DOI:
10.1039/C7TX00236J
(Paper)
Toxicol. Res., 2018,
7, 102-116
The hypothalamic–pituitary–testicular axis and the testicular function are modulated after silver nanoparticle exposure†
Received
31st August 2017
, Accepted 22nd November 2017
First published on 23rd November 2017
Abstract
Silver nanoparticles (AgNPs) are widely used in industrial and medical applications and humans may be exposed through different routes, increasing the risk of toxicity. We investigated the transcript expression of genes involved in the regulation of the hypothalamic–pituitary–testicular (HPT) axis and the parameters associated with sperm functionality after prepubertal exposure. AgNPs modulated the transcript expression of genes involved in the control of the HPT axis and spermatogenesis in the groups treated with lower doses, while the functional parameters related to sperm and puberty were affected in the groups administered higher doses. These results suggest that the HPT axis is disrupted by AgNPs during the prepubertal and pubertal periods, which are highly susceptible windows for the endocrine-disrupting chemical activity.
Introduction
Silver nanoparticles (AgNPs) have potent antimicrobial activity against a wide range of bacteria, including highly pathogenic and multidrug resistant species such as Staphylococcus aureus, Salmonella typhi, Staphylococcus epidermidis and Escherichia coli.1,2 For this reason, AgNPs are included in medical products such as coatings for burn wound dressings, surgical devices, and bone prostheses,3–6 as well as in other consumer products such as toothpaste, cosmetics, sprays, disinfectants, deodorants, diapers, food packaging materials and water purifiers.7–11 Currently, there are approximately 442 different products that contain AgNPs in their chemical composition.12
Therefore, humans may be exposed to AgNPs present in everyday nano-functionalized consumer products.13 Recently, it was demonstrated that AgNP-impregnated toothbrushes release silver, which may cause human and environmental exposure to AgNPs.14 Furthermore, Quadros et al.15 tested the amount of AgNPs leached by infant products (plush toys, baby blankets, and sippy cups) and found that 43.8% of the AgNPs were leached from the spout cover of sippy cups, which represents 0.93 ± 0.02 mg per kg product per day. The toxic effects of silver in humans (argyria) are associated with high levels of exposure during life, but the safety limits of exposure to AgNPs are still under evaluation, because there is very little information available and more risk assessment studies are necessary.16,17
The toxic effects caused by inhalation,18 ingestion,19–22 dermal contact23,24 or intravenous injection25,26 have been described after the in vivo administration of AgNPs in animal models. These toxic effects include the inhibition of mitochondrial activity,27,28 cytotoxicity,29 genotoxicity30 and the production of reactive oxygen species, which causes oxidative stress in the central nervous system.31,32
Regarding reproductive toxicity, in vitro exposure of mammalian spermatogonial stem cells to AgNPs damages the cell membrane, induces apoptosis and necrosis,33 and promotes the decline in cellular proliferation.34 Rabbits exposed intravenously to AgNPs have decreased sperm motility and increased production of reactive oxygen species.35 Furthermore, studies in rats showed that oral exposure to AgNPs reduces sperm production22 and integrity, compromises the integrity of plasma and acrosome membranes, reduces the mitochondrial activity in the mid-piece of the sperm, and alters the reproductive behavior.21
Healthy spermatozoa are the final product of a complex process controlled by the hypothalamic–pituitary–testicular (HPT) axis. In this context, this study sought to investigate the molecular mechanisms involved in the dose–response effects of four dosages of AgNPs (1.875, 3.75, 7.5 or 15 μg AgNPs per kg) on the regulation of the HPT axis and the testicular function, using prepubertal male Wistar rats as an experimental model. During the entire experimental period (from postnatal day (PND) 23 through PND60) the animals were weighed daily to follow their growth, and upon reaching puberty the weight and age were recorded.
The secretion of gonadotropins is finely regulated by the hypothalamus, where the pulse frequency of gonadotropin-releasing hormone (GnRH) controls the differential expression of LH and FSH subunit genes by the gonadotrophs in the pituitary.36,37 Thus, the hypothalamus was evaluated for the transcript expression (mRNA) of gonadotropin releasing hormone 1 (Gnrh1); and the pituitary was evaluated for the transcript expression of gonadotropin releasing hormone receptor (Gnrhr), luteinizing hormone beta polypeptide (Lhb), and follicle stimulating hormone beta subunit (Fshb). Additionally, the serum LH and FSH concentrations were measured in the blood.
