Spatial variation in the atmospheric deposition of perfluoroalkyl acids: source elucidation through analysis of isomer patterns†
Received
9th March 2018
, Accepted 4th May 2018
First published on 5th June 2018
Abstract
To evaluate the relevance of different proposed sources of perfluoroalkyl acids (PFAAs) to air, their isomer patterns were analyzed in deposition samples collected from five geographical locations: two urban sites in China (>360 km from known operational fluorochemical manufacturing facilities), one remote marine site in the Azores archipelago and two Swedish sites representing urban and background conditions. Despite variable contributions from linear perfluorooctanoic acid (PFOA) in the samples, the pattern of branched PFOA isomers was similar to those of technical standards manufactured using electrochemical fluorination (ECF). This indicates that atmospheric fate processes have little influence on the isomer profiles of PFOA and that the relative contribution of PFOA manufactured using ECF (typically 20–26% branched isomers) and telomerization (typically one single linear isomer) can be determined in atmospheric deposition samples by analyzing the proportions of branched and linear isomers. In Chinese samples, branched isomers contributed 15–25% to the total loading of PFOA, indicating that the samples were dominated by ECF PFOA. Samples in the Azores had 8–10% contribution from branched PFOA isomers, indicating an approximately equal influence of ECF and telomer sources. Only three of the samples collected in Sweden displayed a quantifiable contribution from branched PFOA isomers (8–13% of overall PFOA loading in the samples). One branched PFNA isomer was observed in samples from the marine sites. Direct manufacturing discharges, transport of sea spray aerosols and degradation of precursors are all suggested to be contributing sources, albeit to different extents, to PFAAs in air at the different geographical locations where precipitation was sampled.
Environmental significance
A number of theories have been developed to explain the presence of perfluoroalkyl acids in the atmosphere. However, field data useful to discern the relative importance of the suggested source types are scarce. This study demonstrates that two different manufacturing techniques contribute to perfluorooctanoic acid present in atmospheric samples collected in China, Sweden and on the Azores. This suggests that several types of sources currently influence the levels of PFAAs in the global atmosphere.
|
1. Introduction
Perfluoroalkyl carboxylic acids (PFCAs) and structurally similar perfluoroalkane sulfonic acids (PFSAs), collectively known as perfluoroalkyl acids (PFAAs), are performance chemicals used in the production of fluoropolymers and in industrial metal-plating processes, as well as in a large number of other industrial applications.1,2 Following the onset of their production in the 1950s, these anthropogenic chemicals have been released into the environment in large amounts via numerous sources.1,2 Reports of their persistence,3 bioaccumulation potential,4,5 and toxicity,6,7 as well as their global occurrence in humans8–10 and biota,11,12 have raised concerns regarding long-chain PFCAs and PFSAs among scientists13,14 and regulators.15–18 To explain the occurrence of PFAAs in remote environments the scientific community has suggested two main long-range transport pathways, whose relative importance has been debated since the mid-2000s.19–21 The direct hypothesis asserts that PFAAs themselves are emitted to air and environmental waters during manufacturing and use, after which they are transported in the global environment.22–24 As PFAAs are present in their involatile and water soluble anionic form at typical environmental pH (>3.5),25–28 their long-range transport due to direct emissions into environmental waters29,30 is expected to proceed predominantly via ocean currents22–24 Although industrial emissions to air have been shown to impact local environments,31 there is little empirical evidence that direct sources to air impact the atmospheric levels of PFAAs on the regional scale. However, a modelling study by Armitage et al. has demonstrated that PFOA directly released into air may be subject to long-range transport.23 Atmospheric transport of directly released PFAAs could potentially proceed via transfer from oceans to air in association with sea spray aerosols.32–34 While enrichment of PFAAs in spray aerosol has been demonstrated at the laboratory scale,34 no field studies have been carried out to investigate whether this process impacts PFAA levels in air on a regional scale. The indirect hypothesis asserts that PFAAs in the global environment are the result of the release and long-range transport of precursor compounds, such as fluorotelomer alcohols (FTOHs) and perfluoroalkane sulfonamides and sulfonamido ethanols (FASA/FASEs), which are degraded to form PFAAs in the environment.21,35–37 In the vast majority of studies published on PFCAs in air and in remote environments, transformation of FTOHs is discussed as the dominant source of PFAAs to the atmosphere.21,38–40 This study sets out to test whether influence from other sources, such as sea spray and direct industrial emissions, can be observed in atmospheric samples. Currently, knowledge on the homologue patterns emitted from different sources and whether these patterns can be altered by fate processes is lacking. Therefore, we use PFCA isomer patterns as a marker for different types of sources and transport pathways influencing the global atmosphere. Our justification for this approach is outlined below.
Large-scale manufacturing of PFAAs has been undertaken using two manufacturing techniques: electrochemical fluorination (ECF), which produces a specific mixture of branched and linear isomers, and telomerization, which results in an isomerically pure product that retains the geometry of the starting material (usually linear, but also isopropyl branched).41 Between 1947 and 2002, large-scale manufacturing of PFOA and PFSAs was undertaken by electrochemical fluorination (ECF). After the major producer (3M Company) phased out its manufacturing of C6, C8 and C10 substances, other American, European and Japanese fluorochemical manufacturers (hereafter referred to as Western fluorochemical manufacturers) increased their production of PFOA and PFNA using telomerization, which was initiated in the 1970s.2 Later, as part of the PFOA Stewardship Program,42 the majority of the Western fluorochemical manufacturers phased out their production of telomer-derived PFOA in 2015. Chinese manufacturers started to manufacture PFOA and PFOS by ECF shortly after the 3M phase out1,2 and manufacturing using ECF is still ongoing in China.29,30 In other words, PFOS and other PFSAs have only been manufactured using ECF, yielding a technical product with a content of 30% branched isomers. PFOA, on the other hand, has been manufactured both using ECF (approximately 22% branched isomers) and telomerization techniques during different periods. Manufacturing of PFHxA using telomerization is currently ongoing.2 Furthermore, PFCAs are present as impurities in both ECF PFOA and ECF PFOS.2 Such impurities are mixtures of structural isomers which exhibit a substantial contribution from branched isomers (up to 65%).43
