DOI:
10.1039/C8GC00008E
(Communication)
Green Chem., 2018,
20, 1754-1759
Pressurized CO2 as a carboxylating agent for the biocatalytic ortho-carboxylation of resorcinol†
Received
1st January 2018
, Accepted 8th March 2018
First published on 3rd April 2018
Abstract
The utilization of gaseous carbon dioxide instead of bicarbonate would greatly facilitate process development for enzyme catalyzed carboxylations on a large scale. As a proof-of-concept, 1,3-dihydroxybenzene (resorcinol) was carboxylated in the ortho-position using pressurized CO2 (∼30–40 bar) catalyzed by ortho-benzoic acid decarboxylases with up to 68% conversion. Optimization studies revealed tight pH-control and enzyme stability as the most important determinants.
Introduction
Great efforts are currently undertaken to utilize the inexpensive, non-toxic and abundantly available waste gas CO2 as a C1 carbon source for the syntheses of valuable chemicals, materials or fuels.1 However, despite the fact that photosynthetic CO2-fixation mediated by RuBisCO is one of the most dominant reactions in nature, which binds ∼1011 tons CO2 p.a.,2 the chemical activation of CO2 remains challenging due to the high energy input required for substrate activation.1 Recently, the catalytic carboxylation of epoxides using salen complexes, zinc salts and double metal cyanide catalysts opened access to poly(ether)-carbonates for the production of polyurethanes3 and is at the threshold of industrial implementation. Further carboxylation strategies using (transition) metal-4 or organocatalysts5 have been developed to broaden the usability of carbon dioxide, but these methods are still in their infancy regarding commercialization.
The harsh reaction conditions (∼90 bar, 120–300 °C), varying ortho/para-selectivity and incomplete yields are major issues in the large-scale production of salicylic acids via the chemical carboxylation of phenolates using pressurized CO2 gas (Kolbe–Schmitt reaction).6 Although improved by microwave-heating using a bicarbonate-based ionic liquid, the process still suffers from moderate selectivity and yields.7
Biocatalytic methods have been explored as alternatives for the carboxylation of electron-rich (hetero)aromatic compounds to yield the corresponding carboxylic acids.8 Mild reaction conditions, exquisite regioselectivity and excellent yields (e.g. 95% for the bio-carboxylation of resveratrol)9 emphasize the power of bio-carboxylation processes.
However, in the majority of biocatalytic carboxylation protocols reported so far, bicarbonate is used as a CO2 source, which needs to be applied at elevated concentrations (∼3 M) to shift the equilibrium towards the thermodynamically unfavored carboxylation.10 In practice, excess bicarbonate is not only wasteful, but also creates problems during work-up (foaming) upon acidification. In contrast, the use of alternative CO2 sources, such as pressurized or sub/supercritical CO2 for biocatalytic carboxylation is not well investigated. So far, biocatalytic carboxylations were only successful when additional HCO3− (2–3 M) was applied.11 In order to develop an operationally simple protocol amenable to scale-up, the use of pressurized CO2 gas was investigated in the carboxylation of 1,3-dihydroxybenzene (1, resorcinol, Fig. 1a) as a test substrate using 2,3-dihydroxybenzoic acid decarboxylase from Aspergillus oryzae (2,3-DHBD_Ao),12 2,6-dihydroxybenzoic acid decarboxylase from Rhizobium sp. (2,6-DHBD_Rs)13 and salicylic acid decarboxylase from Trichosporon moniliiforme (SAD_Tm),10a which are highly active in the presence of bicarbonate.9–11 Special emphasis was devoted to pressure and pH effects on enzyme stability.