The negative feedback mechanism was investigated in both the hypothalamus and pituitary by the transcript expression of the androgen receptor (Ar), estrogen receptor 1 (Esr1) and estrogen receptor 2 (Esr2), since these receptors may be involved in controlling the synthesis and release of GnRH and LH.38 The transcript expression of inhibin B (Inhbb) in the testis was also analyzed, because of its participation in the negative feedback of FSH.39
Once in the blood, LH stimulates the synthesis of testosterone by binding to the luteinizing hormone/choriogonadotropin receptor (LHCGR) in the Leydig cells in the testis.40 Thus, the testes were evaluated for the transcript expression of Lhcgr and the serum testosterone concentration was measured in the blood. In the Sertoli cells, testosterone binds to the androgen receptor (AR) or is converted into estradiol by the aromatase (CYP19A1) enzyme, both of these events are related to the spermatogenesis process.40 Therefore, the relative transcript expressions of Ar and Cyp19a1 were evaluated in the testis and the serum estradiol concentration was measured in the blood.
Spermatogenesis events take place in the Sertoli cells, and are mainly influenced by the action of FSH, which binds to the follicle stimulating hormone receptor (FSHR) to perform its action.40 Estradiol is another important hormone for the spermatogenesis process, interacting with estrogen receptors (ESR1 and ESR2) located in the nucleus and cytoplasm or with G protein-coupled estrogen receptor 1 (GPER) in the plasma membrane, which is crucial for the regulation of proteins involved in the function of the testis.41 In this sense, we evaluated the transcript expression of Fshr, Esr1, Esr2 and Gper in the testis.
Finally, the sperm production, sperm reserves and sperm transit time in the epididymis segments were evaluated. The sperm functionality was assessed by the verification of acrosome integrity, plasma membrane integrity, sperm defects and mitochondrial activity, since these methods more accurately indicate subfertility and infertility, which reflect the ability of the sperm to fertilize the oocyte.42–44
Results
Characterization of AgNPs
Prior to initiating the experimental protocol it was necessary to determine the AgNP diameter and polydispersity indexes before and after dilution of the commercial solution. The dilution did not alter the particle diameter, which was estimated to be 79.5 ± 11 nm and the polydispersity index measured was 0.575 ± 118. The stability of AgNPs in simulated gastric fluid is presented in ESI Table 1.†
The effects of prepubertal AgNP exposure on the body growth, onset of puberty and reproductive organ weights
Following AgNP prepubertal exposure the rats were routinely weighed to monitor body growth, and had no effect on any of the treated groups, as compared to controls (ESI Fig. 1†). However, the onset of puberty was delayed in the groups receiving AgNP dosages 7.5 and 15 μg kg−1, when compared to the controls (Fig. 1). Furthermore, the weights of the testis, epididymis segments, seminal vesicle and seminal fluid were not affected by any of the treatment dosages (Table 1).
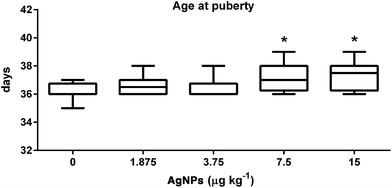 |
| Fig. 1 Age at puberty onset for rats exposed to 0 (control), 15, 7.5, 3.75 or 1.875 μg of AgNPs per kg BW. The values are expressed as median and interquartile ranges (Kruskal–Wallis followed by the post-test of Dunn), *P < 0.05 vs. control, n = 12 per group. AgNPs: silver nanoparticles; BW: body weight. | |
Table 1 The testis, epididymis, seminal vesicle and seminal fluid relative weights in animals exposed to silver nanoparticles (AgNPs)
Organ weight (mg per 100 g BW) |
AgNPs (μg kg−1) |
0 |
1.875 |
3.75 |
7.5 |
15 |
Data were subjected to analysis of variance and are expressed as mean ± standard error of the mean; n = 12 animals per group. |
Testis |
487 ± 18 |
468 ± 7 |
494 ± 14 |
488 ± 10 |
524 ± 10 |
Caput + corpus epididymis |
69 ± 1.5 |
64 ± 1.4 |
73 ± 1.5 |
74 ± 2 |
67 ± 2 |
Cauda epididymis |
44.5 ± 1.2 |
47 ± 0.8 |
49 ± 1.2 |
42 ± 0.8 |
44 ± 1.5 |
Seminal vesicle undrained |
232 ± 9 |
208 ± 14 |
223 ± 8 |
214 ± 11 |
198 ± 6 |
Seminal fluid |
123 ± 8 |
139 ± 16 |
134 ± 7 |
125 ± 9 |
109 ± 6 |
Effects of AgNPs on gonadotropin production
Towards the goal of understanding how AgNPs modulate gonadotropin production, we first examined the transcript expression of Gnrh in the hypothalamus, since this can influence the expressions of LH and FSH in the pituitary. As shown in Fig. 2A, only the 3.75 μg AgNP per kg-treated group displayed an increase in Gnrh expression, as compared to controls; however, the transcript expression of Gnrhr in the pituitary was unaffected (Fig. 2B).
