Assessing the photovoltaic technology landscape: efficiency and energy return on investment (EROI)†
Received
28th June 2017
, Accepted 16th January 2018
First published on 16th January 2018
Abstract
This study builds on previous meta-analyses of photovoltaic (PV) systems to assess the tradeoff between efficiency and energy inputs (i.e. cumulative energy demand, CED) in the energetic performance (as measured by energy return on investment (EROI)) of PV technologies under both high-cost and low-cost balance of system scenarios. This study focuses on three existing technology groups (wafer, thin film, and organic). We find that earlier projections of third-generation (high-efficiency, low-cost), thin-film technologies have not yet emerged, since “third-generation” technologies currently have low-cost but also low-efficiency. However, we also find that the best advances in energetic performance to date come from thin film technology.
Introduction
Solar power is widely promoted as an important means to reduce harmful environmental impacts from electricity generation, particularly avoiding the emission of climate-changing greenhouse gases (GHGs). Governments worldwide support renewable energy by mandating renewable portfolio standards, tax incentives and feed-in tariffs.1 Due in part to government support and large reductions in module costs, the global installed capacity of solar photovoltaic (PV) systems is increasing rapidly.2
The energetic performance of solar cells is dependent on a number of factors: efficiency, lifetime, capacity factor, and energetic cost of cell manufacture. There is a large drive to boost the efficiency of PV cells via a number of techniques including improved light trapping;3 high-efficiency materials, such as gallium arsenide (GaAs);4 multiple-junction cells to capture more of the sunlight spectrum;5 multiple excitation generation and quantum dot cells;6 and plasmonic and hot carrier cells.7 Previous studies have highlighted both the energetic and climate benefits of PV systems with low embodied energy.8–10
This study explores the landscape of energetic performance of a variety of different PV technologies in terms of efficiency, embodied energy (as measured by cumulative energy demand, CED), and energy return on investment (EROI) to identify potential benchmarks for research and technology development.
Background
There has been a large push globally to reduce the financial costs of PV system production. The US Department of Energy (DOE) initiated the SunShot program, targeting a PV system cost of ‘one dollar per watt’ of installed capacity.11 Balance of system is usually considered as components and equipment aside from the PV modules themselves that among other functions convert DC energy, which is generated by the solar panel, to the AC energy system.12 At the outset of the program, the breakdown between financial cost of PV modules versus BOS costs was assumed to be around half–half (50
:
44%, the other 6% being power electronics) of the $3.40 per watt system cost. Other researchers have found a similar split (66
:
34) between module and BOS for conventional crystalline silicon (c-Si) PV technology, with an efficiency of 18%.13
Many of the costs of PV system production (especially BOS components such as support structures) scale with system area. As efficiency increases, the need to generate the same amount of electricity decreases. As such, system efficiency has become the holy grail of PV technology development. The DOE's National Renewable Energy Laboratory (NREL) tracks developments in the field by maintaining a ‘leaderboard’ of PV cell efficiency.14
A seminal work in the field of PV system analysis represents the tradeoff between efficiency [%] and panel cost [USD per m2] for three generations of PV: (I) wafer based; (II) thin film; and (III) advanced thin films.15 In this efficiency–cost space, diagonal lines depict the cost per unit of capacity [USD per W]. The first-generation, wafer-based technology has a higher efficiency than second-generation thin film but at a higher cost per unit area, leading to an overall higher cost per unit capacity. This seminal work projects that third-generation technologies would have dramatically increased efficiency through several approaches (such as multiple energy level, intermediate level cells, and multi carrier excitation), while the cost per unit area would be only slightly higher than traditional thin film.
However, efficiency and financial cost are not the only important metrics by which to judge PV system performance. Net energy performance and environmental impacts (such as GHG emissions or water consumption) may also be important indicators of the benefits and costs of energy delivery technologies.16,17 Previous meta-analyses have: (i) used GHG emissions,18,19 water consumption,20 and CED10,21 to compare PV technologies; (ii) used cumulative electrical energy demand (CEeD) [kW he/Wp] and electrical energy payback time (EePBT) [years] to assess the performance of the global PV industry;8,22 (iii) understood the impact of deploying energy storage technologies to support PV and wind;23 and (iv) compared wind, solar PV, and concentrating solar power (CSP) technologies.24 This paper uses the electrical energy return on investment (EeROI) as a metric to evaluate and compare the performance of different PV technologies with a specific focus on the impact of panel efficiency and BOS.
