DOI:
10.1039/C7FO01388D
(Paper)
Food Funct., 2018,
9, 594-603
Production, optimisation and characterisation of angiotensin converting enzyme inhibitory peptides from sea cucumber (Stichopus japonicus) gonad†
Received
7th September 2017
, Accepted 9th December 2017
First published on 11th December 2017
Abstract
In this study, production of bioactive peptides with angiotensin converting enzyme (ACE) inhibitory activity from sea cucumber (Stichopus japonicus) gonad using commercial protamex was optimised by response surface methodology (RSM). As a result, the optimal condition to achieve the highest ACE inhibitory activity in sea cucumber gonad hydrolysate (SCGH) was hydrolysis for 1.95 h and E/S of 0.75%. For further characterisation, three individual peptides (EIYR, LF and NAPHMR) were purified and identified. The peptide NAPHMR showed the highest ACE inhibitory activity with IC50 of 260.22 ± 3.71 μM. NAPHMR was stable against simulated gastrointestinal digestion and revealed no significant cytotoxicity toward Caco-2 cells. Molecular docking study suggested that Arg, His and Asn residues in NAPHMR interact with the S2 pocket or Zn2+ binding motifs of ACE via hydrogen or π-bonds, potentially contributing to ACE inhibitory effect. Sea cucumber gonad is thus a potential resource to produce ACE inhibitory peptides for preparation of functional foods.
1. Introduction
Angiotensin converting enzyme (ACE, EC 3.4.15.1) plays a central physiological role in the regulation of blood pressure as it promotes the conversion of angiotensin I to angiotensin II in the circulatory or endocrine of human renin–angiotensin system.1In vivo, a high level of ACE activity may be involved in genetic predisposition to high blood pressure.2 Hence, ACE is identified as a potential target for synthetic pharmaceuticals, and the inhibition of its over-activity is expected to reduce blood pressure. Compared with pharmacological agents, peptides with ACE inhibitory activity derived from natural resources have no side effects or symptoms and are preferred by consumers.3 In this regard, naturally occurring ACE inhibitory peptides are proposed as ideal functional food supplements for human health. Such peptides have attracted increasing attention from researchers, particularly the utilisation of by-products from seafood processing for peptide preparation.1
Sea cucumber (Stichopus japonicus) is an important cultured and consumed aquatic species in Asian countries owing to its high nutritional and pharmacological value.4 In 2016, its total production reached 204
359 tons in China.5 During sea cucumber transportation and processing, autolysis frequently occurs due to disturbance by environmental changes or mechanical stress. Endogenous proteinases that exist in the viscera are primarily responsible for autolysis.6 Thus, evisceration is essential for sea cucumber processing to avoid quality deterioration.7 These intestinal constituents as low-value by-products are usually processed into fishmeal for animal feed.1 Among intestinal constituents, the gonad is a protein-rich resource since its protein content is 51.2% (dry basis), which is two-fold higher than that of digestive tract protein.8 However, little information regarding the utilisation of the gonad is available. Considering its high protein content, preparation of gonad hydrolysates as potential functional food supplements would be promising.
Till now, sea cucumber body wall hydrolysate is reported to exhibit many biological activities, such as antioxidant, anti-hypertension and anti-inflammation.3,9 In addition, antioxidant activities are observed in the hydrolysates of sea cucumber gonad,10 viscera,11 and roe.12 Metal chelating activity has been identified in ovum hydrolysate.13 However, to the best of our knowledge, using sea cucumber gonads to prepare bioactive peptides with ACE inhibitory activity has not been reported yet.
Therefore, in the present study, we attempted to optimise the hydrolysis conditions of sea cucumber gonads for preparation of ACE inhibitory peptides. The main ACE inhibitory peptides were isolated and identified to investigate their inhibitory function. In addition, the peptide with the highest ACE inhibitory was synthesised to characterise its stability during simulated digestions by gastrointestinal enzymes. The cytotoxicity of the peptide toward Caco-2 cells was investigated, and its potential binding sites with ACE were simulated by molecular docking.
2. Materials and methods
2.1 Materials
Sea cucumbers (Stichopus japonicus) with body weight of 150–200 g were purchased alive from an aquaculture base in Quangang (Fujian, China). After being transported to the laboratory in 2 h, the sea cucumbers were anesthetised by ice water treatment before sacrificing. Then, the gonads were stripped from the viscera and used for enzymatic hydrolysis or stored at −20 °C until use.
ACE (EC 3.4.15.1, porcine kidney, ≥10 units per mg protein), hippuryl-histidyl-leucine (HHL) and 3-(4,5-dimethylthiazol-2-yl)-2,5-diphenyltetrazolium bromide (MTT) were purchased from Sigma-Aldrich Company (St Louis, MO, USA). Protamex (1.5 AU g−1) were obtained from Novozymes Biotechnology Company (Tianjin, China). Caco-2 cell lines (human colorectal adenocarcinoma) were obtained from Boster Biological Technology Company (Wuhan, China). Iscove's modified Dulbecco's medium (IMDM) and fetal bovine serum (FBS) were obtained from Thermo Fisher Scientific (Rochester, NY, USA). All other reagents used were of analytical grade.
2.2 Preparation of sea cucumber gonad hydrolysate
In order to obtain bioactive peptides, 100 g sea cucumber gonad was hydrolysed by protamex. Briefly, gonads were chopped and homogenised with 2 volumes (w/v) of distilled water. The slurry was mixed with 25 mM phosphate buffer (pH 6.5) and pre-incubated at 45 °C; commercial protamex was then added in the desired enzyme/substrate (E/S) ratio (v/v). Sequentially, the protamex was inactivated by heating at 95 °C for 10 min after the desired hydrolysis time (h). Hydrolysate was cooled in ice water to room temperature and centrifuged at 10
000g for 30 min. Then, the supernatant was carefully collected and dispersed into 2 volumes of ethanol in order to precipitate polysaccharides. The obtained suspension was then concentrated by sufficient vacuum evaporation at 40 °C using a rotary evaporator to remove ethanol and stored at −20 °C until use.