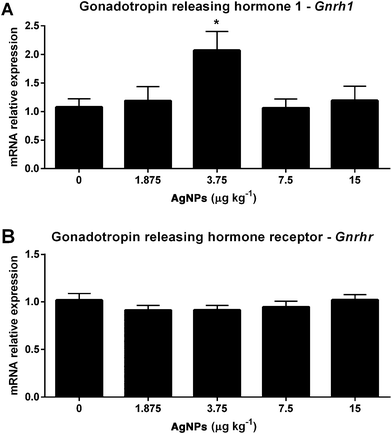 |
| Fig. 2 Transcript expression of Gnrh (A) and Gnrhr (B) for rats exposed to 0 (control), 15, 7.5, 3.75 or 1.875 μg of AgNPs per kg BW. The Rpl19 was used as an internal control. The values are expressed as the mean ± S.E.M. (ANOVA followed by the post-test of Dunnett), *P < 0.05 vs. control, n = 12 per group. Gnrh: Gonadotropin releasing hormone; Gnrhr: gonadotropin releasing hormone receptor; Rpl19: ribosomal protein L19; AgNPs: silver nanoparticles; BW: body weight; SEM: standard error of the mean. | |
While the Lhb transcript expression in the pituitary did not change in any of the treated groups (Fig. 3A), the serum concentrations of LH increased in the 3.75 μg AgNP per kg-treated group, as compared to the controls (Fig. 3B). There were also no significant changes in the Fshb transcript expression in the pituitary (Fig. 3C), yet the serum concentrations of FSH increased in the 1.875 μg AgNP per kg-treated group, when compared to the controls (Fig. 3D).
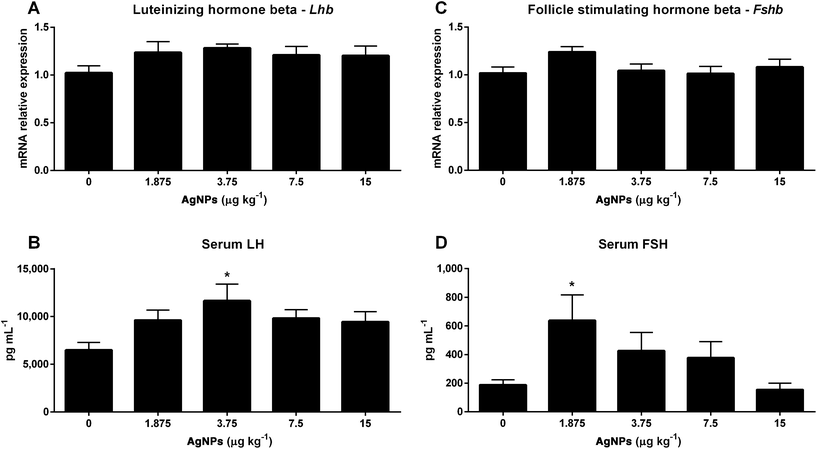 |
| Fig. 3 Pituitary transcript expression of Lhb (A) and Fshb (C), serum concentrations of LH (B) and FSH (D) for rats exposed to 0 (control), 15, 7.5, 3.75 or 1.875 μg of AgNPs per kg BW. The Rpl19 was used as an internal control. The values are expressed as the mean ± S.E.M. (ANOVA followed by the post-test of Dunnett), *P < 0.05 vs. control, n = 12 per group. Lhb: luteinizing hormone beta polypeptide; Fshb: follicle stimulating hormone beta subunit; LH: lutropin; FSH: follitropin; Rpl19: ribosomal protein L19; AgNPs: silver nanoparticles; BW: body weight; SEM: standard error of the mean. | |
Effects of AgNPs on the expression of genes controlling the negative feedback mechanisms in the hypothalamic–pituitary–testicular axis and estradiol receptors
Next we investigated molecular components of the HPT axis that are involved in the negative feedback mechanism regulating the synthesis and secretion of GnRH, LH and FSH. In the hypothalamus, the Ar transcript expression was not altered in any of the groups treated with AgNPs (Fig. 4A). However, the Esr1 transcript expression was decreased in the 7.5 μg AgNP per kg-treated group (Fig. 4B), and the Esr2 transcript expression was decreased in the 1.875, 3.75 and 7.5 μg AgNP per kg-treated groups, when compared to the controls (Fig. 4C).