Another important financial metric that is increasingly used to assess the financial viability of solar PV technologies is the levelized cost of electricity (LOCE) over the full lifetime of the plant. Estimates range between $60–560 per MW h,25 with record-breaking-low bids being made in the United Arab Emirates of $23 per MW h.26
Methodology
Meta-analysis
We build on several previous meta-analyses of the energy inputs to PV systems and update them to find the distribution in CEeD for different PV technologies. For more discussion of the method, see the ESI,† Section S2, and the spreadsheet of research data taken from previous studies27–79 is also uploaded as ESI.† The meta-analysis is based mainly upon currently commercialized technologies, unfortunately meaning a lack of studies on perovskite technologies, which show a great deal of promise at the level of research cells.
Capacity factor
PV system electricity generation is dependent on the average power delivery capacity per watt of nameplate capacity, often termed the capacity factor [Wavg/Wp]. Previous studies have determined the global average capacity factor for PV systems to be around 12%.8,16 We have updated this assessment to obtain a value of 15% (for details see Section S2.1, ESI†).
Electrical energy return on investment
EeROI may be easily defined as | 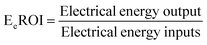 | (1) |
In the context of this study, energy inputs may be defined on a per unit area basis [kW h m−2] by the cumulative electrical energy demand of the PV system CEeDsys, which may be split into two parts for the module (CEeDmod) and the BOS (CEeDBOS). We assume that the energetic costs for operation and maintenance (O&M) as well as disposal are negligible, or that they vary little between different technologies. We may now write the expression for EeROI as | 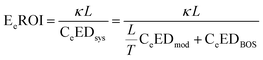 | (2) |
where κ is the capacity factor and L is the standard system lifetime [h], which is 24 × 365 × 25 = 219
000. T is the module life time [h].
Balance of system cost
In order to study the impact of module efficiency on energetic performance, we combine the BOS data (see Section S3.5, ESI†) of all technologies together and analyze two scenarios: (a) a high BOS scenario in which we assume that BOS energetic costs take the maximum value 206 kW he m−2 from the BOS distribution (see Fig. 1); and (b) a low BOS scenario in which BOS energetic costs take the minimum value of 37 kW he m−2.
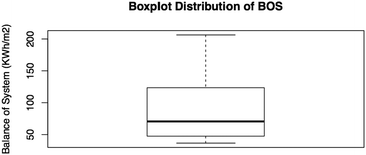 |
| Fig. 1 Distribution in the cumulative electrical energy demand for the balance of system (CEeDBOS) on a per unit area basis [kW he m−2] from our meta-analysis. The 0, 25, 50, 75, and 100 percentile values are, respectively, 36.58, 47.52, 70.59, 123.55, and 206.36 kW he m−2. | |
Lifetime
To compare different technologies on an equal basis, we define a standard lifetime of 25 years. For PV technologies with lower expected lifetimes, e.g. organic PV systems, CEeDmod will be increased to account for the replacement of panels over the 25 year period. For example, if the expected lifetime is 5 years, the CEeDmod would be increased by a factor of 5 because the panel would need to be replaced 5 times to cover the whole period, whereas we assume that the BOS does not need to be replaced. We do not account for any learning that may have decreased the value of CEeDmod, i.e., the panels are all paid for ‘up front’ at the beginning of the 25 period. We assume that organic modules have a lifetime of 5 years80 (i.e., they require the up-front investment for 5 modules). All other technologies are assumed to last 25 years.