2.3 Experimental design, modeling and optimisation
After determining the preliminary range of the two hydrolysis variables (hydrolysis time and E/S ratio) through a single-factor test, a central composite design (CCD) with these two independent variables at five levels was generated to optimise and produce the maximum ACE inhibitory activity peptides from sea cucumber gonad hydrolysate (SCGH) using response surface methodology (RSM).14 The complete design consisted of 13 independent experimental points (4 factorial points, 4 axial points and 5 center points), and measurements were carried out in triplicate for all individual experiments in a random order. Design Expert software (Version 7.0.0, Stat-Ease Inc., Minneapolis, USA) was used for multiple regression and variance analysis. The fitted polynomial equation was expressed as the 3D response surface and 2D contour plots in order to visualise the relationship between the response and the experimental levels of each variable.
2.4 Determination of ACE inhibitory activity
ACE inhibitory activity of SCGH was measured according to the method reported by Chen et al.15 Briefly, 50 μL sample was mixed with 50 μL ACE solution (25 munits per mL) and pre-incubated at 37 °C for 5 min. Then, 125 μL of the substrate (8.3 mM HHL in 50 mM sodium borate buffer containing 0.5 M NaCl, pH 8.3) was added into the mixture to initiate reaction. After incubation for 60 min, the reaction was terminated by the addition of 125 μL 1 M HCl, and 750 μL ethyl acetate was added to extract the hippuric acid (HA) produced. After centrifugation (1000g for 5 min), the upper layer (500 μL) was transferred and evaporated at room temperature in vacuum until ethyl acetate was removed completely. The extracted HA was then dissolved in 1.5 mL distilled water and the absorbance was measured at 228 nm using a UV spectrophotometer. The ACE inhibitory activity was calculated by the following formula:
Inhibition of ACE activity (%) = (A1 − A2)/(A1 − A0)% |
where A1 and A2 indicate HA content generated without and with ACE inhibitor and A0 is the HA content generated without ACE. The potency of the ACE inhibitory activity of a sample was expressed by the IC50 value, which is defined as the concentration of peptide mediating 50% inhibition of ACE activity and is determined by logarithmic regression analysis. Each sample was tested in triplicate.
2.5 Determination of the degree of hydrolysis
The degree of hydrolysis (DH) of SCGH was determined using the o-phthaldialdehyde (OPA) method. Briefly, the OPA reagent was prepared according to the report of Nielsen et al.16 Then, the OPA assay was carried out by the addition of 400 μL of sample to 3 mL of OPA reagent. After gently vortexing for 5 s, the mixture was allowed to stand for 2 min. Then, the absorbance of the mixture was measured at 340 nm to calculate free amino group content as equivalent to that obtained in a standard curve prepared using L-serine. Total amount of the free amino groups in sea cucumber gonads was obtained following acid hydrolysis with 6 M HCl at 110 °C for 24 h.
2.6 Determination of molecular weight distribution
Molecular weight (MW) distribution of SCGH was determined by gel permeation chromatography (GPC) using an Agilent 1260 high-performance liquid chromatography (HPLC) system (CA, USA) equipped with a TSK-gel G 2000 SWXL column (7.8 mm I.D. × 300 mm) (TOSOH, Yamaguchi, Japan).13 Samples were eluted with 0.1 M phosphate buffer (pH 6.7) containing 0.1 M Na2SO4 at a flow rate of 0.5 mL min−1. The column was calibrated with L-cysteine (240 Da), HHL (430 Da), neurotensin (1673 Da), a synthetic peptide (6640 Da) and parvalbumin (11
950 Da) prepared in our laboratory. The data was analysed with GPC software (Agilent, Palo Alto, USA).
2.7 Fractionation and purification of ACE inhibitory peptides
SCGH was filtered sequentially through 10 kDa and 3 kDa MW cutoff membranes (Millipore, USA) and separated into three fractions: fraction I (MW > 10 kDa), fraction II (MW 3–10 kDa) and fraction III (MW < 3 kDa). Based on the ACE inhibitory activity, the concentrated fraction III with the highest activity was loaded onto a Sephadex G-15 column (1.5 cm × 100 cm) and eluted with distilled water at a flow rate of 0.5 mL min−1. The highest ACE inhibitory fraction was pooled, lyophilised and further purified using RP-HPLC on a Zorbax SB-C18 column (4.6 mm I.D. × 250 mm, Agilent, USA) followed by Zorbax SB-C8 column (4.6 mm I.D. × 250 mm, Agilent, USA). The resulting purified peptide was collected for amino acid sequence analysis.
2.8 Peptide identification by MALDI-TOF-MS/MS
The amino acid sequences of purified peptides were analysed by mass spectroscopy (MS) analysis with a time-of-flight/time-of-flight (TOF/TOF) 5800 mass spectrometer (Applied Biosystems, SCIEX, USA). The mass spectra were then used for manual de novo sequencing using Data Explore software (Applied Biosystems SCIEX, USA).
2.9 Peptide synthesis
After identification by MALDI-TOF-MS/MS, a peptide (NAPHMR) with a purity of 98.9% was synthesised by Cellmano Biotech Co. Ltd (Hefei, China).