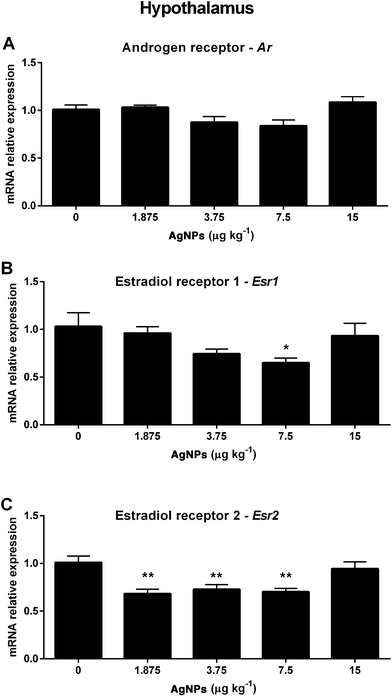 |
| Fig. 4 Hypothalamic transcript expression of Ar (A), Esr1 (B) and Esr2 (C) for rats exposed to 0 (control), 15, 7.5, 3.75 or 1.875 μg of AgNPs per kg BW. The Rpl19 was used as an internal control. The values are expressed as the mean ± S.E.M. (ANOVA followed by the post-test of Dunnett), *P < 0.05 and **P < 0.01 vs.control, n = 12 per group. Ar: androgen receptor 1; Esr1: estradiol receptor 1; Esr2: estradiol receptor 2; Rpl19: ribosomal protein L19; AgNPs: silver nanoparticles; BW: body weight; SEM: standard error of the mean. | |
In the pituitary, the Ar transcript expression was decreased in the 1.875, 3.75 and 7.5 μg AgNP per kg-treated groups (Fig. 5A), the Esr1 transcript expression was not altered in any of the treated groups (Fig. 5B) and the Esr2 transcript expression was decreased in the 1.875, 3.75 and 7.5 μg AgNP per kg-treated groups, as compared to the controls (Fig. 5C).
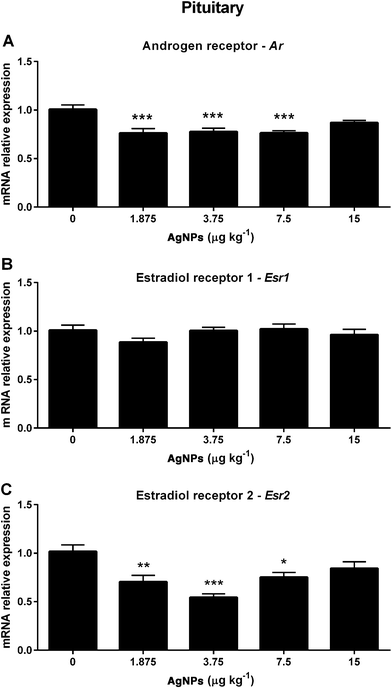 |
| Fig. 5 Pituitary transcript expression of Ar (A), Esr1 (B) and Esr2 (C) for rats exposed to 0 (control), 15, 7.5, 3.75 or 1.875 μg of AgNPs per kg BW. The Rpl19 was used as an internal control. The values are expressed as the mean ± S.E.M. (ANOVA followed by the post-test of Dunnett), *P < 0.05, **P < 0.01 and ***P < 0.001 vs. control, n = 12 per group. Ar: androgen receptor 1; Esr1: estradiol receptor 1; Esr2: estradiol receptor 2; Rpl19: ribosomal protein L19; AgNPs: silver nanoparticles; BW: body weight; SEM: standard error of the mean. | |
In the testis, the Inhbb transcript expression was found to be increased only in the 7.5 μg AgNP per kg-treated group, when compared to controls (Fig. 6).
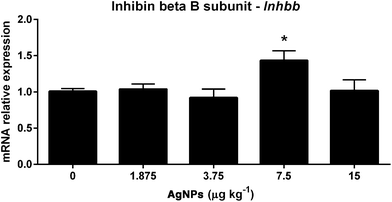 |
| Fig. 6 Testicular transcript expression of Inhbb for rats exposed to 0 (control), 15, 7.5, 3.75 or 1.875 μg of AgNPs per kg BW. The Rpl19 was used as an internal control. The values are expressed as the mean ± S.E.M. (ANOVA followed by the post-test of Dunnett), *P < 0.05 vs. control, n = 12 per group. Inhbb: Inhibin beta B subunit; Rpl19: ribosomal protein L19; AgNPs: silver nanoparticles; BW: body weight; SEM: standard error of the mean. | |
Effects of AgNPs on testicular steroidogenesis
Having established that the onset of puberty and that the transcript expression levels of some of the molecular components involved in gonadotropin synthesis and secretion were modulated, we then investigated the effects of AgNP exposure on testicular steroidogenesis. The Lhcgr transcript expression in the testis was not altered in any of the groups treated with AgNPs (Fig. 7A). An increase in the serum concentrations of testosterone was observed in the 1.875 μg AgNP per kg-treated group, when compared to the controls (Fig. 7B). Interestingly, the estradiol serum concentration was increased in the 7.5 AgNP per kg-treated group (Fig. 7D), despite not being able to detect a significant increase (p = 0.08) in the transcript expression of aromatase (Cyp19a1) for this group, when compared to the controls (Fig. 7C).