Transformation of precursors manufactured using ECF is expected to generate PFCAs of different chain lengths36 with branched isomer patterns of unknown composition (although this has not been tested experimentally to our knowledge). On the other hand, transformation of volatile precursors manufactured using telomer technology, for example fluorotelomer alcohols (FTOHs), is expected to contaminate the environment with linear PFAAs. Direct industrial emissions could generate both ECF and telomer PFOA, reflecting the techniques used in specific plants. Manufacturing and industrial use of PFOA is currently ongoing in China, where ECF is the most common manufacturing technique.44,45 PFOA isomer patterns corresponding to the ECF signal have been observed in rivers downstream of Chinese manufacturing facilities29,30 and in snow collected in Northern China.46 Furthermore, as the ECF isomer pattern has been observed to different degrees throughout the Atlantic Ocean and the Canadian Arctic,47 atmospheric samples influenced by sea spray aerosols are also expected to display an ECF pattern, presuming that this pathway makes an important contribution to the loading of PFOA in the sample and that there is no isomer fractionation during the sea-to-air transport. The aim of this study is to discern the predominant sources of PFAAs to the atmosphere by studying their isomer patterns in precipitation samples collected at sites suspected to be influenced by the proposed sources. For this purpose, five sampling locations were chosen to represent remote, urban, coastal, and inland environments, as well as a region of known manufacturing sources of PFAAs or their precursors (Fig. 1). Our specific objectives are to (1) determine if PFOA isomer patterns in bulk deposition (representing both wet and dry deposition) samples are consistent spatially, (2) determine the relative importance of ECF versus telomerization sources in bulk deposition samples at the locations sampled. All PFCA isomer patterns are relevant for source elucidation and are monitored in this study. However, the focus of the study is PFOA due to the unavailability of standards to characterize the isomer pattern of other PFCAs. Two previous studies have analysed isomer patterns of PFOA in atmospheric samples: De Silva et al. observed branched PFOA isomers in Canadian precipitation (n = 6)48 and Shan et al. observed an ECF pattern of PFOA isomers in snow collected in nineteen cities in Northern China during one single day.46
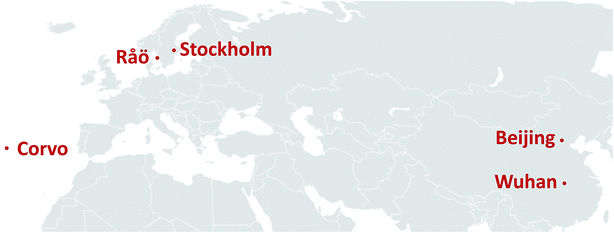 |
| Fig. 1 Map showing the sample sites. | |
2. Materials and methods
2.1 Selection of sampling sites
For several PFAAs and some of their fluorinated alternatives, industrial point sources in China currently dominate the global emissions.29 For this study, two sampling sites in China were selected: Wuhan and Beijing. Wuhan is a large city with 10 million inhabitants located in the Hubei province, where several PFOS manufacturing plants are located.49 To our knowledge, there is no manufacture or large-scale industrial use of PFASs in Beijing (21.5 million inhabitants). However, PFASs may be used in metal-plating industries in the adjacent Heibei province.49 Large scale fluoropolymer manufacturing takes place in Shangdong (about 360 km from Beijing) and in Shanghai (about 690 km from Wuhan). We are not aware of any fluoropolymer plant located closer to the sampling sites. While Wuhan is a site which may be influenced by industrial point sources, Beijing was chosen to represent an urban background environment in a source region. Two sites receiving input from sea spray were included in the study: Råö and Corvo. Corvo is the smallest and the northernmost island of the Azores archipelago, located in the North Atlantic Ocean, 3700 km east of Portugal. The island has approximately 450 inhabitants. Råö is a rural island on the Swedish west coast. Råö is used as a background station in the Swedish environmental monitoring program, but is not considered a remote site as it receives air masses from the European continent. Samples were also taken in Stockholm, a site selected to represent an urban European site. Metropolitan Stockholm has 2
270
000 inhabitants and is located on the Baltic Sea coast. Large-scale manufacturing of PFAAs has not occurred in Scandinavia. Therefore, none of the European sites are expected to be influenced by direct industrial emissions.
2.2 Sampling
Monthly bulk deposition samples were collected on Råö by IVL Swedish Environmental Research Institute, as part of an environmental monitoring program coordinated by the Swedish Environmental Protection Agency (Naturvårdsverket). We received subsamples of these bulk deposition samples, representing seven of the months sampled in 2015. Twelve rain samples and two snow samples were collected on the roof of Stockholm University between May 2015 and May 2016. Five precipitation samples were collected from mid-July to early September 2015 on the roof of the Research Center for Eco-Environmental Science, Chinese Academy of Sciences, Beijing. Four samples were also taken on the roof of Jianghan University in Wuhan from the end of August to the end of November 2015. At the Chinese sites sampling was performed in duplicate, i.e. 18 (5 × 2 in Beijing and 4 × 2 in Wuhan) samples were obtained in total. Five precipitation samples were collected at the meteorological station on the island of Corvo in the Azores, Portugal. The samples were taken from early January to late March 2016.
All precipitation samples were bulk deposition samples collected using an open funnel. The Råö samples were collected using a 26 cm polyethylene funnel and stored refrigerated in 5 L polyethylene bottles, while samples from the other sites were collected using 20 cm polypropylene funnels screwed onto 2 L polypropylene bottles. The 2 L bottles were wrapped in aluminum foil to prevent exposure to sunlight. Apart from bulk deposition samples collected on Råö, samples were not taken during a fixed time period. Instead, precipitation was collected until a volume of precipitation of at least 1 L was obtained. As the frequency, intensity and duration of precipitation events varied greatly over time and at the different geographical locations, collection of the required sample volume (1 L) was achieved at different speeds. Therefore, each sample represents a different time period, ranging from 24 hours to 51 days (Table S1†). Samples were transported to the lab by shipping services within a few weeks of sample collection and all samples were stored at 4 °C until analysis. Snow was sampled using a polypropylene box with dimensions 69 × 33 × 13 cm, as the funnels used to sample precipitation were not efficient at sampling snow. All of the Corvo samples were taken over the course of 7 to 14 days, except one which was taken over the course of 24 hours on an especially rainy day.
2.3 Sample extraction and instrumental analysis
All samples were approximately 1 L, except those from China which were 50 mL due to restrictions on transporting liquids that are enforced by the Chinese customs. Subsampling was performed after 30 minutes of ultrasonication (except for Råö samples, which were subsampled after vigorous shaking). In all samples, the pH was determined to be approximately 4–6, using indicator strips (Merck). In separate 10 mL subsamples, concentrations of major ions were determined by chemically suppressed ion chromatography, as described in the ESI.†
The samples were spiked with 400 pg isotope-labeled internal standard, agitated and left to equilibrate overnight. Subsequently, they were vacuum filtered using glass microfiber filters (VWR, particle retention 1.2 μm). Filtration of the precipitation samples before solid phase extraction (SPE) was necessary to ensure that the SPE cartridges were not clogged and that the complete samples could be extracted. This was especially important for samples taken during spring and summer months. The samples were then cleaned on Oasis weak-anion exchange (WAX) SPE cartridges (6 cm3, 150 mg, 30 μm) using a previously published method.50 The SPE eluent was reduced to 80 μL under a gentle flow of nitrogen at 40 °C. It was then combined with 100 μL of Milli-Q water and spiked with 20 μL volumetric standard (400 pg in methanol).
