DOI:
10.1039/C7AN01706E
(Paper)
Analyst, 2018,
143, 297-303
Highly sensitive detection of hesperidin using AuNPs/rGO modified glassy carbon electrode†
Received
16th October 2017
, Accepted 14th November 2017
First published on 15th November 2017
Abstract
The highly sensitive and selective electrochemical sensor of hesperidin based on gold nanoparticles (AuNPs) and reduced graphene oxide (rGO) modified glassy carbon electrode (GCE) is reported. The AuNPs and rGO were uniformly introduced on the surface of the GCE via electrodeposition without any reducing agents and have been characterized by scanning electron microscopy (SEM), energy dispersive X-ray spectrometry (EDX), X-ray photoelectron spectroscopy (XPS), FT-IR, and electrochemical methods. The AuNPs/rGO not only promoted the accumulation of hesperidin onto the GCE surface for quantitative analysis but also accelerated the electron transfer between hesperidin and the electrode substrates. Under the optimal conditions, hesperidin was determined quantitatively at the AuNPs/rGO/GCE using amperometric i–t curve. The results showed that the current obtained on detection of hesperidin exhibited a linear correlation with its concentration in the range of 5.0 × 10−8 mol L−1–8.0 × 10−6 mol L−1 with a detection limit of 8.2 × 10−9 mol L−1 (S/N = 3). In addition, good specificity, repeatability, reproducibility, and long-term storage stability were achieved for the modified electrode, which could be used for the detection of hesperidin in the traditional Chinese medicine, Pericarpium Citri Reticulatae.
Introduction
Pericarpium Citri Reticulatae (PCR) is a type of widely used traditional Chinese medicines due to its beneficial health effects in strengthening stomach, drying damp, eliminating phlegm, etc.1,2 Of all the flavonoids contained in PCR, hesperidin (Scheme 1) with a stable property is usually selected as an ideal marker to quantitatively determine the content of PCR,3,4 which promotes its broader application prospects in the fields of medicine and food industry, for example, as anticancer, antioxidant, and food additives.1,4–6 During the past decades, the majority of methods used for determining hesperidin are based on chromatography or chromatography equipped with other detectors,3,7–12 as well as spectroscopic methods.13,14 However, their high cost and complicated operation limit their extensive application to a certain degree. Comparatively speaking, electrochemical techniques are known as an ideal alternate due to the advantages of instrument simplicity, low-cost, high sensitivity, and fast response. For example, mesoporous SiO2 modified carbon paste electrode (mesoporous SiO2/CPE),4 reduced graphene oxide on single-wall carbon nanotubes modified glassy carbon electrode (rGO/SWCNTs/GCE),15 and adsorptive stripping voltammetry at bare hanging mercury drop electrode (HMDE)16 were developed for the electrochemical detection of hesperidin. However, the electrochemical determination of hesperidin using gold nanoparticles (AuNPs)/reduced graphene oxide (rGO) modified electrode has not been reported.
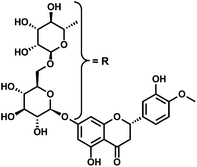 |
| Scheme 1 The molecular structure of hesperidin. | |
Graphene, a honeycomb-like and sp2-hybridized 2D carbon sheet, has attracted increasing attention in the study of electrochemical properties and the preparation of relevant sensors owing to its excellent conductivity, robust mechanical properties as well as large surface area.17–25 In addition, AuNPs as a type of metal nanoparticles have distinctive physicochemical properties of high conductivity, large active sites, good biocompatibility, etc.26–34 Moreover, they have also been extensively studied in fabricating sensitive electrochemical sensors. In view of the above advantages, rGO-AuNPs should provide good opportunities for applications in the fields of electrocatalysis, sensors, etc.