2.10 Simulated gastrointestinal digestion analysis
Synthetic peptide (NAPHMR) was subjected to a two-stage hydrolysis process with pepsin and pancreatin, as described by Huang et al.,17 with some modifications. The simulated gastric fluid (SGF) and simulated intestinal fluid (SIF) were prepared as described in the United States Pharmacopoeia.18 Briefly, for SGF digestion (the first stage) stability assay, the peptide (1 mg mL−1) was mixed with 10-fold SGF, which consists of 2 μg mL−1 porcine pepsin (>250 U mg−1, Sigma) in 35 mM NaCl at pH 1.2 (1
:
50 pepsin to peptide, w
:
w). The SGF digestion was performed at 37 °C with continuous rocking for 1 h and the reaction was immediately terminated by addition of 30 μL 200 mM Na2CO3. For SIF digestion (the secondary stage) stability assay, the peptide hydrolysed by SGF was continuously digested by addition of 10-fold SIF, which consists of 2 μg mL−1 pancreatin (8× USP specifications, Sigma) in 0.05 M phosphate buffer (pH 7.5) (1
:
50 pancreatin to peptide, w
:
w). The SGF digestion was performed at 37 °C for 4 h with continuous rocking and the reaction was stopped by heating at 95 °C for 10 min. The peptide and its hydrolysates obtained after SGF and SIF digestion were analysed by RP-HPLC and the corresponding ACE inhibitory activities were further determined.
2.11 Cytotoxicity assay
The cytotoxicity of NAPHMR toward Caco-2 cells was performed, as described by Ding et al.,19 using the MTT assay. Caco-2 cells with density of 1 × 105 cells per mL were grown in 96-well culture plates and incubated with peptide NAPHMR with different final concentrations of 1–5 mM. After co-culturing at 37 °C in 5% CO2 for 24 h, 20 μL of MTT (5 mg mL−1) was added and incubated for 4 h. Subsequently, 150 μL of dimethyl sulfoxide was added to dissolve formazan crystals and the absorbance was measured at 595 nm using a multimode plate reader (Tecan Infinite M200 Pro, Switzerland). The cytotoxic effect of the peptide to Caco-2 cells was expressed as the percentage of cell viability calculated according to the absorbance at 595 nm.
2.12 Molecular docking of NAPHMR on ACE binding site
Molecular docking was used to predict the interaction of small molecules (peptide) with receptors (ACE). Before docking, the crystal structure of human ACE (PDB: 1O86A) was obtained from the Protein Data Bank (PDB) and the structure of NAPHMR was constructed. The flexible docking of NAPHMR at the ACE-binding site was performed using Discovery Studio 2.5 software to explore their interaction mechanism.
2.13 Statistical analysis
Statistical analysis was performed using a Statistical Package for Social Science (SPSS, software version 17.0). All data were represented as mean ± standard deviation.
3. Results and discussion
3.1 Optimisation of hydrolysis parameters of sea cucumber gonads
According to preliminary study, among commercial proteinases, protamex is more efficient and suitable for digestion of gonad proteins to produce ACE inhibitory peptides than others; therefore, it was chosen to hydrolyse sea cucumber gonad proteins. The hydrolysis time and E/S ratio were determined as two relevant factors that influence the ACE inhibitory activities based on the results of single-factor designs (data not shown). These two variances were further optimised using RSM to obtain the maximum ACE inhibitory-active peptides from SCGH. As shown in Fig. 1A, ACE inhibitory activities significantly increased with alterations of these two variables in the 3D response surface plots. In addition, their 2D contours were elliptical (Fig. 1B) and the effects with p values were lower than 0.05 (Table 1), indicating that the variables of hydrolysis time and E/S ratio were significant model terms for producing ACE inhibitory peptides and their mutual interaction was also significant.
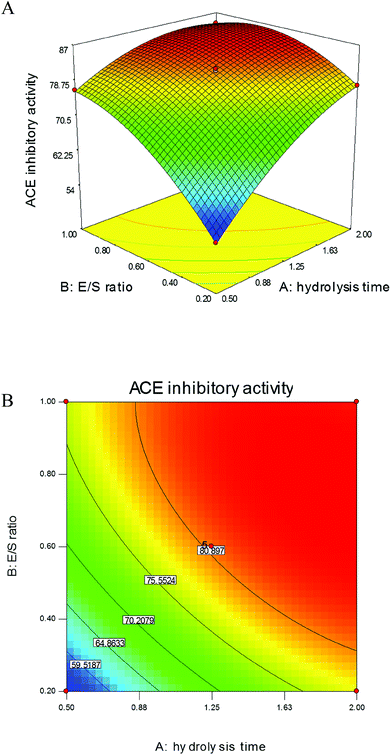 |
| Fig. 1 The effect of variables on ACE inhibitory activities of SCGH using RSM. The 3D response surface plots (A) and the corresponding 2D contours (B) showing the combined effect of hydrolysis time and E/S ratio on ACE inhibitory activity. | |
Table 1 Analysis of variance (ANOVA) for fitted quadratic polynomial regression model for optimisation of ACE inhibitory activity production derived from SCGH
Source |
Sum of squares |
df |
Mean square |
F value |
p value |
Model |
1180.19 |
5 |
236.04 |
4090.33 |
<0.0001 |
Hydrolysis time |
490.94 |
1 |
490.94 |
8507.64 |
<0.0001 |
E/S ratio |
415.54 |
1 |
415.54 |
7200.95 |
<0.0001 |
Hydrolysis time × E/S ratio |
62.41 |
1 |
62.41 |
1081.52 |
<0.0001 |
Hydrolysis time2 |
88.76 |
1 |
88.76 |
1538.13 |
<0.0001 |
E/S ratio2 |
148.93 |
1 |
148.93 |
2580.89 |
<0.0001 |
Residual |
0.40 |
7 |
0.058 |
|
|
Lack of fit |
0.17 |
3 |
0.056 |
0.96 |
0.4932 |
Pure error |
0.23 |
4 |
0.059 |
|
|
Total |
1180.59 |
12 |
|
|
|
Based on multiple regression analysis of the above model, corresponding parameters were calculated and the equation to predict response ACE inhibitory activity of SCGH is expressed as follows:
Y = 27.29 + 34.22A + 69.18B − 13.17A × B − 6.35A2 − 28.92B2 |
where
Y is the predicted response of ACE inhibitory activity, and
A and
B are the actual variables for hydrolysis time and E/S ratio, respectively.