Results and discussion
In Fig. 2, we plot the ‘PV energetic performance landscape’ for three sets of PV technologies: (1) crystalline silicon (c-SI), which includes single-crystal (sc), multi-crystalline (mc), and ribbon silicon; (2) thin film, which includes amorphous silicon (a-Si), cadmium telluride (CdTe), and copper indium gallium (di)selenide (CIGS); and (3) organic polymer (OPV). In the plot, the horizontal axis depicts PV module CEeDmod on a per unit area basis [kW he m−2], the vertical axis depicts PV module efficiency as a percent of incoming sunlight energy converted to DC electricity [%]. As can be seen, the pattern mirrors that of the efficiency vs. financial cost plot discussed earlier; wafer-based (first-generation) technologies have higher efficiency and higher energy ‘cost’ (CEeD) compared with thin-film and OPV (second-generation) technologies. As yet, no high-efficiency, low-CEeD (i.e. third-generation) panels have been produced.
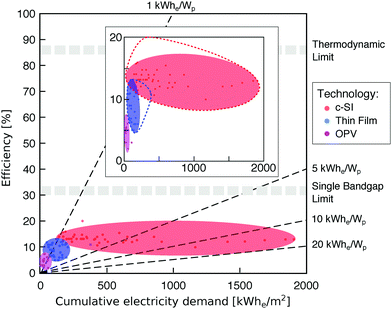 |
| Fig. 2 Energetic performance in terms of efficiency and cumulative electricity demand of three main photovoltaic types: crystalline silicon (c-SI in red), thin film (blue), and organic (OPV, in pink). The inset figure shows the detailed efficiency range between 0 and 20%. The dashed lines show the whole boundaries of each technology. | |
In Fig. 3, we compare the energetic performance of the PV technologies under a high-BOS (left) and low-BOS (right) scenario now using the efficiency-CEeDsys ‘landscape’, i.e. including the CEeDBOS costs. In this case, we are using a log–log plot wherein the EeROI values are depicted as diagonal contours and vary depending on the (high–low) BOS scenario. Switching between the two scenarios changes the position and slope of the EeROI contours in the landscape. The slope of the EeROI contours suggests that low efficiency is more detrimental at high CEeDsys, since the curve bends upward more steeply on the right side of the plot, especially in the high-BOS scenario.
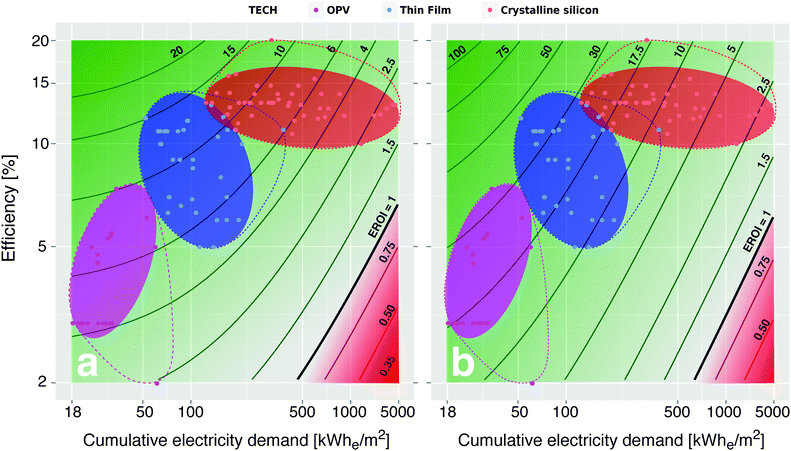 |
| Fig. 3 PV system performance landscape (cumulative electricity demand, efficiency, and EROI) for different scenarios for the CEeD of the balance of system (BOS). (a) A high BOS scenario, value 206 kW he m−2 and (b) a low BOS scenario, value 37 kW he m−2. Outliers of each technology group are captured using a dashed line. As can be seen, the EROI is vastly different in the two scenarios; however, thin-film technologies (CdTe, CIGS, a-Si) perform best in both cases. The higher efficiency of the c-Si technologies (sc-Si, mc-Si) comes at the cost of a higher cumulative electricity demand for the total system. | |
In both scenarios, thin-film technologies perform best (i.e. have the highest and generally higher EeROI values). Additionally, the best gains in energetic performance of the technologies occur when efficiency gains and reduction in CEeDsys move the technology through the landscape perpendicularly to the EeROI contours. As can be seen from the shape and direction of the bounding ovals, OPV technologies are seeing efficiency gains but at the expense of greater CEeDsys, c-Si is reducing CEeDsys, but with smaller relative gains in efficiency, whereas thin-film is seeing concomitant gains in efficiency and a reduction in CEeDsys.