Herein, the electrochemical sensor is fabricated based on rGO and AuNPs on the GCE through an electrodeposition technique, denoted as AuNPs/rGO/GCE, which will be used for the rapid detection of hesperidin. Compared with the reported electrochemical methods for the detection of hesperidin,4,7,15,16,35 the sensor prepared in this study exhibits wider linear range and lower detection limit (LOD), as confirmed from the data presented in Table S1.† This method provides a novel platform for the determination hesperidin and also opens up a new possibility for the widespread use of electrochemical sensors to determine the presence of hesperidin in traditional Chinese medicines.
Results and discussion
Characterization of the AuNPs/rGO nanocomposite
The prepared nanocomposite was characterized by SEM, XPS, FT-IR, EDX, and cyclic voltammetry (CV) methods. The surface morphology of the GCE, rGO/GCE, and AuNPs/rGO/GCE was characterized by SEM. On comparing the SEM images in Fig. 1A and B, it can be observed that the electrodeposited rGO layer is uniformly covered on the smooth GCE surface. Furthermore, the spherical AuNPs (a diameter of 30–40 nm) are embedded on the surface of the rGO layer to form a three-dimensional structure (Fig. 1C). These images indicate that AuNPs are effectively deposited with an even size on the GCE surface with rGO. The creased nature of rGO provides a high specific surface area for the modified electrode, which is beneficial to the adsorption of hesperidin. Moreover, the obtained three-dimensional structure can improve the electrochemical performance of the electrode, as it possesses higher effective surface area and improved charge transfer ability.
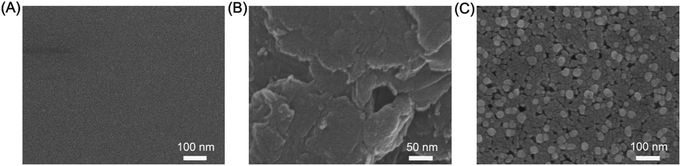 |
| Fig. 1 The SEM images of bare GCE (A), rGO/GCE (B), and AuNPs/rGO/GCE (C). | |
In order to confirm the product in the first electrodeposition step and obtain its bonding structure, the XPS and FT-IR spectroscopies of GO and rGO were carried out. Fig. 2A and B show the XPS analysis of GO and rGO. The C 1s spectrum of GO displays three peaks at 284.5, 286.4, and 288.9 eV (Fig. 2A), corresponding to the C–C/C
C in aromatic rings, C–O (epoxy and alkoxy), and C(
O)–(O–H) groups, respectively. However, as shown in Fig. 2B, the peak corresponding to C–O (epoxy and alkoxy) decreases drastically, which indicates that most of the oxygen-containing functional groups are removed after the reduction, confirming that the electrodeposited product in the first step is rGO.36 Moreover, the FT-IR spectroscopy also exhibits different absorption features of GO and rGO. For example, the vibration and deformation peaks of O–H groups at 3395 cm−1 and 1410 cm−1, the C
O stretching vibrations at 1726 cm−1, the epoxy C–O stretching vibrations at 1226 cm−1, and the alkoxy C–O stretching vibrations at 1052 cm−1 in GO decrease significantly and some of them disappear entirely after GO was reduced by the electrochemical technique. Furthermore, in order to confirm the chemical composition of the product in the second electrodeposited step, the EDX analysis was conducted for the rGO/GCE and AuNPs/rGO/GCE (Fig. 2D). The former only presents the signal for C, while the latter exhibits the signals for C and Au. The apparent peak of Au indicates that AuNPs have been successfully grafted onto the rGO/GCE surface.