The analysis of variance (ANOVA) for second-order polynomial model fitted to the response surface revealed a non-significant value (0.96) for the above model equation, indicating that the regression equation and fitted model were appropriate for predicting the response of ACE inhibitory activity. Thus, the proposed conditions to yield the maximum ACE inhibitory activity were calculated using the above model equation with hydrolysis time of 1.95 h and E/S ratio of 0.75%. Under these conditions, ACE inhibitory activity reached 86.97 ± 1.55% and the DH was 17.6 ± 0.34%, which is close to the predicted value of 86.23%. These values were higher than those for hydrolysates obtained from sardinelle by-products treated with alkaline proteinase (DH of 8% and ACE inhibition of 63.2%)20 and cuttlefish by-products treated with enzyme extracts from Bacillus licheniformis NH1 (DH of 13.5% and ACE inhibition of 79%).21 The results further confirmed that the regression model was adequate to reflect the expected optimisation required to produce high ACE inhibitory-active peptides from sea cucumber gonad proteins.
3.2 Separation and purification of ACE inhibitory peptides
3.2.1 Fractionation of SCGH by ultrafiltration.
Ultrafiltration was performed to separate SCGH prepared under the optimal hydrolysis conditions into three fractions according to MW: fraction I (MW > 10 kDa), fraction II (10 kDa > MW > 3 kDa) and fraction III (MW < 3 kDa). As shown in Fig. 2A, compared with the ACE inhibitory activity of the whole SCGH (IC50 = 3.98 ± 0.18 mg mL−1), fraction III revealed the highest ACE inhibitory activity (IC50 = 1.32 ± 0.04 mg mL−1), while fraction I showed the lowest activity (IC50 = 6.80 ± 0.16 mg mL−1). The MW distribution of fraction III was further investigated (Fig. 2B), which showed that the relative proportion of MW lower than 1000 Da was more than 95% (Fig. 2C). The results suggested that peptide with lower MW exhibited stronger ACE inhibitory activity than peptides with higher MW. This tendency was also observed in peptides derived from grass carp protein, where the fraction with MW < 3 kDa showed stronger ACE inhibitory activity than other fractions with higher MW.15 This may be explained by the result that peptide with lower MW can preferentially pass through the gap and bind with the active sites of ACE.1 In addition, as an orally administered peptide, it was proposed that the lower MW peptide is more resistant to hydrolysis by various proteinases and is more readily absorbed into the intestinal tissue and transported to the site of action in vivo.19 Hence, the lower MW fractions from SCGH (fraction III) are worthy of investigation in the following experiments.
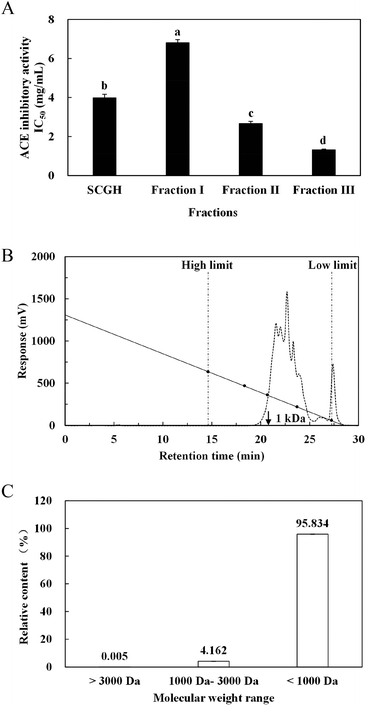 |
| Fig. 2 ACE inhibitory activities and MW distribution of fractions in SCGH. (A) ACE inhibitory activities of SCGH and its three fractions after ultrafiltration (fraction I, MW > 10 kDa; fraction II, 10 kDa > MW > 3 kDa; fraction III, MW < 3 kDa). MW distribution profile (B) and corresponding relative content of different MW ranges (C) of fraction III (MW < 3 kDa) following peptide gel filtration chromatography. The oblique line represents the standard curve of MW distribution (240 Da–11 950 Da). | |
3.2.2 Isolation of peptides from fraction III by gel filtration chromatography and RP-HPLC.
Fraction III was thus pooled and further purified through Sephadex G-15 gel chromatography. As shown in Fig. 3A, six main fractions were obtained, of which FIII-1 showed the highest ACE inhibitory activity with IC50 of 1.03 ± 0.10 mg mL−1 (Fig. 3B). Hence, FIII-1 was chosen for further purification by RP-HPLC in a C18 column. The RP-HPLC profile of FIII-1 is shown in Fig. 3C and 14 peaks for ACE inhibitory activity were identified. Each fraction separated from FIII-1 was then examined using a C8 column, and only a single peak was observed for FIII-1–11, FIII-1–13 and FIII-1–14 (Fig. 3E, F and G), while their ACE inhibitory activities (IC50) were 0.22 ± 0.01 mg mL−1, 0.10 ± 0.01 mg mL−1 and 0.17 ± 0.01 mg mL−1, respectively (Fig. 3D). Because of their high ACE inhibitory activity and purity, these fractions were individually collected and then their amino acid sequences were analysed by MALDI-TOF-MS/MS.