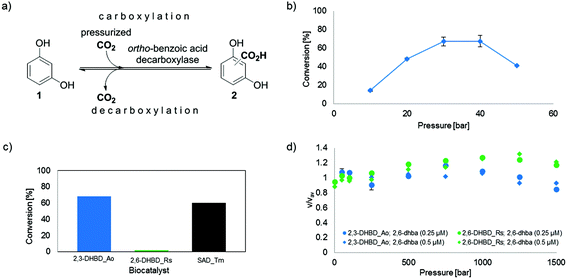 |
| Fig. 1 (a) Enzyme-catalyzed de/carboxylation of resorcinol (1). Carboxylated product 2 is a mixture of regio-isomeric 2,6- (2,6-dhba, 2a) and 2,4-dihydroxybenzoic acid (2,4-dhba, 2b) with a ratio of 3 : 4;10b (b) CO2 pressure dependence of the carboxylation of 1 using 2,3-DHBD_Ao; (c) carboxylation activity of decarboxylases with CO2 (30 bar) using 1 as a substrate; (d) stopped-flow measurements of the decarboxylation of 2,6-dhba (2a) with pressure-pretreated (≤1.5 kbar) 2,3-DHBD_Ao and 2,6-DHBD_Rs. | |
The exposure of enzymes to scCO2‡ pressure has an impact on activity, stability or selectivity,14 which is either due to conformational changes in their secondary and tertiary structure15 or due to the chemical modification of basic amino acid residues (e.g. Lys, Arg, His) by N-carboxylation forming carbamates.16 The most prominent is the carboxylation of lysine residues (e.g. in RuBisCO,17 urease18), which is required for structural reasons (e.g. ligand for binding of metal ions in RuBisCO)17 or even mandatory for catalysis (e.g. β-lactamase OXA-10 from Pseudomonas aeruginosa,19 biotin-dependent enzymes20). In contrast to these rare beneficial effects, the scCO2 treatment of enzymes was reported to cause a decrease or complete loss of enzyme activity due to enforced conformational changes (e.g. horseradish peroxidase,15b lipase,15a tyrosinase15d).
Experimental
General
Resorcinol (1) and 2,6-dihydroxybenzoic acid (2a) were purchased from Sigma Aldrich and 2,4-dihydroxybenzoic acid (2b) was obtained from Fluka Analytical. SYPRO orange was purchased from Invitrogen. The pressure reactor system (DigiCAT-system from the HEL Group, volume 16 mL) was equipped with a gas inlet and a magnetic stirrer. A HisTrapFF column with a Ni-NTA Matrix and a PD10 desalting column were obtained from GE Healthcare and Vivaspin 20 (30 kDa) was obtained from Sartorius AG. CO2 gas (3.0 = 99.9% purity) was obtained from the Linde Group. High pressure stopped-flow measurements were performed with a Hi-Tech Scientific HPSF-56 high pressure stopped-flow spectrophotometer from TgK Scientific. A thermal cycler and a CFX real time system for fluorescence measurements were from Bio Rad and a WebQC calculator was used for pH-calculations.21 2,3-Dihydroxybenzoic acid decarboxylase from Aspergillus oryzae (2,3-DHBD_Ao), 2,6-dihydroxybenzoic acid decarboxylase from Rhizobium sp. (2,6-DHBD_Rs) and salicylic acid decarboxylase from Trichosporon moniliiforme (SAD_Tm) were cloned and overexpressed as previously described.22
General procedure for biotransformation under pressurized CO2 gas
Lyophilized whole cells (90 mg E. coli host cells containing the corresponding overexpressed enzyme with an activity of 5.7 ± 0.7 U mg−1 2,3-DHBD_Ao and 38.1 ± 0.8 U mg−1 2,6-DHBD_Rs, respectively) were rehydrated in TRIS-HCl buffer (2850 μL, pH 9.0, 100 mM) for 30 min. The substrate 1 [10 mM final concentration, dissolved in 150 μL MeOH (5% v/v)] was added to the enzyme solution (3 mL final volume) which was transferred into a pressure reactor. After CO2 gas (30 bar) was applied via an additional gas inlet for ∼1 h, the reaction mixture was stirred at 50 rpm for 24 h at 30 °C in the tightly sealed pressure reactor. After 24 h the reaction was stopped by taking 100 μL of the reaction mixture and diluting it in 900 μL of H2O/MeCN/trifluoroacetic acid (TFA, 50
:
50
:
3) to precipitate the enzyme, which was removed by centrifugation (10 min, 14
000 rpm). The supernatant was directly used for measurements on a reversed-phase HPLC system.
For CO2 pressure studies 10, 20, 30, 40 and 50 bar CO2 gas was applied.
For buffer concentration studies 100, 250 and 500 mM TRIS-HCl buffer was applied.