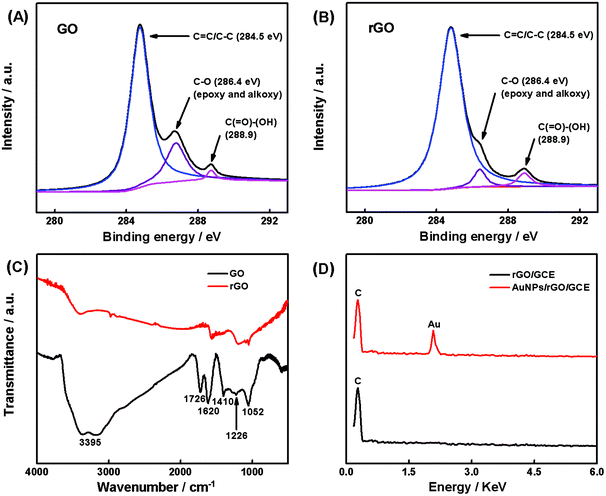 |
| Fig. 2 The XPS spectra of C 1s of GO (A) and rGO (B), the FT-IR spectra of GO and rGO (C), and the EDX analysis of rGO/GCE and AuNPs/rGO/GCE (D). | |
In addition, CV method was applied to characterize the electrochemical property of the prepared nanocomposite. Fig. 3A shows the cyclic voltammograms of the bare GCE, rGO/GCE, and AuNPs/rGO/GCE electrodes in a 0.5 mol L−1 H2SO4 solution. The rGO/GCE displays a reduction peak at −0.18 V (vs. SCE), which is due to the phenolic hydroxyl groups on graphene.37 Such a reduction peak is also observed in the CV curve of the AuNPs/rGO/GCE, accompanied by the characteristic peaks for Au (0.94 V). This indicates that rGO and AuNPs were formed by the electrodeposition technique. The surface chemical properties of the bare and modified GCEs were further studied in 0.1 mol L−1 KCl containing 1 mmol L−1 K3[Fe(CN)6]/K4[Fe(CN)6] as a redox probe. As shown in Fig. 3B, the bare GCE has a pair of typical redox peaks (curve 1) and the peak current increases clearly after rGO or AuNPs are deposited onto the GCE surface (curves 2 and 3). A further increase in the peak current is also obtained for the AuNPs/rGO/GCE (curve 4). These data evidently demonstrate that the modified GCE has more electrochemical active sites than the bare GCE, which is ascribed to the large surface area of rGO and the excellent conductivity of AuNPs.
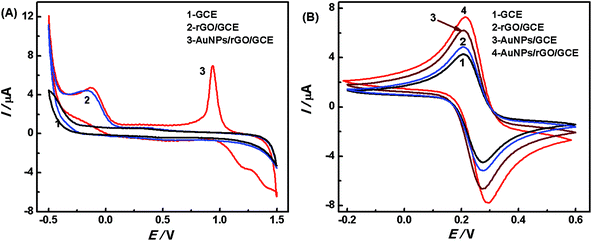 |
| Fig. 3 CVs of the electrodes in 0.5 mol L−1 H2SO4 (A) and 0.1 mol L−1 KCl containing 1 mmol L−1 K3[Fe(CN)6]/K4[Fe(CN)6] (B) at a scan rate of 50 mV s−1. | |
Electrochemical behavior of hesperidin on the modified electrodes
Optimization of the experimental conditions.
Effect of scan rates.
Generally speaking, the useful information involved in the electrochemical mechanism can be obtained from the relationship of the peak current (I) and the scan rate (ν). Therefore, the effect of the scan rate on the oxidation of hesperidin was examined. Fig. 4A presents the cyclic voltammograms of 1.0 × 10−7 mol L−1 hesperidin in the HAc–NaAc solution (pH 3.6) on the AuNPs/rGO/GCE with different scan rates. It can be observed that the oxidation peak currents (Ipa) at 1.21 V increase along with the rise of the scan rate. The linear relationship (Fig. 4B) of Ipa and ν can be expressed as:
Ipa(μA) = −6.2861 − 0.1160ν (mV s−1) (R2 = 0.9935) |
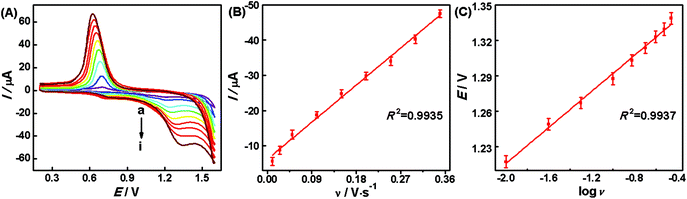 |
| Fig. 4 The CV curves of 1.0 × 10−7 mol L−1 hesperidin on AuNPs/rGO/GCE with the scan rates from 10 to 350 mV s−1 (curves a–i, 10, 25, 50, 100, 150, 200, 250, 300, and 350 mV s−1) in the HAc–NaAc solution (pH 3.6) (A), the plot of the peak current I vs. the scan rate ν (B), and the plot of the peak potential E vs. the logarithm of scan rate log ν (C). | |
The above results indicate that the electrochemical reaction of hesperidin on the AuNPs/rGO/GCE is an adsorption-controlled process, which promotes the accumulation of hesperidin onto the AuNPs/rGO/GCE surface for the quantitative analysis. According to the Laviron theory,38 the calculated adsorption quantity on the surface of the AuNPs/rGO/GCE is 3.06 × 10−9 mol cm−2 for the oxidation reaction of hesperidin.