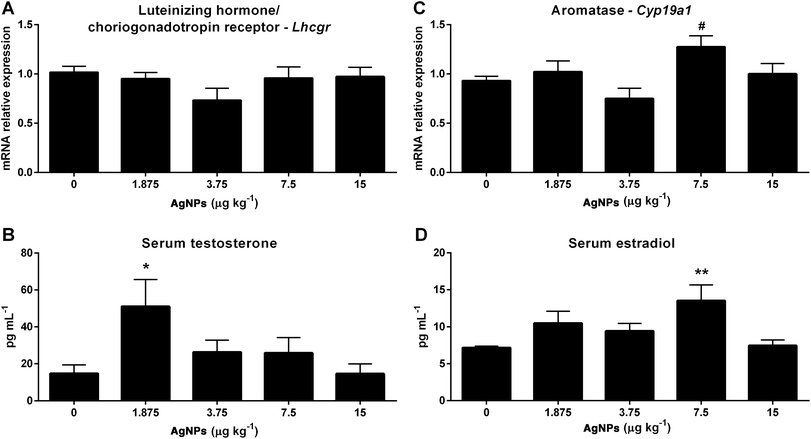 |
| Fig. 7 Testicular transcript expression of Lhcgr (A) and Cyp19a1 (C), serum concentrations of testosterone (B) and estradiol (D), for rats exposed to 0 (control), 15, 7.5, 3.75 or 1.875 μg of AgNPs per kg BW. The Rpl19 was used as an internal control. The values are expressed as the mean ± S.E.M. (ANOVA followed by the post-test of Dunnett), *P < 0.05 and **P < 0.01 vs. control, n = 12 per group. Lhcgr: luteinizing hormone/choriogonadotropin receptor; Cyp19a1: aromatase; Rpl19: ribosomal protein L19; AgNPs: silver nanoparticles; BW: body weight; SEM: standard error of the mean. | |
Effects of AgNPs on the expression of genes related to spermatogenesis
Spermatogenesis relies heavily on the action of FSH and the estrogen receptors; thus the transcript expression levels were measured. The Ar transcript expression was reduced in the 1.875 and 3.75 μg AgNP per kg-treated groups compared to the control (Fig. 8A), whereas the Fshr transcript expression in the testis was not altered (Fig. 8B). In relation to the estradiol receptors, the transcript expression of Gper (Fig. 9A) was reduced in the 1.875 μg AgNP per kg-treated group, and the transcript expression of Esr1 was reduced in the 1.875 and 3.75 μg AgNP per kg-treated groups, when compared to the controls (Fig. 9B). On the other hand, the expression of Esr2 was not affected by AgNP treatment (Fig. 9C).
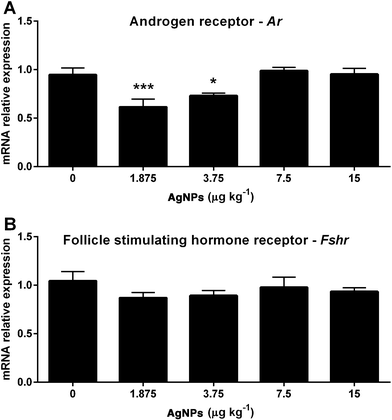 |
| Fig. 8 Testicular transcript expression of Ar (A) and Fshr (B) for rats exposed to 0 (control), 15, 7.5, 3.75 or 1.875 μg of AgNPs per kg BW. The Rpl19 was used as an internal control. The values are expressed as the mean ± SEM; ANOVA followed by the post-test of Dunnett; *P < 0.05 and ***P < 0.001 vs. control, n = 12 animals per group; Ar: androgen receptor; Fshr: follicle stimulating hormone receptor; Rpl19: ribosomal protein L19; AgNPs: silver nanoparticles; BW: body weight; SEM: standard error of the mean. | |
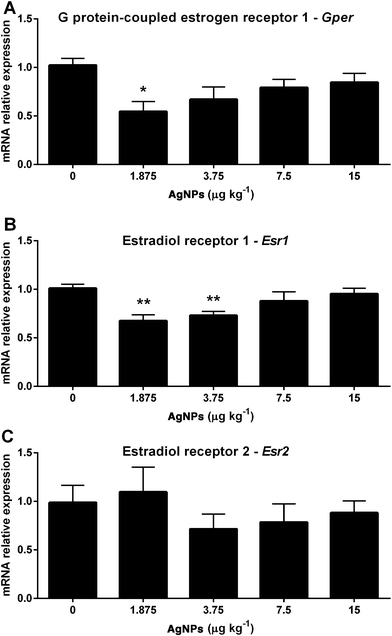 |
| Fig. 9 Testicular transcript expression of Gper (A), Esr1 (B) and Esr2 (C) for rats exposed to 0 (control), 15, 7.5, 3.75 or 1.875 μg of AgNPs per kg BW. The Rpl19 was used as internal control. The values are expressed as the mean ± S.E.M. (ANOVA followed by the post-test of Dunnett), *P < 0.05 and **P < 0.01 vs. control, n = 12 per group. Gper: estrogen receptor 1 coupled to protein G; Esr1: estradiol receptor 1; Esr2: estradiol receptor 2; Rpl19: ribosomal protein L19; AgNPs: silver nanoparticles; BW: body weight; SEM: standard error of the mean. | |
Effects of AgNPs on the sperm production, sperm reserves and sperm transit time
With regard to sperm parameters, none of the AgNP dosages, used in this study, had an effect on sperm production (Table 2). However, the sperm reserves (Fig. 10A) and the sperm transit time (Fig. 10C) through the caput epididymis were decreased in the 1.875 μg AgNP per kg-treated group, when compared to controls. On the other hand, the sperm reserves (Fig. 10B) and the sperm transit time (Fig. 10D) in the cauda epididymis were not affected by AgNP treatment.