The final extracts were analyzed using ultra high performance liquid chromatography tandem mass spectrometry coupled with electrospray ionization on a Thermo Scientific Dionex UltiMate 3000 UHPLC coupled to a ThermoScientific TSQ Quantiva mass spectrometer, according to a previously published method.51 The target analytes were four PFSAs (CnF2n+1SO3−, n = 4, 6, 8, 10) and eleven PFCAs (CnF2n+1COO−, n = 4–14). Linear analytes were quantified using the internal standard method, as described in the ESI.† Concentrations between the LOD and the LOQ (reported in Table S9†) were quantified and used in the statistical analysis. The distribution between the linear and the sum of branched isomers was determined by comparing their respective peak areas in the precursor/product ion transitions 413/369 and 499/80 for PFOA and PFOS, respectively. These values were normalized to the distribution of branched and linear isomers observed for characterized isomeric mixtures of PFOA and PFOS (TPFOA and TPFOS from Wellington Laboratories) in the same transitions. The total concentration of PFOA and PFOS in the samples, i.e. the sum of branched and linear isomers, was calculated from the quantified concentration of linear homologues and the determined distribution between branched and linear isomers. To investigate the fractionation of structural isomers, the intensity of isomer-specific product ions (Table S2†) was monitored and compared to TPFOA and TPFOS, according to a strategy previously described in ref. 51. Sample-specific LODs and LOQs for the sum of branched isomers (∑brPFOA and ∑brPFOS) are expressed as percentage contributions to the sum of branched and linear isomers (i.e. total PFOA and PFOS) and represent the content of branched isomers that can be distinguished from the noise at S/N > 3 and 10 respectively. For substances other than PFOS and PFOA, chromatographic peaks of branched PFAA isomers were distinguished from interferents by fulfilment of two criteria: (i) observation of peaks for multiple product ions (ii) elution within 4 min of the linear isomer peak. Further details on sample extraction and instrumental analysis are given in the ESI.†
2.4 Quality assurance
Each extracted batch included 4–10 samples and a minimum of three method blanks. The blanks were prepared by rinsing an unused sample bottle with 10 mL MilliQ water. This water was subsequently extracted in the same manner as other samples. Blank contamination was detected for n-PFOA in all sample batches and for perfluorohexanoic acid (PFHxA) and perfluorodecanoic acid (PFDA) in two sample batches. The concentration of n-PFOA in sample extracts exceeds that in the blanks by at least one order of magnitude. Therefore, blank contamination of PFOA is not expected to influence the determination of the percentage contribution of the sum of branched PFOA isomers (∑brPFOA). Method detection limits (MDLs) were defined based on the observed blank contamination, as described in the ESI.† The reported concentrations in this study are not blank corrected.
The recovery of internal standard was 30–130% in a majority of the samples (Table S3†). Recoveries outside this range are indicated along with their corresponding analyte concentrations in Table S9.† The use of internal standard accounts for matrix effects and for sorptive losses, which may arise during storage and filtration.52,53 However, concentrations of PFDoDA, PFTriDA, PFTeDA and PFDS are not reported in this study as their corresponding internal standard (13C2-PFDoDA) exhibited poor recovery (<20%) in a majority of the samples. Furthermore, in two thirds of the Swedish samples, reported concentrations of PFUnDA are associated with a higher uncertainty as the recovery of their corresponding internal standard (13C2-PFUnDA) was poor (<30%). Data for perfluorobutanoic acid (PFBA), perfluoropentanoic acid (PFPeA) and perfluorobutane sulfonic acid (PFBS) were omitted due to matrix problems resulting in high baselines and co-elution with matrix.
The response of branched and linear isomers was tested in the range of 5–14
200 pg on the column for TPFOA and 24–2500 pg on the column for TPFOS. The dynamic range, i.e. the range within which the ratios of branched isomers to the linear isomer is stable (described by Benskin et al.54), was found to be 15–14
200 pg on column for PFOA and 80–2500 pg on column for PFOS. All PFOA concentrations reported in this study were within the dynamic range. PFOS concentrations were below the dynamic range for the determination of branched isomers in all Chinese samples. None of the Stockholm samples exhibited signals of branched PFOS isomers which were both within the dynamic range and had an S/N ratio above 10.
2.5 Statistics
Spearman rank correlation coefficients were calculated using the Excel Data Analysis Toolpak (Microsoft). This analysis was performed to investigate whether homologue concentrations were correlated with the input of sea salt (i.e. sodium ions) or the number of sampling days. This analysis was only performed on data from Corvo and Råö and only analytes that had a detection frequency >50% were included. To test whether the patterns of branched PFOA and PFOS isomers differed between locations, one-way Multivariate Analysis of Variance (MANOVA) was performed on the logarithm of ratios of individual branched isomers. If the MANOVA indicated that not all compared means were equal, a pairwise post hoc test was applied to determine which location was statistically different. These tests were performed as contrasts in the MANOVA model using statistics software STATA (version 13).
2.6 Calculation of deposition rates
Deposition rates were calculated using data on rainfall amount collected from the Swedish Meterological and Hydrological Institute (SMHI), Instituto Português do Mar e da Atmosfera (IPMA) and the National Oceanic and Atmospheric Administration (NOAA, data retrieved from http://www.rp5.ru). The level of contamination in precipitation samples is highly dependent on the frequency of rain events during the sampling period and the duration of the rain events. As PFAAs are efficiently scavenged by rain and washed out with the first deposited millimeters,55 long rain events will dilute the PFAA concentration in the samples. The highest concentrations are expected in samples subjected to a high number of short rain events spaced so that concentrations of PFAAs can build up in air between events. Furthermore, input from dry deposition may influence contamination levels in the samples and will have a higher relative influence on samples representing long sample periods with short and light rain events. Dry deposition has not been studied for PFAAs and its importance cannot easily be estimated due to the lack of reliable field measurements of PFAA gas-particle distribution.56
3. Results
3.1 Linear PFAA homologues
All PFCAs included in the study (C6–C11) had 100% detection frequency, except for PFUnDA (89%). More than half of the detected PFUnDA concentrations fell under the quantification limit, while for PFDA predominantly Wuhan samples displayed concentrations below the quantification limit (Table S9†). PFHxS had a low detection frequency in samples from China and Stockholm (25 and 52% respectively) and only exceeded quantifiable levels in samples taken on Corvo and on Råö in the winter. Because the extracted sample volume was 10 times lower in samples from China than in samples from Sweden and the Azores, the Chinese samples exhibited higher LODs for all target analytes. PFOS was below the quantification limit in all Chinese samples and was only detected in three of them.