In addition, with the increase of the scan rates, the oxidation peak potentials (Epa) shift positively. The linear relationships of Epa and log
ν (Fig. 4C) are described as the following regression equation:
Epa(V) = 1.3682 + 0.0760 log ν (R2 = 0.9937) |
The slope of the linear equation is equal to 2.303RT/αnF, where α and n refer to the electron transfer coefficient and the number of the transferred electrons, respectively. According to the above equation, the calculated transferred electron number is 2 for the oxidation reaction of hesperidin when the value of α for the irreversible reaction is assumed to be 0.5.
Effect of pH values.
The influence of the solution pH ranging from 2.8 to 5.6 on the electrochemical response of 5.0 × 10−7 mol L−1 hesperidin at the AuNPs/rGO/GCE was studied in the HAc–NaAc solution and the results are shown in Fig. 5. The absolute values of the oxidation peak currents of hesperidin increase clearly from 7.45 μA to 15.17 μA as the pH values decrease from 5.6 to 3.6, but the oxidation peak currents decrease slightly from 15.17 μA to 14.37 μA as the pH values decrease from 3.6 to 2.8. Hence, pH 3.6 was selected as the optimal value for the buffer solution to perform all experiments as it results in the largest peak currents with well-defined wave shapes. In addition, the oxidation peak potentials are shifted to more negative values with the increase of the pH values, which indicates that protons take part in the electrochemical reaction of hesperidin. The linear relationship between the peak potential and pH is plotted, E(V) = 1.3905–0.0524 pH (R2 = 0.9951), as shown in Fig. 5B. The slope of the regression equation is close to the theoretical value of −0.059 V pH−1, indicating that the electrochemical reaction of hesperidin at the AuNPs/rGO/GCE should involve an equal number of protons and electrons being transferred, which is two protons and two electrons.
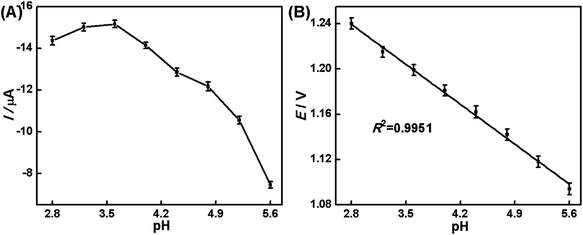 |
| Fig. 5 The influence of pH value on the peak current (A) and the peak potential (B) of 5.0 × 10−7 mol L−1 hesperidin. | |
Combining the above results, it can be concluded that the electrochemical reaction of hesperidin at the AuNPs/rGO/GCE is a two-electron and two-proton transfer process. The possible reaction of hesperidin at the AuNPs/rGO/GCE is described in Scheme 2.