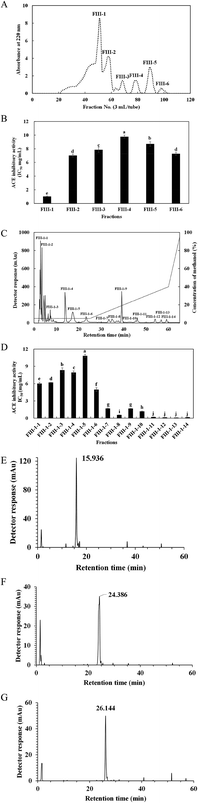 |
| Fig. 3 Purification of peptides from fraction III using multiple column chromatographies. Sephadex G-15 gel chromatography profile of fraction III (A) and ACE inhibitory activity of each fraction (B). Chromatography of FIII-1 from Sephadex G-15 column loaded on Zorbax C18 column by RP-HPLC with the linear gradient of methanol (5–95% in 60 min) containing 0.1% TFA at a flow rate of 1 mL min−1 (C) and ACE inhibitory activities of 14 fractions (FIII-1–1 to FIII-1–14) (D). FIII-1–11 (E), FIII-1–13 (F) and FIII-1–14 (G) were examined separately by Zorbax C8 column with the same conditions as those used in the Zorbax C18 column. Small letters on the bars represent significant difference (p < 0.05). | |
3.3 Characterisation of purified peptides
Through MALDI-TOF-MS/MS analysis, the obtained amino acid sequences of FIII-1–11, FIII-1–13 and FIII-1–14 were EIYR (MW = 633 Da), LF (MW = 279 Da) and NAPHMR (MW = 725 Da), respectively (Fig. 4A, B and C). The IC50 of NAPHMR was 234.48 μM (0.17 mg mL−1), which showed the highest activity among three individual peptides, while the IC50 values of EIYR and LF were 347.55 μM (0.22 mg mL−1) and 358.42 μM (0.10 mg mL−1), respectively. It was noticed that all identified peptides are oligopeptides, which is in agreement with the reports that ACE inhibitory peptides usually contain 2–12 amino acids with MW ranging from 150 to 800 Da.1 Crystallography study also supported the data as the active site of ACE cannot accommodate peptides with higher MWs.22
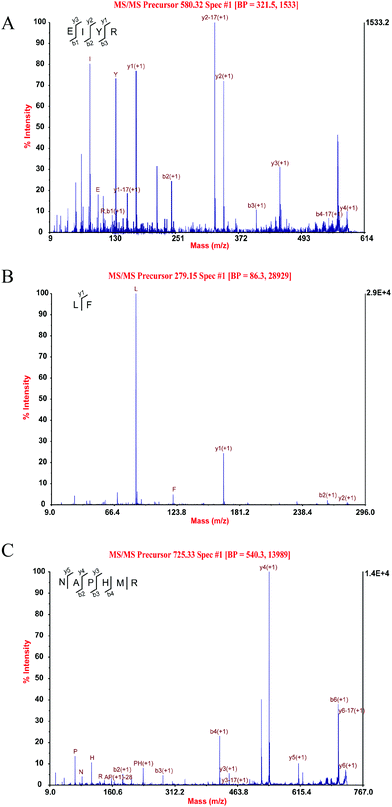 |
| Fig. 4 Three individual peptides from SCGH identified by MALDI-TOF-MS/MS. MS/MS spectra of EIYR (A), LF (B), NAPHMR (C). | |
The identified peptide of LF with IC50 of 358.42 μM is a reverse sequence of FL, which was identified in salmon muscle hydrolysate with IC50 of 383.2 μM,23 indicating that for dipeptides, amino acid composition might majorly affect its ACE inhibitory activity rather than their sequential orders. For tripeptides or longer peptides, their structure–activity correlation studies indicated that ACE binding is strongly influenced by the C-terminal amino acid residue.2 It is reported that the ACE preferred substrates contain Arg residue at the C-terminal position due to the positive charge of guanidine group in side chain of Arg, and this structural property contributes substantially to its inhibitory potency.2 This was supported by the data that the IC50 of peptide LHLPLR was 1.8 μM, which was twice more potent than that of LHLPLP.19,24 Hence, the existence of Arg residue in the C-terminal of EIYR and NAPHMR might contribute to their potent ACE inhibitory activities. Moreover, our present study also showed that the peptide NAPHMR having Asn at the N-terminal is similar to the peptide NMAINPSK from α-casein with IC50 of 60 μM
25 and NLHLPLPLL from β-casein with IC50 of 15 μM.26 Asn residue potentially contributes to the ACE inhibitory activity of NAPHMR due to its unique amide group that C
O group act as a hydrogen bond donor or NH2 group as hydrogen bond acceptor.27
For the peptides identified from SCGH, two peptides showing the egg coat matrix protein (GenBank: BAJ41225.1) sequence (EIYR and LF) as well as one peptide showing the major yolk proteins (GenBank: BAH79576.1 and BAH79577.1) sequence (NAPHMR) were characterised. The relatively low homology of major yolk proteins has been reported among echinoderm species.28 Study on Japanese common sea cucumber showed that major yolk protein 1 and 2 share a 58.2% identity with each other and only share about 29% identity with the major yolk protein of sea urchins.29 Therefore, NAPHMR was quite probably derived from the major yolk protein of sea cucumber gonads, although they share merely 66.7% identity. In consideration of major yolk proteins as a major and important protein in echinoderm gonads,28 NAPHMR with relatively high ACE inhibitory activity was thus synthesised and validated in the following experiments.
3.4 Simulation of gastrointestinal digestion and cytotoxic effect to Caco-2 cells
In vivo, the potential antihypertensive effect of ACE inhibitory peptides has been proposed to depend on the ability to be released after gastrointestinal digestion and then, to reach their target sites at the luminal side of the intestinal tract or in peripheral organs after absorption.19,24 In this regard, the resistance to gastrointestinal digestion was considered as an important factor to evaluate the potent ACE inhibitory activity. With the aim of identifying the fragments released by digestive enzymes, synthetic peptide NAPHMR was subjected to a two-stage digestion under simulated physiological conditions. The RP-HPLC chromatograms of NAPHMR before and after simulated gastrointestinal digestion are shown in Fig. 5A–C. No visible change in NAPHMR was noticed in the main fragment of the final hydrolysate. Similar to LHLPLP, which was stable in gastrointestinal enzymes,24 proline residue in NAPHMR might potentially make it less susceptible to proteolytic enzymes. Correspondingly, no significant decrease in ACE inhibitory activity occurred after gastric digestion (IC50 = 252.15 ± 7.64 μM) and intestinal digestion (IC50 = 253.65 ± 10.50 μM) compared with the activity before digestion (IC50 = 260.22 ± 3.71 μM) (p < 0.05). These results indicated that NAPHMR possess better resistance to gastrointestinal digestion and is appropriate for oral administration.