In the Chinese samples the deposition fluxes of ∑PFAAs were 12–170 ng per m2 per day, while in the precipitation samples collected outside of China the deposition rates were 1.0–56 ng per m2 per day (Table S10†). On average, PFHpA, PFOA and PFNA made an approximately equal contribution to the homologue pattern in Swedish samples (15, 23 and 23% by weight, respectively). However, their relative contribution was quite variable in individual samples (Fig. 2). On Råö, the contribution of PFHxS and PFOS was elevated in winter (November–February) relative to summer (July–September) by on average a factor of 5 and 3.5, respectively. The precipitation collected on Corvo between January and March 2016 displayed a uniform homologue pattern dominated by PFOA, PFNA and PFOS each contributing to the overall homologue pattern with on average 28, 25 and 20%, respectively. PFOA strongly dominated the homologue pattern in the Chinese samples, contributing on average 53%. However, in one of the Wuhan samples PFHxA made up 55% of the ∑PFAAs.
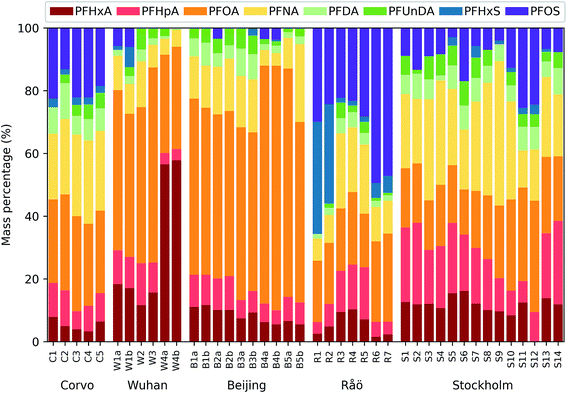 |
| Fig. 2 Homologue pattern of PFAAs displayed as percentage by weight in precipitation sampled on Corvo (C), in Wuhan (W), in Beijing (B), on Råö (R) and in Stockholm (S). Each data point represents an individual sample. PFOA and PFOS concentrations are expressed as the sum of branched and linear isomers. Concentrations below the MDL are not displayed. PFHxA was not quantified in sample S12 due to interference of the matrix. | |
3.2 Ion analysis
Precipitation collected in both Wuhan and Beijing contained high amounts of sulfate (SO42−), nitrate (NO3−) and ammonium (NH4+) (Fig. S1†). The presence of SO42−, NO3− and NH4+ indicates a significant anthropogenic influence whereby the gaseous precursors SO2 and NOx (of anthropogenic origin) react with oxidants such as O3 and OH radicals to form H2SO4-containing aerosols and gaseous HNO3 which subsequently undergo gas-to-particle reactions with ammonia (NH3) to form ammonium salts.57 In addition to this anthropogenic signature, the Wuhan samples contained a relatively high amount of calcium (Ca2+), indicative of terrestrial dust sources.58 All samples from Corvo had an ion mass fraction similar to that of seawater (e.g.ref. 59) with a chloride to sodium mass ratio (Cl−
:
Na+) of ∼1.8 (Fig. S2†) which suggests a strong influence of sea spray aerosol on bulk deposition at this location. Similarly, bulk deposition at Råö was significantly influenced by sea spray aerosol during November, December and January (R1, R6, R7) when these samples exhibited a mass fraction similar to seawater and a Cl−
:
Na+ of ∼1.8. However, departure of the ion mass fraction from that of seawater and lower Cl−
:
Na+ ratios in other months at Råo suggest that this station was less affected by sea spray aerosols outside of November, December and January. The Stockholm samples exhibited variable ion mass fractions and the bulk deposition samples collected here were likely influenced by both sea spray and anthropogenic aerosols. A statistically significant (p < 0.05) positive correlation with sodium, which can be used as a proxy for sea spray aerosol, was observed for PFOA, PFHxS and PFOS in samples taken on Råö (Spearman's rho > 0.96, Fig. S3†).
3.3 Isomer patterns
Branched isomers contributed to the total loading of PFOA with 8–10%, 17–24% and 15–25% in samples from Corvo, Wuhan and Beijing respectively (Fig. 3). In the Swedish samples, the sum of branched isomers had a low detection frequency (38%) and exceeded the LOQ in only three cases. Detectable ∑brPFOA was 8–13%, i.e. in the same range as the detection limits in the samples that did not exhibit detectable levels (3–14%). In all samples that displayed quantifiable ∑brPFOA, the pattern of individual branched isomers was close to that of ECF PFOA (Tables S8 and S11, Fig S5†). One-way MANOVA was applied to ratios of concentrations of 4- and 5-PFOA to 6-PFOA (i.e. 4-PFOA/6-PFOA and 5-PFOA/6-PFOA). The null hypothesis, that the pattern of individual branched PFOA isomers was similar at all locations, was rejected (Wilk's lambda = 0.146, p < 0.05). A subsequent post hoc test indicated that the patterns of structural isomers observed in samples from Beijing, Wuhan and Råö differed from each other (Table S13†).
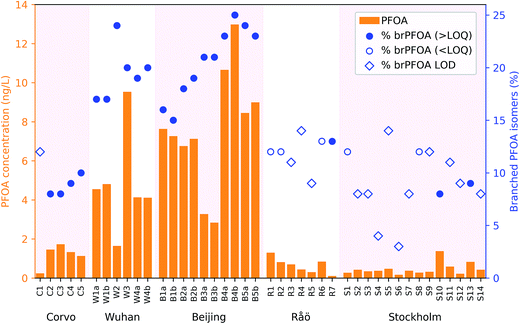 |
| Fig. 3 Concentration of PFOA (ng L−1) and contribution from ∑branched PFOA isomers (%) in precipitation sampled on Corvo (C), in Wuhan (W), in Beijing (B), on Råö (R) and in Stockholm (S). Each data point represents an individual sample. Contribution from ∑branched PFOA isomers (%) is displayed as filled blue dots for samples which had a signal of branched isomers with a S/N ratio > 10 and blue circles for samples which had a detectable contribution from branched PFOA isomers, although at a S/N ratio < 10. Sample-specific detection limits for the contribution of branched isomers (%) are displayed as blue diamonds. | |
The percentage contribution of the sum of branched PFOS isomers (∑brPFOS) was 37–47% and 35–42% in precipitation collected on Corvo and Råö, respectively. In samples from Stockholm, ∑brPFOS ranged from 31% to 42%, but exceeded the LOQ only in 42% of the samples. Furthermore, in a majority of the Stockholm samples the observed PFOS concentrations were not within the dynamic range for isomer analysis, making quantification of the contribution of branched PFOS isomers uncertain. As Chinese samples displayed concentrations of n-PFOS < LOQ, the corresponding ∑brPFOS fell below the LOD. MANOVA was performed on the mean ratios of 3- + 4-PFOS and 5-PFOS to 6-PFOS. Only data from Corvo and Råö were included in this analysis, as the branched PFOS isomers were below the LOQ or not within the dynamic range in the data from the other sites. The pattern of the branched PFOS isomers (displayed in Table S12†) did not differ between Råö and Corvo (Wilk's lambda = 0.947, p = 0.804). Two partly coeluting branched isomers were observed for PFHxS in samples C2–5 and all samples from Råö, except R4–5. These peaks had an S/N ratio close to 3. One branched isomer was observed for PFNA in samples C1–5 and sample R7. Examples of chromatograms for branched PFHxS and PFNA isomers are displayed in Fig. S5.†
4. Discussion
Previous studies have made different observations regarding PFAA homologue patterns in atmospheric deposition samples. Dreyer et al. reported uniform homologue patterns of PFAAs in deposition samples over the course of a year in Northern Germany.60 On the other hand, Kwok et al. reported that PFAA homologue patterns varied spatially and temporally in deposition samples collected in several countries.61 The observation that PFAA homologue patterns can vary in space and time suggests that (i) the global atmosphere is impacted by several different source types or (ii) PFAA homologues undergo different fate processes in the atmosphere and are as a consequence fractionated in relation to each other. Current knowledge on what homologue patterns are emitted from different types of sources and whether these patterns are altered by fate processes is very limited. Therefore, homologue patterns observed in atmospheric samples are not useful tools for source elucidation at this point in time. Since all PFCAs have been manufactured using both ECF and telomerization techniques, study of their isomer patterns in environmental matrices is relevant to source elucidation. However, standards to characterize the isomer pattern of PFCAs other than PFOA are currently not available. This prevents optimization of mass spectrometer settings for their analysis and determination of their detection limits. Consequently, a low signal of branched PFCA isomers could be due to both low abundance of the isomers in the samples and to the fact that the instrumental method may be less sensitive to these than their corresponding linear isomers. Isomer characterization of PFSAs is less useful for source elucidation as these substances have only been manufactured using ECF and are therefore expected to display similar isomer patterns across different sources. Study of PFSA isomer patterns can however contribute to understanding isomer fractionation in the environment.