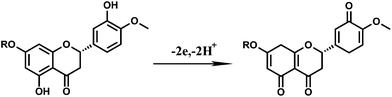 |
| Scheme 2 Mechanism of the redox process of hesperidin at AuNPs/rGO/GCE. | |
Electrochemical determination of hesperidin
Under the optimal conditions, CV is used for the detection of hesperidin. Fig. 6A shows the CV response of the AuNPs/rGO/GCE in the absence (curve 1) and the presence (curve 2) of 5.0 × 10−7 mol L−1 hesperidin in the HAc–NaAc buffer solution (pH 3.6). In the absence of hesperidin, the AuNPs/rGO/GCE exhibits a characteristic peak for AuNPs at 0.64 V and a wide and small oxidation peak at 1.21 V. However, a good electrochemical behavior is observed in the presence of hesperidin. On comparing curve 1 and curve 2, it can be observed that the characteristic peaks of Au are similar, while the oxidation peak at 1.21 V is enhanced greatly after the addition of hesperidin and a new and small oxidation peak appears at 0.71 V. The two oxidation peaks indicate that two hydroxyls at different benzene rings of hesperidin can be electro-oxidized on the AuNPs/rGO/GCE. This phenomenon proves that the modified electrode is electrochemically active for detection of hesperidin and the AuNPs/rGO can improve the accumulation of hesperidin from the solution to the sensor surface, thus enhancing the sensitivity for the determination.
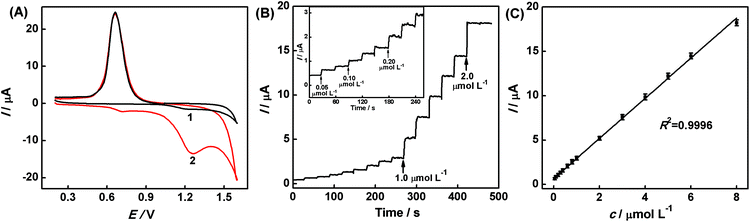 |
| Fig. 6 The CV response of the AuNPs/rGO/GCE in the absence (curve 1) and the presence (curve 2) of 5.0 × 10−7 mol L−1 hesperidin in the HAc–NaAc buffer solution at the scan rate of 50 mV s−1 (A). Amperometric i–t curve response of the AuNPs/rGO/GCE with different concentrations of hesperidin from 5.0 × 10−8–8.0 × 10−6 mol L−1 (the concentration of each injection is 0.05, 0.05, 0.10, 0.10, 0.10, 0.20, 0.20, 0.20, 1.0, 1.0, 1.0, 1.0, 1.0, and 2.0 μmol L−1) in the HAc–NaAc buffer solution at 1.21 V (B). Plot of current vs. the concentrations of hesperidin (C) for the AuNPs/rGO/GCE. | |
Fig. 6B shows the typical amperometric i–t curve of the AuNPs/rGO/GCE upon successive addition of different concentrations (5.0 × 10−8–8.0 × 10−6 mol L−1) of hesperidin to the HAc–NaAc buffer solution (pH 3.6) under stirring at 1.21 V. Upon the addition of hesperidin, a good and well-defined amperometric response is observed and the currents increase linearly with the increase in hesperidin concentration. The corresponding linear regression equation can be described as follows (Fig. 6C): I(μA) = 0.6361 + 2.2658c μmol L−1 (R2 = 0.9996). The linear range, LOD, and sensibility of the resultant sensor are estimated to be 5.0 × 10−8 mol L−1–8.0 × 10−6 mol L−1, 8.2 × 10−9 mol L−1 (S/N = 3), and 18.04 A mol L−1 cm−2, respectively.
Practical application of the constructed sensor
The selectivity of the hesperidin sensor is more important particularly in the presence of other chemicals with similar structural features. Hence, the interference test was carried out by recording the CV response of the AuNPs/rGO/GCE in 1.0 × 10−6 mol L−1 hesperidin in the presence of 1.0 × 10−4 mol L−1 interferents (Fig. 7A). It is observed that the prepared AuNPs/rGO/GCE delivers a well-defined response towards 1.0 × 10−6 mol L−1 hesperidin, but no significant responses are observed for the 100-fold concentrations of glucose (GLU), riboflavin (VB2), vitamin C (VC), glycine (GLY), and L-serine (L-SER). Hence, the existence of excess interferents mentioned above does not affect the determination of hesperidin (deviations are below 5%), indicating the good selectivity of the AuNPs/rGO/GCE for hesperidin.
