F. Silvestre, Sci. Rep., 2016, 6 DOI:10.1038/srep34254.
- P. Y. Lam, E. A. Harvie and A. Huttenlocher, PLoS One, 2013, 8 DOI:10.1371/journal.pone.0084436.
- H. M. Salo, E. I. Jokinen, S. E. Markkula and T. M. Aaltonen, Photochem. Photobiol., 2000, 71, 65–70 CrossRef CAS PubMed.
- E. I. Jokinen, H. M. Salo, S. E. Markkula, A. K. Immonen and T. M. Aaltonen, Photochem. Photobiol., 2001, 73, 505–512 CrossRef CAS PubMed.
- A. C. Cheng, S. A. Cheng, Y. Y. Chen and J. C. Chen, Fish Shellfish Immunol., 2009, 26, 768–772 CrossRef CAS PubMed.
- J. E. Bly and L. W. Clem, Vet Immunol Immunopathol., 1991, 28, 365–377 CrossRef CAS PubMed.
- J. E. Bly, S. M. A. Quiniou and L. W. Clem, Dev. Biol., 1997, 90, 33–43 CAS.
- S. Banerjee and M. Leptin, J. Immunol., 2014, 193, 1408–1415 CrossRef CAS PubMed.
- S. Dios, A. Romero, R. Chamorro, A. Figueras and B. Novoa, Fish Shellfish Immunol., 2010, 29, 1019–1027 CrossRef CAS PubMed.
- K. P. Grant and L. E. Licht, Can. J. Zool., 1995, 73, 2292–2301 CrossRef.
- S. D. Broomhall, W. S. Osborne and R. B. Cunningham, Conserv. Biol., 2000, 14, 420–427 CrossRef.
- J. Hou, L. Li, T. Xue, M. Long, Y. J. Su and N. Wu, Aquat. Toxicol., 2014, 155, 110–118 CrossRef CAS PubMed.
- R. J. Griffitt, R. Weil, K. A. Hyndman, N. D. Denslow, K. Powers, D. Taylor and D. S. Barber, Environ. Sci. Technol., 2007, 41, 8178–8186 CrossRef CAS PubMed.
- U. Oyarbide, I. Iturria, S. Rainieri and M. A. Pardo, Zebrafish, 2015, 12, 71–80 CrossRef CAS PubMed.
- J. H. Jiang, S. G. Wu, C. X. Wu, X. H. An, L. M. Cai and X. P. Zhao, Fish Shellfish Immunol., 2014, 41, 493–500 CrossRef CAS PubMed.
|
This journal is © The Royal Society of Chemistry and Owner Societies 2018 |
Click here to see how this site uses Cookies. View our privacy policy here.