Conclusions
This study builds on previous meta-analyses of PV systems to assess the trade-off between efficiency and cumulative energy demand in the energetic performance of PV technologies under both a high-cost and low-cost balance of system scenario. We find that earlier projections of third-generation (high-efficiency, low-cost), thin-film technologies have not yet emerged. We further find that, of the existing technology groups (wafer, thin film, and organic), thin-film has, to date, seen the best advances in energetic performance and is currently performing better.
Conflicts of interest
There are no conflicts to declare.
Acknowledgements
The authors acknowledge the support of the Environmental Engineering & Earth Sciences Department at Clemson University, without whose support this would not have been possible.
Notes and references
- K. H. Solangi,
et al., A review on global solar energy policy, Renewable Sustainable Energy Rev., 2011, 15(4), 2149–2163 CrossRef.
- F. Dincer, Renewable Sustainable Energy Rev., 2011, 15(1), 713–720 CrossRef.
- E. Garnett and P. Yang, Light trapping in silicon nanowire solar cells, Nano Lett., 2010, 10(3), 1082–1087 CrossRef CAS PubMed.
- M. Konagai, M. Sugimoto and K. Takahashi, High efficiency GaAs thin film solar cells by peeled film technology, J. Cryst. Growth, 1978, 45, 277–280 CrossRef CAS.
- J. Gilot, M. M. Wienk and R. A. J. Janssen, Double and triple junction polymer solar cells processed from solution, Appl. Phys. Lett., 2007, 90(14), 143512 CrossRef.
- A. J. Nozik, Quantum dot solar cells, Phys. E, 2002, 14(1), 115–120 CrossRef CAS.
- C. Clavero, Plasmon-induced hot-electron generation at nanoparticle/metal-oxide interfaces for photovoltaic and photocatalytic devices, Nat. Photonics, 2014, 8(2), 95–103 CrossRef CAS.
- M. Dale and S. M. Benson, Environ. Sci. Technol., 2012, 47, 3482 CrossRef PubMed.
- C. J. M. Emmott, N. J. Ekins-Daukes and J. Nelson, Energy Environ. Sci., 2014, 7, 1810 CAS.
- R. H. E. M. Koppelaar, Renewable Sustainable Energy Rev., 2017, 72, 1241–1255 CrossRef.
-
S. Chu, US Department of Energy Dollar a Watt Workshop, Washington, DC, 2010 Search PubMed.
- Q. Li and P. Wolfs, A review of the single phase photovoltaic module integrated converter topologies with three different DC link configurations, IEEE Trans. Power Electron., 2008, 23(3), 1320–1333 CrossRef.
-
R. M. Swanson, Stanford Energy Seminar, November 14, 2011, http://https://energy.stanford.edu/events/solar-energy-mini-series-silicon-photovoltaic-roadmap.
- NREL, Research Cell Record Efficiency Chart, National Renewable Energy Laboratory, http://www.nrel.gov/pv/.
- G. Conibeer, Mater. Today, 2007, 10, 42 CrossRef CAS.
- M. Raugei and E. Leccisi, Energy Policy, 2016, 90, 46–59 CrossRef.
- M. Carbajales-Dale, C. J. Barnhart, A. R. Brandt and S. M. Benson, Nat. Clim. Change, 2014, 4(7), 524–527 CrossRef.
- H. C. Kim, V. Fthenakis, J. K. Choi and D. E. Turney, J. Ind. Ecol., 2012, 16(s1), S110–S121 CrossRef CAS.
- D. D. Hsu, P. O’Donoughue, V. Fthenakis, G. A. Heath, H. C. Kim, P. Sawyer, J. K. Choi and D. E. Turney, J. Ind. Ecol., 2012, 16(s1), S122–S135 CrossRef CAS.
-
J. Macknick, J. Meldrum, S. Nettles-Anderson, G. Heath and A. Miara, (2014), Photovoltaic Specialist Conference (PVSC), 2014 IEEE 40th, pp. 1458–1460.
- K. P. Bhandari, J. M. Collier, R. J. Ellingson and D. S. Apul, Renewable Sustainable Energy Rev., 2015, 47, 133–141 CrossRef.