The range of ∑brPFOA observed in samples from Wuhan and Beijing (15–25%) overlaps with the content of branched isomers (20–26%) in technical APFO products currently marketed in large quantities by the five major manufacturers in China.30 A similar observation was made by Shan et al.46 who reported that branched PFOA isomers contributed 13–22% to the overall concentration of PFOA in precipitation from 19 cities in Northern China. This suggests that ECF sources make a substantial contribution to the contamination of PFOA in Chinese precipitation. In the European samples, branched PFOA isomers contributed with under 13% to the overall PFOA loading. This suggests that a mixture of ECF and telomer-derived sources contributes to the PFOA deposition at the studied sites. In the majority of the Stockholm samples, only n-PFOA was detected, indicating that deposition of PFOA in Stockholm predominantly stems from telomer sources. However, due to the low overall concentration of PFOA in these samples, it is possible that branched PFOA isomers, although not detectable, could contribute with 3–14% to the total loading of PFOA. Contribution from ECF PFOA may stem from ongoing manufacturing sources, transport of sea spray aerosols or degradation of ECF-derived precursors. Additionally, direct emissions of legacy ECF PFOA, that is still in use or present in waste streams, could influence the global atmosphere. However, the scientific literature has not identified any sources of this type relevant for emissions to air. A study performed on Atlantic and Canadian Arctic seawater reported that the isomer pattern of PFOA varies spatially, with elevated telomer signals more often observed in coastal regions than in the open ocean.47 If the isomer pattern of PFOA was constant throughout the global oceans it might be possible to use this tracer to quantify the input of PFOA from sea spray in an atmospheric sample. As this is not the case, the presence of ECF PFOA in an atmospheric sample can only be used to indicate the input of sea spray-associated PFOA and cannot be used quantitatively. Furthermore, as ECF PFOA could also stem from industrial emissions sea spray is here only discussed as a source for samples (i) collected distant from industrial sources (ii) with substantial levels of sea salt. As the contribution of sea spray aerosol was likely minor in the Chinese precipitation samples (Fig. S1†), the ECF PFOA observed in these samples is not likely to stem from sea spray aerosols. In Beijing and Wuhan, there is no known ongoing manufacturing or industrial use of PFOA production. Therefore, the isomer pattern of PFOA observed in Chinese precipitation in this study suggests that emissions to air from industrial sources in Asia contribute to regional-scale atmospheric transport of PFOA. It is unclear whether PFOA emitted from such sources has a sufficient long-range atmospheric transport potential to influence precipitation in Europe, where no large-scale manufacturing or industrial use of ECF PFOA is currently ongoing. If degradation of ECF-derived precursors was a significant source of PFCAs in the samples investigated, we would expect to see more branching in PFCA homologues other than PFOA. Such branching would also be observed if unintentional ECF manufacturing was a dominant source of PFCAs (other than PFOA) to the atmosphere. However, we only observed one single branched isomer of PFNA in precipitation from Corvo. This single isomer could either be a marker of ECF manufacturing or the use of an isopropyl telogen during the telomerization process. Isopropyl-branched PFCAs, as well as ECF-derived PFCAs, were previously observed by De Silva et al.48 in the Canadian environment. In precipitation, these authors observed branched isomers of PFHxA, PFHpA and PFOA, but not of PFNA. In conclusion, the Swedish and the Azorian samples collected as part of this study were likely influenced both by PFOA transferred from seawater in association with sea spray aerosols and of PFOA formed in the atmosphere via degradation of FTOHs. Furthermore, as the Chinese sampling sites do not have any known local sources, the PFOA measured in Chinese precipitation was likely emitted to air from industrial sources and subject to long-range transport.
An underlying assumption for using PFCA isomer patterns as a source indicator is that linear and branched isomers have similar environmental fates. This assumption seems to be valid for aqueous matrices where advection dominates the fate, such as ocean47 and river water,30 but not for matrices influenced by partitioning to organic matter, such as soil.38,62 It is currently not known whether fractionation occurs during emission to air from manufacturing sources or on sea spray aerosols. Furthermore, no previous studies have investigated PFAA isomer fractionation as a consequence of partitioning in the atmosphere. However, as PFAAs are efficiently scavenged by rain and washed out with the first deposited millimeters,55,63 this process is not expected to discriminate between structural isomers. Furthermore, laboratory experiments28 have shown that PFOA isomers are not expected to fractionate in the environment via volatilization, suggesting that Henry's law constants and acid dissociation constants are similar for branched and linear isomers. Given the apparent similarity in the Henry's law constants of PFOA isomers we further do not expect isomer fractionation resulting from differences in scavenging of gaseous PFOA by cloud droplets. The present study demonstrates patterns of individual branched isomers consistent with that of ECF PFOA in precipitation collected in China, in Sweden and on the Azores. This suggests that PFOA isomer patterns are not subject to substantial fractionation during regional transport in the atmosphere. The statistical analysis performed on our data showed that the average pattern of individual branched PFOA isomers differed between samples taken in Beijing, in Wuhan and on Råö. This could be explained either by differences in environmental partitioning of individual isomers or by the different sampling sites being influenced by sources of ECF PFOA which do not have identical ECF fingerprints. Variations in the pattern of individual branched isomers were previously reported in technical APFO products manufactured in China30 and in commercial samples of PFOS of different origins.64 Although the overall isomer composition of ECF-derived fluorochemicals can vary from manufacturer to manufacturer,43,64 we are not aware of any technical PFOS or perfluorooctanesulfonyl fluoride-based product that has a content of branched isomers exceeding 34%. Therefore, the elevated ∑brPFOS (up to 47%) observed in the European precipitation analyzed within this study is likely the result of fractionation in the environment. Enrichment of branched PFOS isomers has previously been observed in surface waters52,65–67 and may be a consequence of the fact that linear isomers are more prone to partitioning to particulate material, leading to enrichment in sediments (as observed by Kärrman et al.62).