The CO2 pressure pretreatment experiments with 2,6-DHBD_Rs were performed under the same conditions as described above at 10, 40 and 50 bar CO2 gas (30 mg whole cells, 950 μL TRIS-HCl buffer, pH 9.0, 100 mM), however, without the addition of the substrate. The pressure pretreated enzyme was then used for the decarboxylation of 2,6-dhba 2a [final concentration 10 mM, dissolved in 50 μL MeOH (5% v/v)] in a glass vial (1 mL final volume). The vials were tightly sealed with screw caps and samples were shaken for 24 h, 120 rpm at 30 °C.
For the determination of the enzymatic activity, the lyophilized whole cells of 2,3-DHBD_Ao and 2,6-DHBD_Rs (10 mg mL−1) were rehydrated in TRIS-HCl buffer (950 μL, pH 9.0, 100 mM) for 30 min. The substrate 2a (10 mM final concentration) was added to the enzyme solution (1 mL final volume). The vials were shaken at 30 °C with 120 rpm for 0, 1, 2, 4, 6, 8, 10, 12, 15 and 20 min.
All screening experiments were carried out at least in triplicates and all reactor experiments at least in duplicates.
High pressure stopped-flow system
High pressure stopped-flow measurements were performed under a pressure of 1 bar–1.5 kbar using a purified enzyme (0.5 μM 2,3-DHBD_Ao and 2,6-DHBD_Rs, respectively) in TRIS-HCl buffer (pH 9.0, 100 mM) with two different 2,6-dhba concentrations (0.25 and 0.5 μM 2a, dissolved in 5% v/v MeOH) over 1 min at 30 °C. Spectral changes of the reaction were monitored at 320 nm. All screening experiments were carried out at least in triplicates.
Thermostability experiments
For differential scanning fluorimetry, protein solution [10 μL, 0.2 g L−1 in 5 mM MES (pH 6), 150 mM NaCl], SYPRO orange (10 μL, 1
:
500 diluted in sterile ultrapure water) and multicomponent buffer (pH 4 to 9) (10 μL, 1
:
2
:
2 molar ratio of L-malic acid, MES and TRIS; 1 M total concentration)23 were mixed in 96 well plates. Using a C1000 thermal cycler, the solution was heated at 1.2 °C per minute, from 25 °C to 95 °C. Fluorescence was measured every 0.3 °C, using channel 2 of a CFX real time system. For the smaller step size experiment between pH 4 and 5, sodium citrate buffer (100 mM) was used and the temperature range extended from 10 °C to 95 °C. The melting temperature Tm was calculated as the minimum of the first derivative of the fluorescence vs. the temperature. All experiments were carried out in triplicates.
Analytics
HPLC analysis.
HPLC/UV experiments were performed on a HPLC Agilent 1260 Infinity system with a diode array detector and a reversed phase Phenomenex Luna column C18 (100 Å, 250 × 4.6 mm, 5 μm, column temperature 24 °C). Conversions were determined by comparison with calibration curves for products and substrates prepared with an authentic reference material. All compounds were spectrophotometrically detected at 263 nm. The method was run over 22 min with H2O/TFA (0.1%) as the mobile phase (flow rate 1 mL min−1) and a MeCN/TFA (0.1%) gradient (0–2 min 5%, 2–15 min 5–100%, 15–17 min 100%, 17–22 min 100–5%).
HR-MS analysis.
HR-MS analysis was performed on a nanoHPLC (Ultimate 3000 RSLCnano system – Dionex) system coupled with q-TOF Maxis II-ETD with an ESI-ionisation in positive mode.
Results and discussion
In spite of the previous reports that carboxylation of phenols requires significant concentrations of bicarbonate (∼0.5 M minimum, levelling off at ∼1 M to reach a flat plateau at ∼3 M) to achieve appreciable conversion levels,11 but fails with CO2 (gas) alone,11b we tested the use of commercial sparkling water as a medium for the carboxylation of resorcinol (1) using 2,3-DHBD_Ao. Surprisingly, conversions of 23% and 7% were measured in water samples containing only 55 mM or 4 mM HCO3−, respectively, which favorably compares to 22% obtained under standard conditions (3 M bicarbonate) (for details see the ESI†).