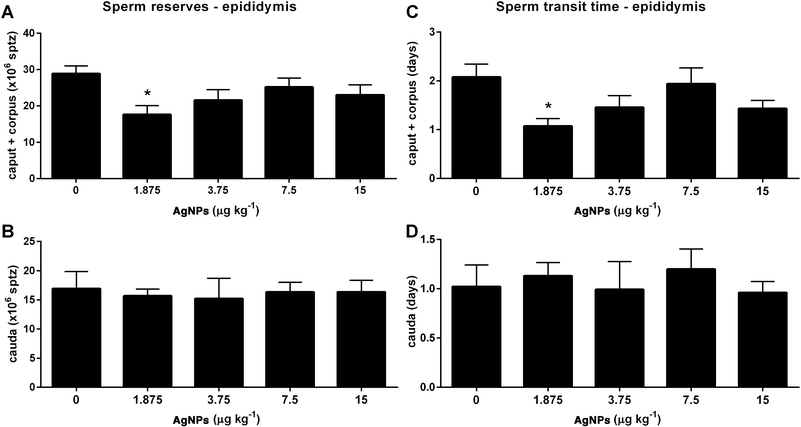 |
| Fig. 10 Sperm reserves (A and B) and sperm transit time (C and D) in epididymis segments for rats exposed to 0 (control), 15, 7.5, 3.75 or 1.875 μg of AgNPs per kg BW. The values are expressed as the mean ± SEM; ANOVA followed by the post-test of Dunnett; *P < 0.05 vs. control, n = 12 per group. AgNPs: silver nanoparticles; BW: body weight; SEM: standard error of the mean. | |
Table 2 Sperm production in animals exposed to silver nanoparticles (AgNPs)
Sperm production |
AgNPs (μg kg−1) |
0 |
1.875 |
3.75 |
7.5 |
15 |
Data were subjected to analysis of variance and are expressed as the mean ± standard error of the mean; n = 12 animals per group. |
Total
|
×106 per testis |
97.1 ± 3.5 |
98.3 ± 8.5 |
92.7 ± 8.3 |
98.1 ± 10.0 |
99.4 ± 7.2 |
×106 per g testis |
69.0 ± 2.9 |
70.4 ± 6.0 |
64.8 ± 5.9 |
69.8 ± 7.5 |
65.6 ± 5.4 |
Daily
|
×106 per testis |
15.9 ± 0.5 |
16.1 ± 1.4 |
15.2 ± 1.3 |
16.1 ± 1.6 |
16.3 ± 1.1 |
×106 per g testis |
11.3 ± 0.4 |
11.5 ± 0.9 |
10.6 ± 0.9 |
11.4 ± 1.2 |
10.8 ± 0.8 |
Effect of AgNPs on the sperm functionality
Finally, we examined sperm functionality parameters, which may impact the ability of the sperm to fertilize the oocyte. The acrosome integrity was reduced in the 15 μg AgNP per kg-treated group, when compared to controls (Fig. 11A); however, the plasma membrane integrity and the sperm morphology were unchanged in all of the AgNP dosages utilized in this study (Fig. 11B and C). The mitochondrial activity was reduced in the 7.5 and 15 μg AgNP per kg-treated groups, as compared to controls (Fig. 11D).