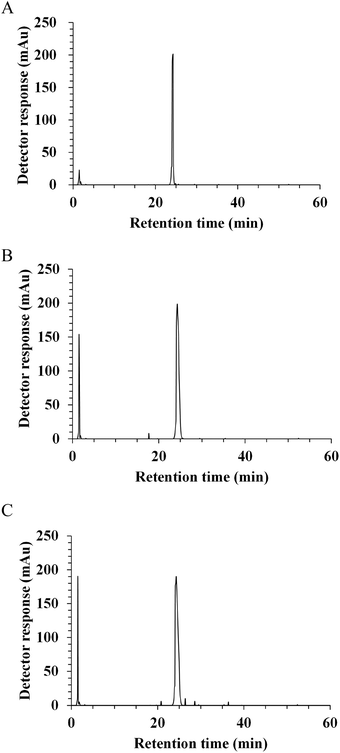 |
| Fig. 5 Stability of synthetic peptide NAPHMR against gastrointestinal digestion observed by RP-HPLC chromatographies. Reverse-phase spectra of peptide before simulated digestion (A), peptide hydrolysed by pepsin for 1 h (B) and peptide subsequently hydrolysed by pancreatin for 4 h (C). | |
After gastrointestinal digestion, orally administered peptides, as exogenous regulators to exert physiological effects, must uptake across the intestinal epithelium in the small intestine before reaching the target site.2 Usually, the Caco-2 cell monolayer is used as a good intestinal cell model to evaluate peptide stability and bioavailability based on their morphological and functional similarities to intestinal enterocytes.30 No visible cell viability decrease was noticed when NAPHMR was incubated with Caco-2 cells for 24 h at a concentration ranging from 1 to 5 mM (data not shown), which is similar to data obtained in case of QIGLF19 and KVLPVP,31 demonstrating that NAPHMR had no significant cytotoxic effect on Caco-2 cells.
Food-derived bioactive peptides are always considered as potential substitutes for drugs owing to the merits of low toxicity and negative side effects.2 Our results strongly suggested that NAPHMR can be used as a potential ACE inhibitory peptide across the intestinal epithelium.
3.5 Insight into molecular docking simulation
To further investigate the intermolecular interactions of NAPHMR to ACE, a flexible docking model was established and the potential binding sites between them were proposed. The best position with potential interaction energy value of −115.944 kcal mol−1 was obtained and its 3D and 2D structures are shown in Fig. 6A and B, respectively. Hydrogen, electrostatic and π-bonds were the three main types of bonds involved in their interaction. Specifically, hydrogen bonds were formed at Gln281, Lys511, Tyr520, Arg522, Glu403, and Asp415 of ACE, while electrostatic bonds were formed at Glu403 and Lys511. In addition, π-bonds were formed at Zn701, Phe457, Tyr523, His383, His410, and His387.
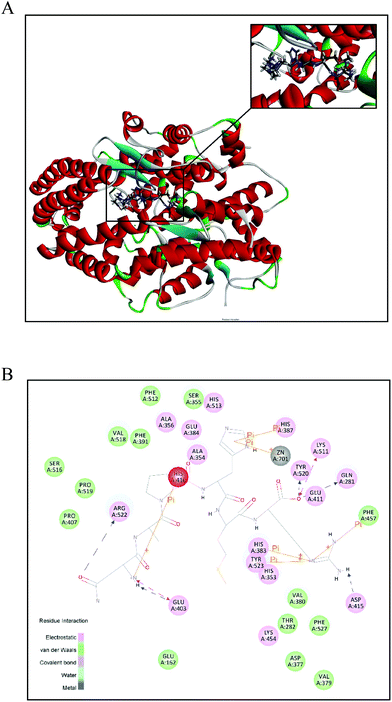 |
| Fig. 6 Best ranked docking position of NAPHMR binding with ACE (PDB: 1O8A). (A) General overview of molecular docking of NAPHMR at the ACE active site. The upper right corner image shows the enlarged view of the binding area of NAPHMR with ACE. NAPHMR is presented in stick model. (B) 2D diagram showing interactions between NAPHMR and ACE amino acid residue obtained from molecular docking simulation. Electrostatic and π-bonds were represented by dotted lines and solid lines, respectively. | |
It was proposed that hydrogen bonds and hydrophobic interactions are two major interactions contributing to stability and inhibitory effect based on X-ray crystallographic structures of complexes of ACE and inhibitory drugs.32 The present molecular docking study revealed that NAPHMR was buried deep inside the ACE structure and was stabilised primarily by seven hydrogen bonds. The presence of multiple hydrogen bonds between NAPHMR and ACE played the most important role for stabilizing the docking complex and inhibiting enzyme catalytic reactions. Among them, the hydrogen bonding of the C-terminal Arg residue in NAPHMR with the S2 pocket of ACE (Gln281:HE22, Lys511:HZ1, Tyr520:HH) was supposed to play a particularly important role, which is in accordance with the report that Lys residue in the C-terminal of YSK established hydrogen bonds with the S2 pocket (Gln281, Lys511 and Tyr520).33 It was reported that Arg or Lys residue in the peptide often experience favorable electrostatic interactions via hydrogen bonds due to the positively charged amino groups of Arg (guanidine group) and Lys (ε-amino group), and Arg is regarded as a zwitterionic amino acid capable of forming hydrogen bonds either as a donor or a receptor.22 In addition, other hydrogen bonds contributed to the stability between the ligand and receptor, such as hydrogen bonding (Arg522:HH12, Arg522:HH22, Glu403:OE1 and Asp415:OD2) established by the N-terminal Asn in NAPHMR with ACE. The amide group of Asn exhibited a higher propensity to form hydrogen bond as the donor or acceptor.27
Moreover, Zn2+ binding motifs in ACE, including Zn701, His383, His387, Glu384 and Glu411, were also reported to influence ACE activity when interacted with other ligands.32,34 Molecular docking showed that NAPHMR binds with Zn701, His383 and His387 of ACE via π-bonds, which might obstruct the binding of ACE with Zn2+ and thus suppress ACE activity. The basic nitrogen atom in the imidazole group of His residue in the peptide was considered as a potential reason for the formation of π-bonds with ACE.35 Thus, in the interaction of ACE with NAPHMR, the Gln281, Lys511, Tyr520, His383 and His387 residues from the ACE active sites and atoms from Arg, Asn and His greatly contributed to the combined stabilisation.