In order to evaluate the usability of CO2 (gas) for carboxylation, the influence of various levels of CO2 pressure (10–50 bar) on the conversion of resorcinol (1, 10 mM, TRIS-HCl buffer 100 mM, pH 9.0) using 2,3-DHBD_Ao12 was determined (Fig. 1b). A bell-shaped curve of the CO2 pressure with an optimum between 30 and 40 bar was found corresponding to a maximum conversion of 68% of carboxylated product (2). The conversion was very low below ≤10 bar and dropped significantly at 50 bar. A time study proved that under these conditions equilibrium was reached at ∼24 h (see ESI, Fig. S3†).
In order to examine whether pressurized CO2 gas (30 bar) is also accepted by other decarboxylases, 2,6-DHBD_Rs13 and SAD_Tm10a were tested (Fig. 1c). While SAD_Tm yielded similar results obtained with 2,3-DHBD_Ao (66% and 60% conv., respectively), 2,6-DHBD_Rs did not lead to an appreciable amount of carboxylated product (2, conv. <2%). This result corroborates a previous observation, that 2,6-DHBD_Rs is inactive under 50–80 bar of CO2.11b
To answer the question whether pressure per se (a physical consequence) or pressurized carbon dioxide (a chemical effect) is responsible for the inactivation of 2,6-DHBD_Rs, high pressure stopped-flow experiments were performed. For reasons of simplicity, the activity of (hydrostatic) pressure-pretreated 2,6-DHBD_Rs was determined in the (energetically favored) decarboxylation direction with 2a as a substrate (Fig. 1d). The fairly constant velocity (v/vav = 0.8–1.3) of substrate consumption (monitored by a decrease of absorbance at 320 nm) of both enzymes pretreated with up to 1.5 kbar reveals their general pressure stability (Fig. 1d, see also ESI, Fig. S5–S7†). Consequently, the inactivation of 2,6-DHBD_Rs can be explicitly assigned to the action of pressurized CO2.
In order to determine whether the CO2 dependent inactivation of 2,6-DHBD_Rs is reversible, the biocatalyst was pretreated with CO2 pressure (10, 40 and 50 bar, respectively) before measuring its decarboxylation activity (Fig. 2a). The sharp drop in conversion between pretreatments with 40 and 50 bar CO2 (92% versus 40% conv.) clearly indicates that 2,6-DHBD_Rs is irreversibly deactivated beyond ∼40 bar CO2.
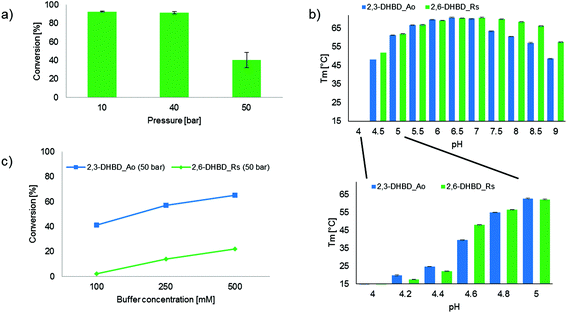 |
| Fig. 2 (a) Decarboxylation activity of CO2 pressure pretreated (10, 40 and 50 bar) 2,6-DHBD_Rs using 2a as a substrate; (b) DSF comparison of the pH dependent melting temperature of 2,3-DHBD_Ao and 2,6-DHBD_Rs in multicomponent buffer (pH 4 to 9) and citrate buffer (pH 4 to 5); (c) Influence of TRIS-HCl buffer concentration on the carboxylation of 1 using 2,6-DHBD_Rs and 2,3-DHBD_Ao under 50 bar CO2 pressure. | |
Since carbamate formation via the carboxylation of lysine residues is a prime suspect for enzyme deactivation, HR-MS measurements were performed. However, no difference in mass between the native and CO2 pressure (50 bar) treated 2,6-DHBD_Rs was detected, thus inactivation is most likely not caused by the carboxylation of basic amino acid residues (see ESI, Fig. S4†).