To enable the use of isomer patterns for quantitative determination of the relative importance of ECF and telomer sources, an increased understanding is required both of the isomer composition emitted from different sources and of the processes which may cause fractionation of isomer patterns in the environment. Nevertheless, our results demonstrate that ECF and telomer sources both influence PFOA contamination levels in the global atmosphere. This implies that direct releases from manufacturing sources, transport of sea spray aerosols and degradation of precursors may all contribute to atmospheric PFOA pollution to different extents at various geographical locations. Further research is needed to determine the relative importance of these sources.
Conflicts of interest
There are no conflicts of interest to declare.
Acknowledgements
We thank António Pimentel and Gregory Domingos of Estação Meteorológica do Corvo for carrying out precipitation sampling. We thank Diamantino Henriques of Instituto Português do mar e da atmosfera (IPMA) for coordinating the sampling on Corvo. We thank the IVL Swedish Environmental Research Institute for providing precipitation samples from Råö. We thank Zhen Zhou of Jianghan University for carrying out sampling in Wuhan. We thank Robin Vestergren of Stockholm University for providing contact to collaborators in China. This research was funded by the Swedish Research Council Formas (project number 2011-1345) and the National Natural Science Foundation of China (project number 21677154).
References
- Z. Wang, J. M. Boucher, M. Scheringer, I. T. Cousins and K. Hungerbühler, Toward a comprehensive global emission inventory of C4–C10 perfluoroalkane sulfonic acids (PFSAs) and related precursors: focus on the life cycle of C8-based products and ongoing industrial transition, Environ. Sci. Technol., 2017, 51(8), 4482–4493 CrossRef PubMed.
- Z. Wang, I. T. Cousins, M. Scheringer, R. C. Buck and K. Hungerbühler, Global emission inventories for C4–C14 perfluoroalkyl carboxylic acid (PFCA) homologues from 1951 to 2030, Part I: production and emissions from quantifiable sources, Environ. Int., 2014, 70, 62–75 CrossRef PubMed.
-
T. K. Frömel and T. P. Knepper, in Reviews of Environmental Contamination and Toxicology, ed. D. M. Whitacre and P. de Voogt, Springer, New York, 2010, pp. 161−178 Search PubMed.
- J. M. Conder, R. A. Hoke, W. De Wolf, M. H. Russell and R. C. Buck, Are PFCAs bioaccumulative? A critical review and comparison with regulatory criteria and persistent lipophilic compounds, Environ. Sci. Technol., 2008, 42, 995–1003 CrossRef PubMed.
- L. Vierke, C. Staude, A. Biegel-Engler, W. Drost and C. Schulte, Perfluorooctanoic acid (PFOA)—main concerns and regulatory developments in Europe from an environmental point of view, Environ. Sci. Eur., 2012, 24, 16 CrossRef.
- C. Lau, K. Anitole, C. Hodes, D. Lai, A. Pfahles-Hutchens and J. Seed, Perfluoroalkyl acids: A review of monitoring and toxicological findings, Toxicol. Sci., 2007, 99, 366–394 CrossRef PubMed.
- D. Borg, B.-O. Lund, N.-G. Lindquist and H. Håkansson, Cumulative health risk assessment of 17 perfluoroalkylated and polyfluoroalkylated substances (PFASs) in the Swedish population, Environ. Int., 2013, 59, 112–123 CrossRef PubMed.
- L. S. Haug, C. Thomsen and G. Becher, Time Trends and the Influence of Age and Gender on Serum Concentrations of Perfluorinated Compounds in Archived Human Samples, Environ. Sci. Technol., 2009, 43, 2131–2136 CrossRef PubMed.
- K. Kato, L.-Y. Wong, L. T. Jia, Z. Kuklenyik and A. M. Calafat, Trends in Exposure to Polyfluoroalkyl Chemicals in the U.S. Population: 1999–2008†, Environ. Sci. Technol., 2011, 45, 8037–8045 CrossRef PubMed.
- T. Zhang, Q. Wu, H. W. Sun, X. Z. Zhang, S. H. Yun and K. Kannan, Perfluorinated compounds in whole blood samples from infants, children, and adults in China, Environ. Sci. Technol., 2010, 44, 4341–4347 CrossRef PubMed.
- M. Houde, A. O. De Silva, D. C. G. Muir and R. J. Letcher, Monitoring of Perfluorinated Compounds in Aquatic Biota: An Updated Review PFCs in Aquatic Biota, Environ. Sci. Technol., 2011, 45, 7962–7973 CrossRef PubMed.
- J. P. Giesy and K. Kannan, Global distribution of perfluorooctane sulfonate in wildlife, Environ. Sci. Technol., 2001, 35, 1339–1342 CrossRef PubMed.
- A. Blum, S. A. Balan, M. Scheringer, X. Trier, G. Goldenman, I. T. Cousins, M. Diamond, T. Fletcher, C. Higgins and A. E. Lindeman, The Madrid statement on poly-and perfluoroalkyl substances (PFASs), Environ. Health Perspect., 2015, 123, A107 CrossRef PubMed.
- M. Scheringer, X. Trier, I. T. Cousins, P. de Voogt, T. Fletcher, Z. Wang and T. F. Webster, Helsingør Statement on poly-and perfluorinated alkyl substances (PFASs), Chemosphere, 2014, 114, 337–339 CrossRef PubMed.
- Stockholm Convention on Persistent Organic Pollutants, Annex B, Decision SC-4/17, http://chm.pops.int/, accessed September 9, 2015.
- ECHA, European Chemical Agency, Candidate List of Substances of Very High Concern for Authorisation, http://echa.europa.eu/web/guest/candidate-list-table, 2013.
- ECHA, ANNEX XV PROPOSAL FOR A RESTRICTION – Perfluorooctanoic acid (PFOA), PFOA salts and PFOA-related substances, 2014.
- UNEP, Draft risk profile – Pentadecafluorooctanoic acid (PFOA, Perfluorooctanoic acid), its salts and PFOA-related compounds, 2016.
- F. Wania, A global mass balance analysis of the source of perfluorocarboxylic acids in the Arctic Ocean, Environ. Sci. Technol., 2007, 41, 4529–4535 CrossRef PubMed.
- I. T. Cousins, D. Kong and R. Vestergren, Reconciling measurement and modelling studies of the sources and fate of perfluorinated carboxylates, Environ. Chem., 2011, 8, 339–354 Search PubMed.