- A. Louwen, W. G. Van Sark, A. P. Faaij and R. E. Schropp, Nat. Commun., 2016, 7, 13728 CrossRef CAS PubMed.
- M. Carbajales-Dale, C. J. Barnhart and S. M. Benson, Energy Environ. Sci., 2014, 7, 1538 CAS.
- M. Dale, Appl. Sci., 2013, 3, 325 CrossRef.
-
OpenEI (2017) Transparent Cost Database, Open Energy Information, URL: http://https://openei.org/apps/TCDB/, accessed 09/21/2017.
-
L. A. Graves, (2016), Record low bids submitted for Abu Dhabi's 350MW solar plant in Sweihan, The National UAE Edition, Sept 19 2016, URL: http://https://www.thenational.ae/business/record-low-bids-submitted-for-abu-dhabi-s-350mw-solar-plant-in-sweihan-1.213135, accessed 09/21/2017.
-
A. Anctil, et al., Life-cycle assessment of organic solar cell technologies, Photovoltaic Specialists Conference (PVSC), 2010 35th IEEE. IEEE, 2010.
- E. A. Alsema, Energy pay-back time and CO2 emissions of PV systems, Prog. Photovoltaics Res. Appl., 2000, 8(1), 17–25 CrossRef CAS.
-
E. A. Alsema, M. J. de Wild-Scholten and V. M. Fthenakis, Environmental impacts of PV electricity generation-a critical comparison of energy supply options, 21st European Photovoltaic Solar Energy Conference, 2006.
- M. B. Amor,
et al., Can distributed generation offer substantial benefits in a Northeastern American context? A case study of small-scale renewable technologies using a life cycle methodology, Renewable Sustainable Energy Rev., 2010, 14(9), 2885–2895 CrossRef.
- R. Battisti and A. Corrado, Evaluation of technical improvements of photovoltaic systems through life cycle assessment methodology, Energy, 2005, 30(7), 952–967 CrossRef CAS.
- P. N. Botsaris and F. Filippidou, Estimation of the energy payback time (EPR) for a PV module installed in North Eastern Greece, Appl. Sol. Energy, 2009, 45(3), 166–175 CrossRef.
- R. H. Crawford, Life cycle energy and greenhouse emissions analysis of wind turbines and the effect of size on energy yield, Renewable Sustainable Energy Rev., 2009, 13(9), 2653–2660 CrossRef.
- S. B. Darling and F. You, The case for organic photovoltaics, RSC Adv., 2013, 3(39), 17633–17648 RSC.
-
M. de Wild-Scholten and E. A. Alsema, Environmental life cycle inventory of crystalline silicon photovoltaic module production, MRS Online Proceedings Library Archive, 2005, p. 895.
- M. J. M. de Wild-Scholten, Energy payback time and carbon footprint of commercial photovoltaic systems, Sol. Energy Mater. Sol. Cells, 2013, 119, 296–305 CrossRef CAS.
- N. Espinosa,
et al., A life cycle analysis of polymer solar cell modules prepared using roll-to-roll methods under ambient conditions, Sol. Energy Mater. Sol. Cells, 2011, 95(5), 1293–1302 CrossRef CAS.
- N. Espinosa,
et al., OPV for mobile applications: an evaluation of roll-to-roll processed indium and silver free polymer solar cells through analysis of life cycle, cost and layer quality using inline optical and functional inspection tools, J. Mater. Chem. A, 2013, 1(24), 7037–7049 CAS.
- N. Espinosa and F. C. Krebs, Life cycle analysis of organic tandem solar cells: When are they warranted?, Sol. Energy Mater. Sol. Cells, 2014, 120, 692–700 CrossRef CAS.
-
V. M. Fthenakis, E. A. Alsema and M. J. de Wild-Scholten, Life cycle assessment of photovoltaics: Perceptions, needs, and challenges, Photovoltaic Specialists Conference, 2005. Conference Record of the Thirty-first IEEE. IEEE, 2005.
-
V. Fthenakis, et al., Update of PV energy payback times and life-cycle greenhouse gas emissions, 24th European Photovoltaic Solar Energy Conference and Exhibition, 2009.