Carbon dioxide is readily dissolved in the aqueous reaction medium leading to a drop in pH due to the dissociation of H2CO3.24 This effect was applied by Hofland et al.25 using CO2 gas as a ‘volatile acid’ within a range of pH 4–9 to precipitate proteins. To evaluate whether differences in pH-dependent structural stability between 2,3-DHBD_Ao and 2,6-DHBD_Rs could explain their disparate activity, differential scanning fluorimetry (DSF) experiments were performed with both proteins. A first experiment using a multi-component buffer system (L-malic acid, MES, TRIS)23 shows a broad pH-window from pH 4 to 9, while a second run using citrate buffer and smaller increments reveals details within the pH range of 4 to 5. Overall, 2,3-DHBD_Ao and 2,6-DHBD_Rs behave similarly over the whole pH range. Both are thermally most stable between pH 6–7 and show a continuous decrease in denaturation temperature upon higher or lower pH levels (Fig. 2b). Both enzymes are unstable already at room temperature when the pH of the medium reaches below 4.6.
Given that this pH is likely reached in water in a CO2 pressurized system (30 bar CO2 in 0.1 M TRIS-buffer corresponds to a calculated pH of 4.6),21 the influence of the buffer capacity was investigated. An increase of buffer concentration/capacity (TRIS-HCl buffer, 100, 250 and 500 mM) to compensate for acidification due to H2CO3 dissociation and product formation considerably improved the conversion of the carboxylation of 1 with both enzymes (2,3-DHBD_Ao ∼1.5-fold increase; 2,6-DHBD_Rs ∼10-fold increase) (Fig. 2c). These results as well as the DSF analysis clearly indicate that the pH value in the pressure chamber is at the edge of the operational pH-window for both enzymes, with 2,3-DHBD_Ao performing slightly better.
Since the economic usage of resources constitutes an important parameter, the atom economy of various o-carboxylation systems was compared (Table 1, see the ESI†). An excellent atom efficiency of 100% combined with a good yield (68%) verifies the benefit of the biocatalytic approach using CO2 (gas). By way of comparison, the biocatalytic alternative using high amounts of bicarbonate shows a significant drop in atom efficiency (73%), which further drops in the case of traditional chemical (52% and 61%, respectively) or microwave-assisted methods (55%).
Table 1 Comparison of the atom economy of various carboxylation methods of resorcinol (1)
Method |
Yield [%] |
Atom economy [%] |
Ref. |
Biocatalytic (CO2 gas) |
68 |
100 |
This work |
Biocatalytic (HCO3−) |
22 |
73 |
22
|
Chemical (Kolbe–Schmitt) |
56 |
52 |
6b
|
Chemical (Kolbe–Schmitt) |
47 |
61 |
26
|
Chemical (microwave-assisted Kolbe–Schmitt) |
62 |
55 |
7
|
Conclusion
In summary, we have demonstrated that pressurized carbon dioxide can be used directly as a carboxylating agent in the enzyme catalyzed o-carboxylation of a phenol as an alternative to the high concentration of bicarbonate. Two enzyme candidates (2,3-DHBD_Ao and SAD_Tm) readily accepted the alternative CO2 source for the carboxylation of the model substrate resorcinol. In contrast, 2,6-DHBD_Rs was inapplicable under CO2 pressure due to irreversible inactivation, which was correlated to a decrease in pH caused by the dissociation of H2CO3.
Overall, the use of pressurized CO2 gas significantly improves the efficiency of biocatalytic carboxylations and facilitates downstream-processing of this benign and sustainable approach in using CO2 as a carbon feedstock for the synthesis of organic acids.
Conflicts of interest
There are no conflicts of interest to declare.
Acknowledgements
Funding by the Austrian Science Fund (FWF, projects I 1637-N19 and P 26863-N19) is gratefully acknowledged. This work has been supported by the Austrian BMWFJ, BMVIT, SFG, Standortagentur Tirol and ZIT through the Austrian FFG-COMET-Funding Program. Joerg H. Schrittwieser (University of Graz) is cordially thanked for his support regarding the atom efficiency experiments. Special thanks to Stefan E. Payer and Haifeng Liu (University of Graz) for their helpful ideas and fruitful discussions. Ruth Birner-Gruenberger and Laura Liesinger from the Institute of Pathology (Medical University of Graz) are cordially thanked for their support in HR-MS analysis.