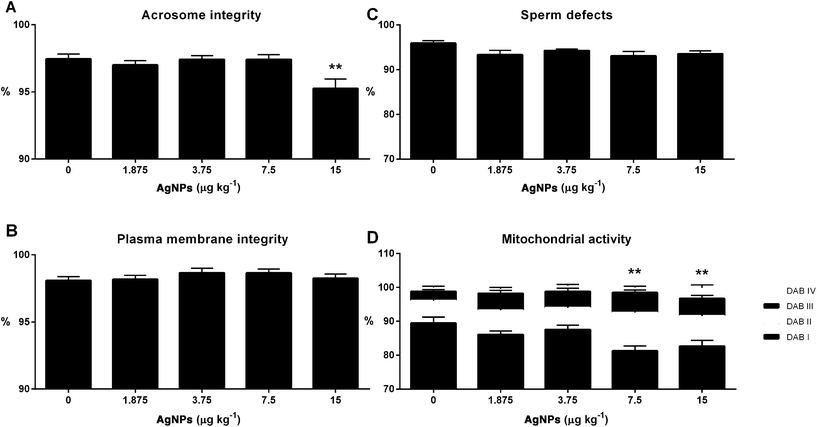 |
| Fig. 11 Frequency of acrosome integrity (A), plasma membrane integrity (B), defects (C) and mitochondrial activity (D) of the sperm for rats exposed to 0 (control), 15, 7.5, 3.75 or 1.875 μg of AgNPs per kg BW. The values are expressed as the mean ± SEM; ANOVA followed by the post-test of Dunnett; *P < 0.05 vs. control, n = 12 per group. AgNPs: silver nanoparticles; BW: body weight; SEM: standard error of the mean. | |
Discussion
Reproductive physiology involves complex biological processes that may be sensitive to environmental chemical contaminants, which may increase the incidence of male reproductive disorders.45 In the present study, we investigated the molecular mechanisms involved in the dose–response effects of four dosages of AgNPs (1.875, 3.75, 7.5 or 15 μg AgNPs per kg) on the regulation of the HPT axis and the testicular function using prepubertal male Wistar rats as an experimental model.
The mean size of silver nanoparticles estimated by dynamic light scattering was 79.5 nm, possibly due to differences in the evaluation method (the manufacturer used transmission electron microscopy7,46). In the simulated gastric fluid, the mean particle size of AgNPs increased with time in the low pH environment of the stomach. These data are similar to those reported by Pinďáková et al. (2017).47 In the simulated intestinal fluid, the AgNPs did not present this behavior, remaining dispersed in the solution.47 After oral exposure, the liver, the kidney and the blood present higher levels of AgNPs, which consistently decline in the subsequent weeks.48 Nevertheless, in the testis and brain the levels are still elevated.48–50 This may contribute to the development of the toxic effects observed in this study.
The central nervous system is the main initiator of puberty in rats. A network not yet fully understood controls the GnRH neurons, in which before puberty their activity is predominantly inhibitory and after puberty predominantly excitatory.51 Prior to puberty, a pulsatile release of GnRH is increased and stimulatory events lead to the release of LH and FSH from pituitary, which will perform their action in the testicular cells.51–53 The action of FSH in the Sertoli cells helps to coordinate the onset of puberty and progression, but the androgens play a more relevant role in the process.52 The balanopreputial separation is a good indicator of the progress of puberty in rats because there is a positive correlation between the rise in the serum concentrations of testosterone at the beginning of puberty and the externalization of the penis from the glans.54 Exposure to chemicals with estrogenic or antiandrogenic effects can delay the onset of puberty in males.55,56 The delayed puberty in the rats, treated with 7.5 and 15 μg AgNPs per kg, observed in this study suggests that hormonal imbalances occurred during the prepubertal period. In addition to these events, peripheral insulin growth factor 1 (IGF-1) stimulates GnRH secretion, which correlates positively with body growth and the progress of puberty.51 In this study, the body development was not affected by AgNP oral exposure during the prepubertal period. In fact, this parameter is affected by oral exposure to AgNPs only at higher dosages (125 mg kg−1) and after longer periods of treatment in male rats.57
After puberty, the hypothalamic neurons release GnRH in a pulse frequency that stimulates the expression of Lhb and Fshb and the secretion of LH and FSH by the gonadotrophs in the pituitary.36,37 The regulation of GnRH-producing neurons is still under investigation,58 but is suggestive that estradiol receptors mediate their activity.59 Estradiol binds to ESR2 activating the transcriptional complex, which promotes the transcription of the GnRH gene,60 while ESR1 activation is the predominant mechanism involved in the estradiol-mediated suppression of GnRH synthesis/release.61 In this study, the transcript expression of Gnrh1 is increased (3.75 μg AgNPs per kg) and the transcript expression of Esr2 is reduced (1.875, 3.75 and 7.5 μg AgNPs per kg), which could suggest that there is a disruption in estradiol signaling in the hypothalamus. Furthermore, AgNP treatment (7.5 μg AgNPs per kg) also reduced the transcript expression of Esr1 in the hypothalamus.