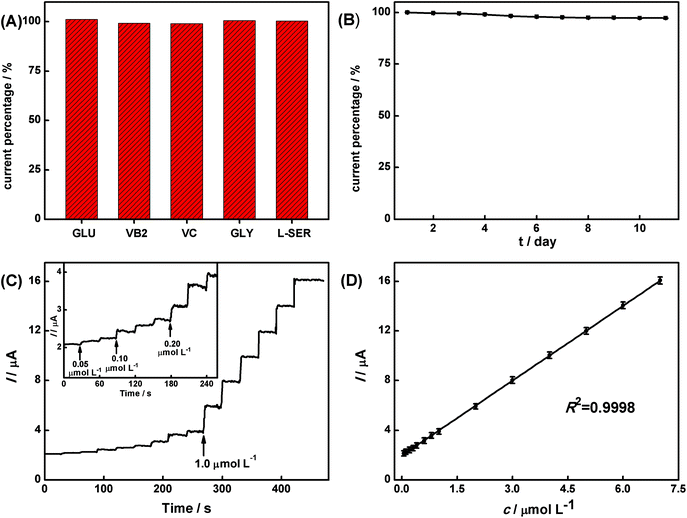 |
| Fig. 7 Effects of the interferents on the hesperidin detection for AuNPs/rGO/GCE (A). The vertical axis “current percentage/%” refers to the percentage of the current in the presence and the absence of the interferents; the current response of the AuNPs/rGO/GCE toward 1.0 × 10−6 mol L−1 hesperidin day-to-day (B). The i–t response of the AuNPs/rGO/GCE with the concentration of hesperidin from 5.0 × 10−8–7.0 × 10−6 mol L−1 (the concentration of each injection is 0.05, 0.05, 0.10, 0.10, 0.10, 0.20, 0.20, 0.20, 1.0, 1.0, 1.0, 1.0, 1.0, and 1.0 μmol L−1) in the real sample at 1.21 V (C). Plot of current vs. the concentration of hesperidin for the AuNPs/rGO/GCE (D). | |
The reproducibility of the method used to prepare the AuNPs/rGO/GCE was explored by fabricating five different electrodes independently and comparing their response to hesperidin. The results indicate that the electrochemical deposition method possesses good reproducibility with a relative standard deviation (RSD) of 3.7%. In addition, a long-term stability of the AuNPs/rGO/GCE was also evaluated from 1 to 11 days (Fig. 7B). Clearly, the response of the sensor still remains about 97.3% of its initial value, indicating the good stability of the AuNPs/rGO/GCE.
In order to develop a viable practical method, the analytical response of hesperidin in PCR was analyzed under the optimized conditions by a standard addition method as shown in Fig. 7C and D. According to the calibration curves of Fig. 7D, the linear regression equation can be described as follows:
I(μA) = 1.9809 + 2.1085c μmol L−1 (R2 = 0.9998) |
The linear range, LOD, and sensibility of the prepared sensor for the real sample were estimated to be 7.0 × 10−6–5.0 × 10−8 mol L−1, 9.7 × 10−9 mol L−1 (S/N = 3), and 15.99 A mol L−1 cm−2, respectively. In addition, the recovery experiments were also performed by adding a series of known concentrations of hesperidin to the sample solution (Table 1). The value of the recovery was in the range from 96.7% to 105.0% with a RSD from 1.44% to 2.85%. These results indicate that the proposed sensor is satisfactory for the determination of hesperidin in traditional Chinese medicine, PCR.