References
-
(a) Q. Liu, L. Wu, R. Jackstell and M. Beller, Nat. Commun., 2015, 6, 5933–5948 CrossRef PubMed;
(b) M. Aresta, A. Dibenedetto and A. Angelini, Chem. Rev., 2014, 114, 1709–1742 CrossRef CAS PubMed.
- G. Schneider, Y. Lindqvist and C.-I. Bränden, Annu. Rev. Biophys. Biomol. Struct., 1992, 21, 119–143 CrossRef CAS PubMed.
-
(a) S. Elmas, M. A. Subhani, W. Leitner and T. E. Müller, Beilstein J. Org. Chem., 2015, 11, 42–49 CrossRef PubMed;
(b) J. Langanke, A. Wolf, J. Hofmann, K. Böhm, M. A. Subhani, T. E. Müller, W. Leitner and C. Gürtler, Green Chem., 2014, 16, 1865–1870 RSC.
-
(a) T. Fujihara and Y. Tsuji, J. Jpn. Pet. Inst., 2016, 59, 84–92 CrossRef CAS;
(b) K. Huang, C.-L. Sun and Z.-J. Shi, Chem. Soc. Rev., 2011, 40, 2435–2452 RSC.
-
(a) V. Saptal, D. B. Shinde, R. Banerjee and B. M. Bhanage, Catal. Sci. Technol., 2016, 6, 6152–6158 RSC;
(b) B. A. Vara, T. J. Struble, W. Wang, M. C. Dobish and J. N. Johnston, J. Am. Chem. Soc., 2015, 137, 7302–7305 CrossRef CAS PubMed;
(c) C. J. Whiteoak, A. Nova, F. Maseras and A. W. Kleij, ChemSusChem, 2012, 5, 2032–2038 CrossRef CAS PubMed.
-
(a) A. S. Lindsey and H. Feskey, Chem. Rev., 1957, 57, 583–614 CrossRef CAS;
(b)
S. S. Mathur, A. Vijayan, V. H. Nougare, S. R. Bhosale, M. K. Dapake, S. D. Parkar, P. D. Damania, N. S. Jagtap, A. Kalirajan, R. H. Khamkar, N. J. Jain, M. M. More, S. B. Aher and S. S. Padwal, Processes for the preparation of bispyribac sodium and intermediates thereof, WO 2014128719A2; CAN, 2014, 161, 390016 Search PubMed.
- A. Stark, S. Huebschmann, M. Sellin, D. Kralisch, R. Trotzki and B. Ondruschka, Chem. Eng. Technol., 2009, 32, 1730–1738 CrossRef CAS.
-
(a)
R. Lewin, M. L. Thompson and J. Micklefield, Enzyme Carboxylation and Decarboxylation, in Science of Synthesis: Biocatalysis, in Organic Synthesis, ed. K. Faber, W.-D. Fessner and N. J. Turner, Thieme, Stuttgart, New York, 2015, vol. 2, pp. 133–157 Search PubMed;
(b) A. Alissandratos and C. J. Easton, Beilstein J. Org. Chem., 2015, 11, 2370–2387 CrossRef CAS PubMed;
(c) S. M. Glueck, S. Gümüs, W. M. F. Fabian and K. Faber, Chem. Soc. Rev., 2010, 39, 313–328 RSC.
- K. Plasch, V. Resch, J. Hitce, J. Popłoński, K. Faber and S. M. Glueck, Adv. Synth. Catal., 2017, 359, 959–965 CrossRef CAS PubMed.
-
(a) K. Kirimura, H. Gunji, R. Wakayama, T. Hattori and Y. Ishii, Biochem. Biophys. Res. Commun., 2010, 394, 279–284 CrossRef CAS PubMed;
(b) C. Wuensch, J. Gross, G. Steinkellner, A. Lyskowski, K. Gruber, S. M. Glueck and K. Faber, RSC Adv., 2014, 4, 9673–9679 RSC.