- V. M. Fthenakis and H. C. Kim, Photovoltaics: Life-cycle analyses, Sol. Energy, 2011, 85(8), 1609–1628 CrossRef CAS.
-
V. Fthenakis, et al., Life cycle analysis of high-performance monocrystalline silicon photovoltaic systems: energy payback times and net energy production value, 27th European Photovoltaic Solar Energy Conference and Exhibition, 2012.
- R. García-Valverde, J. A. Cherni and A. Urbina, Life cycle analysis of organic photovoltaic technologies, Prog. Photovoltaics Res. Appl., 2010, 18(7), 535–558 CrossRef.
-
R. Gløckner, et al., Environmental life cycle assessment of the Elkem Solar metallurgical process route to solar grade silicon with focus on energy consumption and greenhouse gas emissions, Silicon for the Chemical and Solar Industry IX, Oslo, Norway, 2008.
- D. Gürzenich and H.-J. Wagner, Cumulative energy demand and cumulative emissions of photovoltaics production in Europe, Energy, 2004, 29(12), 2297–2303 CrossRef.
- M. Held and R. Ilg, Update of environmental indicators and energy payback time of CdTe PV systems in Europe, Prog. Photovoltaics Res. Appl., 2011, 19(5), 614–626 CrossRef CAS.
- M. Ito,
et al., A comparative study on cost and life-cycle analysis for 100 MW very large-scale PV (VLS-PV) systems in deserts using m-Si, a-Si, CdTe, and CIS modules, Prog. Photovoltaics Res. Appl., 2008, 16(1), 17–30 CrossRef CAS.
- M. Ito,
et al., A comparative study on life cycle analysis of 20 different PV modules installed at the Hokuto mega-solar plant, Prog. Photovoltaics Res. Appl., 2011, 19(7), 878–886 CrossRef CAS.
- N. Jungbluth,
et al., Life cycle assessment for emerging technologies: case studies for photovoltaic and wind power, Int. J. Life Cycle Assess., 2005, 10(1), 24–34 CrossRef CAS.
- R. Kannan,
et al., Life cycle assessment study of solar PV systems: an example of a 2.7 kW p distributed solar PV system in Singapore, Sol. Energy, 2006, 80(5), 555–563 CrossRef CAS.
- K. Kato, A. Murata and K. Sakuta, An evaluation on the life cycle of photovoltaic energy system considering production energy of off-grade silicon, Solar energy materials and solar cells, 1997, 47(1–4), 95–100 CrossRef CAS.
-
G. A. Keoleian and G. M. D. Lewis, Application of life-cycle energy analysis to photovoltaic module design, 1997.
-
K. E. Knapp, T. L. Jester and G. B. Mihaiik, Energy balances for photovoltaic modules: status and prospects, Photovoltaic Specialists Conference, 2000, Conference Record of the Twenty-Eighth IEEE, IEEE, 2000.
- C. Koroneos, N. Stylos and N. Moussiopoulos, LCA of Multicrystalline Silicon Photovoltaic Systems, Int. J. Life Cycle Assess., 2006, 11(3), 183–188 CrossRef CAS.
- S. Krauter and R. Rüther, Considerations for the calculation of greenhouse gas reduction by photovoltaic solar energy, Renewable Energy, 2004, 29(3), 345–355 CrossRef CAS.
- F. Kreith, P. Norton and D. Brown, A comparison of CO2 emissions from fossil and solar power plants in the United States, Energy, 1990, 15(12), 1181–1198 CrossRef CAS.
- R. Laleman, J. Albrecht and J. Dewulf, Life cycle analysis to estimate the environmental impact of residential photovoltaic systems in regions with a low solar irradiation, Renewable Sustainable Energy Rev., 2011, 15(1), 267–281 CrossRef.
-
F. Lenzmann, et al., Refined life-cycle assessment of polymer solar cells, 26th European Photovoltaic Solar Energy Conference and Exhibition, 2011.
- J. E. Mason,
et al., Energy payback and life-cycle CO2 emissions of the BOS in an optimized 3· 5 MW PV installation, Prog. Photovoltaics Res. Appl., 2006, 14(2), 179–190 CrossRef CAS.