Table 1 Recovery studies of hesperidin from PCR
Added (μmol L−1) |
Found (μmol L−1) |
Recovery (%) |
RSD (%) |
0 |
0.061 |
— |
1.44 |
0.020 |
0.082 |
105.0 |
2.85 |
0.030 |
0.090 |
96.7 |
1.91 |
0.040 |
0.100 |
97.5 |
1.58 |
Conclusions
In this study, the AuNPs and rGO were uniformly introduced at the surface of GCE to fabricate the AuNPs/rGO/GCE by an electrochemical deposition method without any reducing agents. The successful formation of the AuNPs/rGO/GCE was confirmed by SEM, EDX, XPS, FT-IR, and electrochemical methods. Under the optimal conditions, hesperidin was determined at the AuNPs/rGO/GCE using the i–t curve in the laboratory samples and in a real sample, Pericarpium Citri Reticulatae. The AuNPs/rGO/GCE exhibited a low detection limit of 8.2 × 10−9 mol L−1 (S/N = 3) for hesperidin. In general, the sensor provides a high sensitivity for the direct detection of hesperidin in real sample due to its low detection limit, wide linear range, long-term stability as well as good selectivity. As a conventional, convenient, and cost-effective sensor, it presents a great potential for determining the trace amounts of hesperidin in the fields of pharmacy, biology, etc.
Conflicts of interest
There are no conflicts to declare.
Acknowledgements
This work was supported by the National Natural Science Foundation of China (no. 21705103), the Doctor Fund of Shanxi Normal University (0505/02070298), Natural Science Foundation of Shanxi Normal University (0505/02080123), and the 1331 Engineering of Shanxi Province.
References
-
The People's Republic of China Pharmacopoeia, China Chemistry Industry Publishing House, 2005, vol. I Search PubMed.
- A. Garg, S. Garg, L. J. D. Zaneveld and A. K. Singla, Phytother. Res., 2001, 15, 655–659 CrossRef CAS PubMed.
- J. Xia, A. Kotani, H. Hakamata and F. Kusu, J. Pharm. Biomed. Anal., 2006, 41, 1401–1405 CrossRef CAS PubMed.
- D. Sun, F. R. Wang, K. B. Wu, J. W. Chen and Y. K. Zhou, Microchim. Acta, 2009, 167, 35–39 CrossRef CAS.
- Y. Y. Peng, F. H. Liu and J. N. Ye, J. Chromatogr. B: Anal. Technol. Biomed. Life Sci., 2006, 830, 224–230 CrossRef CAS PubMed.
- A. Masek, M. Zaborski and E. Chrzescijanska, Food Chem., 2011, 127, 699–704 CrossRef CAS PubMed.
- G. Chen, L. Y. Zhang, J. Q. Zhao and J. N. Ye, Anal. Bioanal. Chem., 2002, 373, 169–173 CrossRef CAS PubMed.
- P. Dugo, M. Lo Presti, M. Öhman, A. Fazio, G. Dugo and L. Mondello, J. Sep. Sci., 2005, 28, 1149–1156 CrossRef CAS PubMed.
- S. Gorinstein, D. Huang, H. Leontowicz, M. Leontowicz, K. Yamamoto, R. SolivaFortuny, O. M. Belloso, A. L. M. Ayala and S. Trakhtenberg, Acta Chromatogr., 2006, 17, 108–124 CAS.
- D. Cautela, B. Laratta, F. Santelli, A. Trifirò, L. Servillo and D. Castaldo, J. Agric. Food Chem., 2008, 56, 5407–5414 CrossRef CAS PubMed.
- V. Bhatt, S. Sharma, N. Kumar, U. Sharma and B. Singh, J. Pharm. Biomed. Anal., 2017, 132, 46–55 CrossRef CAS PubMed.
- M. S. Sammani, S. Clavijo, L. Portugal, R. Suárez, H. Seddik and V. Cerdà, Talanta, 2017, 167, 695–702 CrossRef CAS PubMed.
- J. Vuković, S. Matsuoka, K. Yoshimura, V. Grdinić and R. J. Grubešić, Microchim. Acta, 2007, 159, 277–285 CrossRef.
- D. Srilatha, M. Nasare, B. Nagasandhya, V. Prasad and P. Diwan, ISRN Spectrosc., 2013, 2013, 1–4 CrossRef.
- J. J. Wu, L. Wang, Q. Q. Wang, L. N. Zhou and B. X. Ye, Talanta, 2016, 150, 61–70 CrossRef CAS PubMed.