4. Conclusion
In conclusion, potent ACE inhibitory activity peptides were successfully produced from sea cucumber gonad proteins using protamex with hydrolysis time of 1.95 h and E/S ratio of 0.75%. Among them, NAPHMR exhibited the highest potential and its inhibitory effect on ACE was quite possibly due to binding with S2 pocket and interaction with Zn2+ binding motifs. Based on simulated gastrointestinal digestion as well as cytotoxicity to Caco-2 cells, NAPHMR may be used as a potential anti-hypertension functional food.
Conflicts of interest
There are no conflicts to declare.
Acknowledgements
This work received financial support from National Natural Scientific Foundations of China (31271838, 31701676), Science and Technology Planning Project of Fujian Province, China (2017N5011).
References
- J. Wilson, M. Hayes and B. Carney, Angiotensin-I-converting enzyme and prolyl endopeptidase inhibitory peptides from natural sources with a focus on marine processing by-products, Food Chem., 2011, 129, 235–244 CrossRef CAS.
- B. A. Murray and R. J. FitzGerald, Angiotensin converting enzyme inhibitory peptides derived from food proteins: biochemistry, bioactivity and production, Curr. Pharm. Des., 2007, 13, 773–791 CrossRef CAS PubMed.
- J. A. Pérez-Vega, L. Olivera-Castillo, J. Á. Gómez-Ruiz and B. Hernández-Ledesma, Release of multifunctional peptides by gastrointestinal digestion of sea cucumber (Isostichopus badionotus), J. Funct. Foods, 2013, 5, 869–877 CrossRef.
- S. Bordbar, F. Anwar and N. Saari, High-value components and bioactives from sea cucumbers for functional foods-a review, Mar. Drugs, 2011, 9, 1761–1805 CrossRef CAS PubMed.
-
Anonymous, China fisheries yearbook, China Agricultural Press, Beijing, 2017 Search PubMed.
- L. J. Yan, C. L. Zhan, Q. F. Cai, L. Weng, C. H. Du, G. M. Liu, W. J. Su and M. J. Cao, Purification, characterization, cDNA cloning and in vitro expression of a serine proteinase from the intestinal tract of sea cucumber (Stichopus japonicus) with collagen degradation activity, J. Agric. Food Chem., 2014, 62, 4769–4777 CrossRef CAS PubMed.
- J. R. Bi, Y. Li, S. S. Cheng, X. P. Dong, T. Kamal, D. Y. Zhou, D. M. Li, P. F. Jiang, B. W. Zhu and M. Q. Tan, Changes in body wall of sea cucumber (Stichopus japonicus) during a two-step heating process assessed by rheology, LF-NMR, and texture profile analysis, Food Biophys., 2016, 11, 257–265 CrossRef.
- J. Mamelona, R. Saint-Louis and É. Pelletier, Proximate composition and nutritional profile of by-products from green urchin and Atlantic sea cucumber processing plants, Int. J. Food Sci. Technol., 2010, 45, 2119–2126 CrossRef CAS.
- L. X. He, Z. F. Zhang, B. Sun, Q. H. Chen, R. Liu, J. W. Ren, J. B. Wang and Y. Li, Sea cucumber (Codonopsis pilosula) oligopeptides: immunomodulatory effects based on stimulating Th cells, cytokine secretion and antibody production, Food Funct., 2016, 7, 1208–1216 CAS.
- J. Mamelona, É. Pelletier, K. Girard-Lalancette, J. Legault, S. Karboune and S. Kermasha, Quantification of phenolic contents and antioxidant capacity of Atlantic sea cucumber, Cucumaria frondosa, Food Chem., 2007, 104, 1040–1047 CrossRef CAS.
- M. Y. Yan, H. T. Tao and S. Qin, Effect of enzyme type on the antioxidant activities and functional properties of enzymatic hydrolysates from sea cucumber (Cucumaria frondosa) viscera, J. Aquat. Food Prod. Technol., 2016, 25, 940–952 CrossRef CAS.
- J. Zhang, S. W. Liu, Y. Zhang, Y. H. Lu, M. J. Wang, G. M. Wang and X. Liu, Purification and antioxidant ability of peptide from egg in sea cucumber Apostichopus japonicus, Int. J. Food Prop., 2017, 20, 306–317 CrossRef CAS.
- N. Sun, P. B. Cui, Z. Q. Jin, H. T. Wu, Y. X. Wang and S. Y. Lin, Contributions of molecular size, charge distribution, and specific amino acids to the iron-binding capacity of sea cucumber (Stichopus japonicus) ovum hydrolysates, Food Chem., 2017, 230, 627–636 CrossRef CAS PubMed.
- A. Santana, A. Melo, T. Tavares and I. M. Ferreira, Biological activities of peptide concentrates obtained from hydrolysed eggshell membrane byproduct by optimisation with response surface methodology, Food Funct., 2016, 7, 4597–4604 CAS.
- J. W. Chen, Y. M. Wang, Q. X. Zhong, Y. N. Wu and W. S. Xia, Purification and characterization of a novel angiotensin-I converting enzyme (ACE) inhibitory peptide derived from enzymatic hydrolysate of grass carp protein, Peptides, 2012, 33, 52–58 CrossRef CAS PubMed.
- P. M. Nielsen, D. Petersen and C. Dambmann, Improved method for determining food protein degree of hydrolysis, J. Food Sci., 2001, 66, 642–646 CrossRef CAS.