-
J. Mathur, et al., Energy and environmental analysis of wind energy systems, World Renewable Energy Congress VI, 2000.
- J. Mathur, N. K. Bansal and H.-J. Wagner, Energy and environmental correlation for renewable energy systems in India, Energy Sources, 2002, 24(1), 19–26 CrossRef.
- N. J. Mohr,
et al., Life cycle assessment of thin-film GaAs and GaInP/GaAs solar modules, Prog. Photovoltaics Res. Appl., 2007, 15(2), 163–179 CrossRef CAS.
- T. Muneer,
et al., Life cycle assessment of a medium-sized photovoltaic facility at a high latitude location, Proc. Inst. Mech. Eng., Part A, 2006, 220(6), 517–524 CrossRef CAS.
- A. Nishimura,
et al., Life cycle assessment and evaluation of energy payback time on high-concentration photovoltaic power generation system, Appl. Energy, 2010, 87(9), 2797–2807 CrossRef CAS.
- M. Oliver and T. Jackson, Energy and economic evaluation of building-integrated photovoltaics, Energy, 2001, 26(4), 431–439 CrossRef.
-
S. Pacca, D. Sivaraman and G. A. Keoleian, Life cycle assessment of the 33 kW photovoltaic system on the Dana Building at the University of Michigan: Thin film laminates, multi-crystalline modules, and balance of system components, University of Michigan, 2006 Search PubMed.
- W. Palz and H. Zibetta, Energy pay-back time of photovoltaic modules, International Journal of Solar Energy, 1991, 10(3–4), 211–216 CrossRef.
- O. Perpiñan,
et al., Energy payback time of grid connected PV systems: comparison between tracking and fixed systems, Progress in Photovoltaics: Research and Applications, 2009, 17(2), 137–147 CrossRef.
- R. Prakash and N. K. Bansal, Energy analysis of solar photovoltaic module production in India, Energy Sources, 1995, 17(6), 605–613 CrossRef.
- M. Raugei, S. Bargigli and S. Ulgiati, Life cycle assessment and energy pay-back time of advanced photovoltaic modules: CdTe and CIS compared to poly-Si, Energy, 2007, 32(8), 1310–1318 CrossRef CAS.
- M. Raugei and P. Frankl, Life cycle impacts and costs of photovoltaic systems: current state of the art and future outlooks, Energy, 2009, 34(3), 392–399 CrossRef.
- A. L. Roes,
et al., Ex-ante environmental and economic evaluation of polymer photovoltaics, Prog. Photovoltaics Res. Appl., 2009, 17(6), 372–393 CrossRef CAS.
- H. Schaefer and G. Hagedorn, Hidden energy and correlated environmental characteristics of PV power
generation, Renewable Energy, 1992, 2(2), 159–166 CrossRef CAS.
- A. Stoppato, Life cycle assessment of photovoltaic electricity generation, Energy, 2008, 33(2), 224–232 CrossRef CAS.
-
M. J. de Wild-Scholten, et al., LCA comparison of the Elkem Solar metallurgical route and conventional gas routes to solar silicon, Proceedings of the 23rd European photovoltaic solar energy conference, Valencia, Spain, 2008.
- K. Yamamoto,
et al., A high efficiency thin film silicon solar cell and module, Solar Energy, 2004, 77(6), 939–949 CrossRef CAS.
- D. Yue,
et al., Deciphering the uncertainties in life cycle energy and environmental analysis of organic photovoltaics, Energy Environ. Sci., 2012, 5(11), 9163–9172 CAS.
- P. Zhai and E. D. Williams, Dynamic hybrid life cycle assessment of energy and carbon of multicrystalline silicon photovoltaic systems, Environ. Sci. Technol., 2010, 44(20), 7950–7955 CrossRef CAS PubMed.
- J. Kalowekamo and E. Baker, Estimating the manufacturing cost of purely organic solar cells, Sol. Energy, 2009, 83(8), 1224–1231 CrossRef CAS.
Footnote |
† Electronic supplementary information (ESI) available. See DOI: 10.1039/c7ee01806a |
|
This journal is © The Royal Society of Chemistry 2018 |
Click here to see how this site uses Cookies. View our privacy policy here.