- J. B. Hu, Q. L. Li and X. C. Tan, Anal. Lett., 2006, 29, 1779–1789 CrossRef.
- K. S. Novoselov, A. K. Geim, S. V. Morozov, D. Jiang, Y. Zhang, S. V. Dubonos, I. V. Grigorieva and A. A. Firsov, Science, 2004, 306, 666–669 CrossRef CAS PubMed.
- A. K. Geim and K. S. Novoselov, Nat. Mater., 2007, 6, 183–191 CrossRef CAS PubMed.
- Y. X. Xu, H. Bai, G. W. Lu, C. Li and G. Q. Shi, J. Am. Chem. Soc., 2008, 130, 5856–5857 CrossRef CAS PubMed.
- C. N. R. Rao, A. K. Sood, K. S. Subrahmanyam and A. Govindaraj, Angew. Chem., Int. Ed., 2009, 48, 7752–7777 CrossRef CAS PubMed.
- D. A. C. Brownson and C. E. Banks, Analyst, 2010, 135, 2768–2778 RSC.
- L. Hua, X. Q. Wu and R. Wang, Analyst, 2012, 137, 5716–5719 RSC.
- M. M. I. Khan, A. M. J. Haque and K. Kim, J. Electroanal. Chem., 2013, 700, 54–59 CrossRef CAS.
- M. L. Yola, N. Atar, T. Eren, H. Karimi–Maleh and S. Wang, RSC Adv., 2015, 5, 65953–65962 RSC.
- Z. G. Liu, H. D. Forsyth, N. Khaper and A. C. Chen, Analyst, 2016, 141, 4074–4083 RSC.
- N. Chauhan, A. Singh, J. Narang, S. Dahiyab and C. S. Pundir, Analyst, 2012, 137, 5113–5122 RSC.
- X. C. Fu, J. Wu, L. Nie, C. G. Xie, J. H. Liu and X. J. Huang, Anal. Chim. Acta, 2012, 720, 29–37 CrossRef CAS PubMed.
- H. Liu, X. Su, C. Y. Duan, X. N. Dong, S. L. Zhou and Z. F. Zhu, J. Electroanal. Chem., 2014, 731, 36–42 CrossRef CAS.
- M. L. Yola, T. Eren and N. Atar, Sens. Actuators, B, 2015, 210, 149–157 CrossRef CAS.
- S. Y. Guo, L. Xu, B. B. Xu, Z. X. Sun and L. H. Wang, Analyst, 2015, 140, 820–826 RSC.
- R. Sedghi and Z. Pezeshkian, Sens. Actuators, B, 2015, 219, 119–124 CrossRef CAS.
- X. Sun, Z. Jiang, H. Wang and H. Zhao, Int. J. Electrochem. Sci., 2015, 10, 9714–9724 CAS.
- J. Zhao, W. J. Guo, M. S. Pei and F. Ding, Anal. Methods, 2016, 8, 4391–4397 RSC.
- S. Palanisamy, K. Thangavelu, S. M. Chen, B. Thirumalraj and X. H. Liu, Sens. Actuators, B, 2016, 233, 298–306 CrossRef CAS.
- X. Xu, X. Qi, J. Yang and G. Chen, Chem. Sens., 2003, 23, 58–61 CAS.
- J. L. Zhang, H. J. Yang, G. X. Shen, P. Cheng, J. Y. Zhang and S. W. Guo, Chem. Commun., 2010, 46, 1112–1114 RSC.
- Y. Zhang, J. Zheng and M. D. Guo, Chin. J. Chem., 2016, 34, 1268–1276 CrossRef CAS.
- E. Laviron, J. Electroanal. Chem., 1974, 52, 355–393 CrossRef CAS.
Footnote |
† Electronic supplementary information (ESI) available. See DOI: 10.1039/c7an01706e |
|
This journal is © The Royal Society of Chemistry 2018 |
Click here to see how this site uses Cookies. View our privacy policy here.