- Y. Y. Huang, G. M. Liu, Q. F. Cai, W. Y. Weng, S. J. Maleki, W. J. Su and M. J. Cao, Stability of major allergen tropomyosin and other food proteins of mud crab (Scylla serrata) by in vitro gastrointestinal digestion, Food Chem. Toxicol., 2010, 48, 1196–1201 CrossRef CAS PubMed.
-
United States Pharmacopoeia, The United States Pharmacopoeia 23, the National Fomulary 18, The United States Pharmacopoeia Convention Inc., Rockville, MD, Simulated gastric fluid and simulated intestinal flud, 2053 Search PubMed.
- L. Ding, Y. Zhang, Y. Q. Jiang, L. Y. Wang, B. Q. Liu and J. B. Liu, Transport of egg white ACE-inhibitory peptide, Gln-Ile-Gly-Leu-Phe, in human intestinal Caco-2 cell monolayers with cytoprotective effect, J. Agric. Food Chem., 2014, 62, 3177–3182 CrossRef CAS PubMed.
- A. Bougatef, N. Nedjar-Arroume, R. Ravallec-Plé, Y. Leroy, D. Guillochon, A. Barkia and M. Nasria, Angiotensin I-converting enzyme (ACE) inhibitory activities of sardinelle (Sardinella aurita) by-products protein hydrolysates obtained by treatment with microbial and visceral fish serine proteases, Food Chem., 2008, 11, 350–356 CrossRef PubMed.
- R. Balti, A. Bougatef, N. E. Ali, D. Zekri, A. Barkia and M. Nasri, Influence of degree of hydrolysis on functional properties and angiotensin I-coverting enyzme inhibitory activity of protein hydrolysates from cuttlefish (Sepia officinalis) by-products, J. Sci. Food Agric., 2010, 90, 2006–2014 CAS.
- A. M. Shi, H. Z. Liu, L. Liu, H. Hu, Q. Wang and B. Adhikari, Isolation, purification and molecular mechanism of a peanut protein-derived ACE-inhibitory peptide, PLoS One, 2014, 9, e111188 Search PubMed.
- S. Ono, M. Hosokawa, K. Miyashita and K. Takahashi, Inhibition properties of dipeptides from salmon muscle hydrolysate on angiotensin I-converting enzyme, Int. J. Food Sci. Technol., 2006, 41, 383–386 CrossRef CAS.
- A. Quirós, M. d. M. Contreras, M. Ramos, L. Amigo and I. Recio, Stability to gastrointestinal enzymes and structure-activity relationship of β-casein-peptides with antihypertensive properties, Peptides, 2009, 30, 1848–1853 CrossRef PubMed.
- J. Tauzin, L. Miclo and J. L. Gaillard, Angiotensin-I-converting enzyme inhibitory peptides from tryptic hydrolysate of bovine αS2-casein, FEBS Lett., 2002, 531, 369–374 CrossRef CAS PubMed.
- M. C. Robert, A. Razaname, M. Mutter and M. A. Juillerat, Identification of angiotensin-I-converting enzyme inhibitory peptides derived from sodium caseinate hydrolysates produced by lactobacillus helveticus NCC 2765, J. Agric. Food Chem., 2004, 52, 6923–6931 CrossRef CAS PubMed.
- T. Dudev and L. Doudeva, How the extra methylene group affects the ligation properties of Glu vs. Asp and Gln vs. Asn amino acids: a DFT/PCM study, J. Mol. Model., 2017, 23, 45 CrossRef PubMed.
- T. Unuma, T. Yamamoto, T. Akiyama, M. Shiraishi and H. Ohta, Quantitative changes in yolk protein and other components in the ovary and testis of the sea urchin Pseudocentrotus depressus, J. Exp. Biol., 2003, 206, 365–372 CrossRef CAS PubMed.
- A. Fujiwara, T. Unuma, K. Ohno and K. Yamano, Molecular characterization of the major yolk protein of the Japanese common sea cucumber (Apostichopus japonicus) and its expression profile during ovarian development, Comp. Biochem. Physiol., Part A: Mol. Integr. Physiol., 2010, 155, 34–40 CrossRef PubMed.
- P. Shah, V. Jogani, T. Bagchi and A. Misra, Role of caco-2 cell monolayers in prediction of intestinal drug absorption, Biotechnol. Prog., 2006, 22, 186–198 CrossRef CAS PubMed.
- H. Y. Sun, D. Liu, S. M. Li and Z. Y. Qin, Transepithelial transport characteristics of the antihypertensive peptide, Lys-Val-Leu-Pro-Val-Pro, in human intestinal caco-2 cell monolayers, Biosci., Biotechnol., Biochem., 2009, 73, 293–298 CrossRef CAS PubMed.
- A. S. Pina and A. C. A. Roque, Studies on the molecular recognition between bioactive peptides and angiotensin-converting enzyme, J. Mol. Recognit., 2009, 22, 162–168 CrossRef CAS PubMed.
- X. M. Wang, H. X. Chen, X. G. Fu, S. Q. Li and J. Wei, A novel antioxidant
and ACE inhibitory peptide from rice bran protein: biochemical characterization and molecular docking study, LWT–Food Sci. Technol., 2017, 75, 93–99 CrossRef CAS.
- P. Li, J. Jia, M. Fang, L. J. Zhang, M. R. Guo, J. L. Xie, Y. L. Xia, L. Zhou and D. Z. Wei,
In vitro and in vivo ACE inhibitory of pistachio hydrolysates and in silico mechanism of identified peptide binding with ACE, Process Biochem., 2014, 49, 898–904 CrossRef CAS.
- S. M. Liao, Q. S. Du, J. Z. Meng, Z. W. Pang and R. B. Huang, The multiple roles of histidine in protein interactions, Chem. Cent. J., 2013, 7, 44 CrossRef PubMed.
Footnote |
† Electronic supplementary information (ESI) available. See DOI: 10.1039/c7fo01388d |
|
This journal is © The Royal Society of Chemistry 2018 |