- D. A. Ellis, J. W. Martin, A. O. De Silva, S. A. Mabury, M. D. Hurley, M. P. S. Andersen and T. J. Wallington, Degradation of fluorotelomer alcohols: A likely atmospheric source of perfluorinated carboxylic acids, Environ. Sci. Technol., 2004, 38, 3316–3321 CrossRef PubMed.
- J. M. Armitage, M. MacLeod and I. T. Cousins, Comparative Assessment of the Global Fate and Transport Pathways of Long-Chain Perfluorocarboxylic Acids (PFCAs) and Perfluorocarboxylates (PFCs) Emitted from Direct Sources, Environ. Sci. Technol., 2009, 43, 5830–5836 CrossRef PubMed.
- J. M. Armitage, M. MacLeod and I. T. Cousins, Modeling the Global Fate and Transport of Perfluorooctanoic Acid (PFOA) and Perfluorooctanoate (PFO) Emitted from Direct Sources Using a Multispecies Mass Balance Model, Environ. Sci. Technol., 2009, 43, 1134–1140 CrossRef PubMed.
- J. M. Armitage, U. Schenker, M. Scheringer, J. W. Martin, M. MacLeod and I. T. Cousins, Modeling the Global Fate and Transport of Perfluorooctane Sulfonate (PFOS) and Precursor Compounds in Relation to Temporal Trends in Wildlife Exposure, Environ. Sci. Technol., 2009, 43, 9274–9280 CrossRef PubMed.
- L. Vierke, U. Berger and I. T. Cousins, Estimation of the acid dissociation constant of perfluoroalkyl carboxylic acids through an experimental investigation of their water-to-air transport, Environ. Sci. Technol., 2013, 47, 11032–11039 CrossRef PubMed.
- J. Cheng, E. Psillakis, M. R. Hoffmann and A. J. Colussi, Acid Dissociation versus Molecular Association of Perfluoroalkyl Oxoacids: Environmental Implications, J. Phys. Chem. A, 2009, 113, 8152–8156 CrossRef PubMed.
- K. U. Goss, The pKa values of PFOA and other highly fluorinated carboxylic acids, Environ. Sci. Technol., 2008, 42, 456–458 CrossRef PubMed.
- J. H. Johansson, H. Yan, U. Berger and I. T. Cousins, Water-to-air transfer of branched and linear PFOA: Influence of pH, concentration and water type, Emerging Contam., 2017, 3, 46–53 CrossRef.
- T. Wang, R. Vestergren, D. Herzke, J. Yu and I. T. Cousins, Levels, Isomer Profiles, and Estimated Riverine Mass Discharges of Perfluoroalkyl Acids and Fluorinated Alternatives at the Mouths of Chinese Rivers, Environ. Sci. Technol., 2016, 50, 11584–11592 CrossRef PubMed.
- Y. Shi, R. Vestergren, L. Xu, X. Song, X. Niu, C. Zhang and Y. Cai, Characterizing direct emissions of perfluoroalkyl substances from ongoing fluoropolymer production sources: A spatial trend study of Xiaoqing River, China, Environ. Pollut., 2015, 206, 104–112 CrossRef PubMed.
- C. A. Barton, M. A. Kaiser and M. H. Russell, Partitioning and removal of perfluorooctanoate during rain events: the importance of physical-chemical properties, J. Environ. Monit., 2007, 9, 839–846 RSC.
- K. Prevedouros, I. T. Cousins, R. C. Buck and S. H. Korzeniowski, Sources, fate and transport of perfluorocarboxylates, Environ. Sci. Technol., 2006, 40, 32–44 CrossRef PubMed.
- C. J. McMurdo, D. A. Ellis, E. Webster, J. Butler, R. D. Christensen and L. K. Reid, Aerosol enrichment of the surfactant PFO and mediation of the water–air transport of gaseous PFOA, Environ. Sci. Technol., 2008, 42, 3969–3974 CrossRef PubMed.
- M. Reth, U. Berger, D. Broman, I. T. Cousins, E. D. Nilsson and M. S. McLachlan, Water-to-air transfer of perfluorinated carboxylates and sulfonates in a sea spray simulator, Environ. Chem., 2011, 8, 381–388 Search PubMed.
- J. W. Martin, D. A. Ellis, S. A. Mabury, M. D. Hurley and T. J. Wallington, Atmospheric chemistry of perfluoroalkanesulfonamides: Kinetic and product studies of the OH radical and Cl atom initiated oxidation of N-ethyl perfluorobutanesulfonamide, Environ. Sci. Technol., 2006, 40, 864–872 CrossRef PubMed.
- J. C. D'Eon, M. D. Hurley, T. J. Wallington and S. A. Mabury, Atmospheric chemistry of N-methyl perfluorobutane sulfonamidoethanol, C4F9SO2N(CH3)CH2CH2OH: Kinetics and mechanism of reaction with OH, Environ. Sci. Technol., 2006, 40, 1862–1868 CrossRef.
- T. J. Wallington, M. D. Hurley, J. Xia, D. J. Wuebbles, S. Sillman, A. Ito, J. E. Penner, D. A. Ellis, J. Martin, S. A. Mabury, O. J. Nielsen and M. P. S. Andersen, Formation of C7F15COOH (PFOA) and other perfluorocarboxylic acids during the atmospheric oxidation of 8:2 fluorotelomer alcohol, Environ. Sci. Technol., 2006, 40, 924–930 CrossRef PubMed.
- K. Rankin, S. A. Mabury, T. M. Jenkins and J. W. Washington, A North American and global survey of perfluoroalkyl substances in surface soils: Distribution patterns and mode of occurrence, Chemosphere, 2016, 161, 333–341 CrossRef PubMed.
- J. J. MacInnis, K. French and D. C. G. Muir,
et al., Emerging investigator series: a 14-year depositional ice record of perfluoroalkyl substances in the high arctic, Environ. Sci.: Processes Impacts, 2017, 19(1), 22–30 Search PubMed.
- G. Sammut, E. Sinagra, R. Helmus and P. de Voogt, Perfluoroalkyl substances in the Maltese environment – (I) surface water and rain water, Sci. Total Environ., 2017, 589, 182–190 CrossRef PubMed.
-
E. Kissa, in Surfactant Science Series, ed. M. Dekker, New York, 2001, vol. 97 Search PubMed.
- US EPA, 2010/2015 PFOA Stewardship programme, https://www.epa.gov/assessing-and-managing-chemicals-under-tsca/fact-sheet-20102015-pfoa-stewardship-program, accessed December 8th, 2016.
-
J. P. Benskin, A. O. De Silva and J. W. Martin, in Reviews of Environmental Contamination and Toxicology, Springer, 2010, vol. 208, pp. 111–160 Search PubMed.
- L. Li, Z. Zhai, J. Liu and J. Hu, Estimating industrial and domestic environmental releases of perfluorooctanoic acid and its salts in China from 2004 to 2012, Chemosphere, 2015, 129, 100–109 CrossRef PubMed.