-
(a) T. Matsui, T. Yoshida, T. Yoshimura and T. Nagasawa, Appl. Microbiol. Biotechnol., 2006, 73, 95–102 CrossRef CAS PubMed;
(b) L. Pesci, S. M. Glueck, P. Gurkov, I. Smirnova, K. Faber and A. Liese, FEBS J., 2015, 282, 1334–1345 CrossRef CAS PubMed;
(c) T. Matsuda, Y. Ohashi, T. Harada, R. Yanagihara, T. Nagasawa and K. Nakamura, Chem. Commun., 2001, 2194–2195 RSC.
- R. Santha, N. A. Rao and C. S. Vaidyanathan, Biochim. Biophys. Acta, 1996, 1293, 191–200 CrossRef.
-
(a) M. Yoshida, N. Fukuhara and T. Oikawa, J. Bacteriol., 2004, 186, 6855–6863 CrossRef CAS PubMed;
(b) M. Goto, H. Hayashi, I. Miyahara, K. Hirotsu, M. Yoshida and T. Oikawa, J. Biol. Chem., 2006, 281, 34365–34373 CrossRef CAS PubMed.
-
(a) S. Cantone, U. Hanefeld and A. Basso, Green Chem., 2007, 9, 954–971 RSC;
(b) S. Garcia, N. M. T. Lourenço, D. Lousa, A. F. Sequeira, P. Mimoso, J. M. S. Cabral, C. A. M. Afonsoc and S. Barreiros, Green Chem., 2004, 6, 466–470 RSC.
-
(a) D. Chen, H. Zhang, J. Xu and Y. Yan, Enzyme Microb. Technol., 2013, 53, 110–117 CrossRef CAS PubMed;
(b) F. Gui, F. Chen, J. Wu, Z. Wang, X. Liao and X. Hu, Food Chem., 2006, 97, 480–489 CrossRef CAS;
(c) X. Liao, Y. Zhang, J. Bei, X. Hu and J. Wu, Innovative Food Sci. Emerging Technol., 2009, 10, 47–53 CrossRef CAS;
(d) W. Hu, Y. Zhang, Y. Wang, L. Zhou, X. Leng, X. Liao and X. Hu, Langmuir, 2010, 27, 909–916 CrossRef PubMed.
- M. L. Drummond, A. K. Wilson and T. R. Cundari, J. Phys. Chem. B, 2012, 116, 11578–11593 CrossRef CAS PubMed.
- M. Oliva, V. S. Safont and J. Andres, J. Phys. Chem. A, 1999, 103, 8725–8732 CrossRef CAS.
- E. Jabri, M. Carr, R. Hausinger and P. Karplus, Science, 1995, 268, 998–1004 CAS.
- J. Li, J. B. Cross, T. Vreven, S. O. Meroueh, S. Mobashery and H. B. Schlegel, Proteins: Struct., Funct., Bioinf., 2005, 61, 246–257 CrossRef CAS PubMed.
- P. V. Attwood and J. C. Wallace, Acc. Chem. Res., 2002, 35, 113–120 CrossRef CAS PubMed.
- pH Calculator – Calculates pH of a Solution, https://www.webqc.org/pHsolver.php, accessed 02/22/2018.
- C. Wuensch, S. M. Glueck, J. Gross, D. Koszelewski, M. Schober and K. Faber, Org. Lett., 2012, 14, 1974–1977 CrossRef CAS PubMed.
- J. Newman, Acta Crystallogr., Sect. D: Biol. Crystallogr., 2004, 60, 610–612 CrossRef PubMed.
-
(a) Z. Duan and R. Sun, Chem. Geol., 2003, 193, 257–271 CrossRef CAS;
(b) A. C. Pierre, ISRN Chem. Eng., 2012 Search PubMed , 753687.
- G. W. Hofland, A. de Rijke, R. Thiering, L. A. M. van der Wielen and G.-J. Witkamp, J. Chromatogr. B: Biomed. Sci. Appl., 2000, 743, 357–368 CrossRef CAS.
- D. K. Hale, A. R. Hawdon, J. I. Jones and D. I. Packham, J. Chem. Soc., 1952, 3503–3509 RSC.
Footnotes |
† Electronic supplementary information (ESI) available. See DOI: 10.1039/c8gc00008e |
‡ scCO2 = supercritical carbon dioxide. |
|
This journal is © The Royal Society of Chemistry 2018 |