- W. Jiang, Y. Zhang, L. Yang, X. Chu and L. Zhu, Perfluoroalkyl acids (PFAAs) with isomer analysis in the commercial PFOS and PFOA products in China, Chemosphere, 2015, 127, 180–187 CrossRef PubMed.
- G. Shan, X. Chen and L. Zhu, Occurrence, fluxes and sources of perfluoroalkyl substances with isomer analysis in the snow of northern China, J. Hazard. Mater., 2015, 299, 639–646 CrossRef PubMed.
- J. P. Benskin, L. Ahrens, D. C. Muir, B. F. Scott, C. Spencer, B. Rosenberg, G. Tomy, H. Kylin, R. Lohmann and J. W. Martin, Manufacturing origin of perfluorooctanoate (PFOA) in Atlantic and Canadian Arctic seawater, Environ. Sci. Technol., 2011, 46, 677–685 CrossRef PubMed.
- A. O. De Silva, D. C. Muir and S. A. Mabury, Distribution of perfluorocarboxylate isomers in select samples from the North American environment, Environ. Toxicol. Chem., 2009, 28, 1801–1814 Search PubMed.
- S. Xie, T. Wang, S. Liu, K. C. Jones, A. J. Sweetman and Y. Lu, Industrial source identification and emission estimation of perfluorooctane sulfonate in China, Environ. Int., 2013, 52, 1–8 CrossRef PubMed.
- J. Löfstedt Gilljam, J. Leonel, I. T. Cousins and J. P. Benskin, Is ongoing Sulfluramid use in South America a significant source of perfluorooctane sulfonate (PFOS)? Production inventories, environmental fate, and local occurrence, Environ. Sci. Technol., 2015, 50(2), 653–659 CrossRef PubMed.
- J. P. Benskin, M. G. Ikonomou, M. B. Woudneh and J. R. Cosgrove, Rapid characterization of perfluoralkyl carboxylate, sulfonate, and sulfonamide isomers by high-performance liquid chromatography-tandem mass spectrometry, J. Chromatogr. A, 2012, 1247, 165–170 CrossRef PubMed.
- P. Labadie and M. Chevreuil, Partitioning behaviour of perfluorinated alkyl contaminants between water, sediment and fish in the Orge River (nearby Paris, France), Environ. Pollut., 2011, 159, 391–397 CrossRef PubMed.
- B. Chandramouli, J. P. Benskin, M. C. Hamilton and J. R. Cosgrove, Sorption of per- and polyfluoroalkyl substances (PFASs) on filter media: Implications for phase partitioning studies, Environ. Toxicol. Chem., 2015, 34, 30–36 CrossRef PubMed.
- J. P. Benskin, M. Bataineh and J. W. Martin, Simultaneous characterization of perfluoroalkyl carboxylate, sulfonate, and sulfonamide isomers by liquid chromatography-tandem mass spectrometry, Anal. Chem., 2007, 79, 6455–6464 CrossRef PubMed.
- S. Taniyasu, N. Yamashita, H.-B. Moon, K. Y. Kwok, P. K. Lam, Y. Horii, G. Petrick and K. Kannan, Does wet precipitation represent local and regional atmospheric transportation by perfluorinated alkyl substances?, Environ. Int., 2013, 55, 25–32 CrossRef PubMed.
- J. H. Johansson, U. Berger and I. T. Cousins, Can the use of deactivated glass fibre filters eliminate sorption artefacts associated with active air sampling of perfluorooctanoic acid?, Environ. Pollut., 2017, 224, 779–786 CrossRef PubMed.
-
J. H. Seinfeld and S. N. Pandis, Atmospheric Chemistry and Physics from Air Pollution to Climate Change, John Wiley & Sons, Hoboken, 2016 Search PubMed.
- T. Claquin, M. Schulz and Y. J. Balkanski, Modeling the mineralogy of atmospheric dust sources, J. Geophys. Res.: Atmos., 1999, 104, 22243–22256 CrossRef.
-
T. R. S. Wilson, in Chemical Oceanography, ed. J. P. Riley and G. Skirrow, Academic, Orlando, Fla, 2nd edn, 1975, vol. 1, pp. 365–413 Search PubMed.
- A. Dreyer, V. Matthias, I. Weinberg and R. Ebinghaus, Wet deposition of poly- and perfluorinated compounds in Northern Germany, Environ. Pollut., 2010, 158, 1221–1227 CrossRef PubMed.
- K. Y. Kwok, S. Taniyasu, L. W. Y. Yeung, M. B. Murphy, P. K. S. Lam, Y. Horii, K. Kannan, G. Petrick, R. K. Sinha and N. Yamashita, Flux of Perfluorinated Chemicals through Wet Deposition in Japan, the United States, And Several Other Countries, Environ. Sci. Technol., 2010, 44, 7043–7049 CrossRef PubMed.
- A. Kärrman, K. Elgh-Dalgren, C. Lafossas and T. Møskeland, Environmental levels and distribution of structural isomers of perfluoroalkyl acids after aqueous fire-fighting foam (AFFF) contamination, Environ. Chem., 2011, 8, 372–380 CrossRef.
- L. Zhao, M. Zhou, T. Zhang and H. Sun, Polyfluorinated and perfluorinated chemicals in precipitation and runoff from cities across eastern and central China, Arch. Environ. Contam. Toxicol., 2013, 64, 198–207 CrossRef PubMed.
- S. M. Vyas, I. Kania-Korwel and H.-J. Lehmler, Differences in the isomer composition of perfluoroctanesulfonyl (PFOS) derivatives, J. Environ. Sci. Health, Part A: Toxic/Hazard. Subst. Environ. Eng., 2007, 42, 249–255 CrossRef PubMed.
- M. Houde, G. Czub, J. M. Small, S. Backus, X. W. Wang, M. Alaee and D. C. G. Muir, Fractionation and Bioaccumulation of Perfluorooctane Sulfonate (PFOS) Isomers in a Lake Ontario Food Web, Environ. Sci. Technol., 2008, 42, 9397–9403 CrossRef PubMed.
- N. Yu, W. Shi, B. Zhang, G. Su, J. Feng, X. Zhang, S. Wei and H. Yu, Occurrence of Perfluoroalkyl Acids Including Perfluorooctane Sulfonate Isomers in Huai River Basin and Taihu Lake in Jiangsu Province, China, Environ. Sci. Technol., 2013, 47, 710–717 CrossRef PubMed.
- C. Eschauzier, J. Haftka, P. J. Stuyfzand and P. de Voogt, Perfluorinated Compounds in Infiltrated River Rhine Water and Infiltrated Rainwater in Coastal Dunes, Environ. Sci. Technol., 2010, 44, 7450–7455 CrossRef PubMed.
Footnote |
† Electronic supplementary information (ESI) available. See DOI: 10.1039/c8em00102b |
|
This journal is © The Royal Society of Chemistry 2018 |