The transcript expressions of gonadotropins (Lhb and Fshb) were not affected by treatment, but the serum concentrations of LH and FSH were increased (3.75 and 1.875 μg AgNPs per kg, respectively). This increase may be related to mechanisms involved in the posttranscriptional regulation, such as the half-life of mRNA in the cytoplasm, the translational rate of mRNA,62 or through altered gonadotropin secretion.63
The negative feedback mechanism in the hypothalamus and pituitary is mediated by testosterone binding to the AR, which represses the synthesis and release of LH, while the negative feedback of FSH is mediated, in the pituitary, by the action of inhibin b, which represses its synthesis and release.39 The transcript expression of Ar is reduced in the pituitary of rats (1.875, 3.75 and 7.5 μg AgNPs per kg), weakening the negative feedback mechanism controlling the synthesis and secretion of LH. The transcript expression of Inhbb is only elevated in the 7.5 μg AgNPs per kg group. These molecular mechanisms are unclear, but the disruption in the LH synthesis and secretion may perturb the fine regulation of FSH, since it is produced by the same pituitary cells.
In the testis, LH stimulates the synthesis of testosterone by the Leydig cells.40 The exposure to AgNPs increased the serum concentrations of testosterone (1.875 μg AgNPs per kg). In previous studies using higher doses (15, 30 or 50 μg AgNPs per kg) this parameter was not affected.21,22
Testosterone binds to the AR in the Sertoli cells40 and plays a key role in the maintenance of spermatogenesis, participating in the maintenance of the blood–testis barrier, as well as the adhesion and release of sperm from the Sertoli cells.64,65 The transcript expression of Ar was reduced (1.875 and 3.75 μg AgNPs per kg).
Normal sexual development and reproduction in mammals depends on the balance between androgens and estrogens. In the testis, maintaining this balance relies on endocrine and paracrine factors, but it is also related to the activity of aromatase, an enzyme complex located in the endoplasmic reticulum that ensures the conversion of androgen into estrogen.66 Testicular estradiol is produced in the testis from the aromatization of testosterone by the aromatase cytochrome P450 family 19 subfamily A member 1 enzyme, encoded by the gene CYP19A1.67 In the present study, serum estradiol concentrations were increased (7.5 μg AgNPs per kg), whereas Cyp19a1 expression was not altered in any treated group. Interestingly, the estradiol serum concentrations and aromatase expression were not affected in the 1.875 μg AgNP per kg-treated group (increased testosterone), which may indicate a protective mechanism avoiding higher and more deleterious estradiol levels.68
The estradiol interacts with estrogen receptors located in the nucleus and cytoplasm (ESR1 and ESR2) or in the plasma membrane (GPER), which is crucial for the regulation of proteins involved in the function of the testis.41 In fact, ESR1 and ESR2 knockout mice exhibit compromised spermatogenesis.69 Furthermore, ESR1 and ESR2 were found in the testis of adult and immature rats and were also expressed in co-culture of Sertoli cells obtained from mice at 15 days of age.70 Additionally, GPER has been detected in the extranuclear regions of Sertoli cells and can regulate the expression of genes involved in cell apoptosis.71 It has also been shown that ESR1 and ESR2 are involved in the control of cell proliferation and differentiation by modulating enhancers and inhibitors of the cell cycle.72 Additionally, ESR1 is necessary for the viability of germ cells and is involved in chromatin condensation and DNA integrity of spermatozoa.73 In this study, the expression of the transcripts of Gper was decreased in the group receiving the lowest dosage (1.875 μg AgNPs per kg), whereas the transcripts of Esr1 were decreased in the groups treated with 1.875 and 3.75 μg AgNPs per kg. As a consequence of these alterations a reduction in the sperm production could occur, but it was surprisingly not affected. A possible explanation is that the spermatic cycle could be compromised, because Sleiman et al.22 only observed a reduction in the sperm production thirty-seven days after AgNP exposure. In this sense, the compromised spermatogenesis observed in these groups exposed to lower doses of AgNPs should not be disregarded.
Furthermore, the sperm reserves and the sperm transit time in caput and corpus epididymis were reduced in the 1.875 μg AgNP per kg-treated group. During the transit through the epididymis segments, the sperm released from the lumen of seminiferous tubules undergoes maturation and acquires motility and the ability to fertilize oocytes.74 The sperm transit time through the epididymis segments is due to the movement of the fluid secreted by the Sertoli cells, which results from the contractile activity of the smooth muscle epididymis, which is controlled by androgen action and the autonomic nervous system.75 In this study, the reduced sperm reserves and the sperm transit time may be due to increased serum concentrations of testosterone which could stimulate the contraction of the smooth muscle of the epididymis; as a result these increased contractions could contribute to the observed decrease in sperm transit time.
Next, the sperm collected from the cauda epididymis was subjected to the functional analysis of acrosome integrity, plasma membrane integrity, mitochondrial activity and sperm morphology. In this study, the sperm morphology and the plasma membrane integrity were not affected. The acrosome integrity was reduced (15 μg AgNPs per kg) and the mitochondrial activity was reduced (7.5 and 15 μg AgNPs per kg). The acrosome plays a fundamental role in the fertilization process and the reduction in its integrity may compromise the ability to fertilize.76 Mitochondria are located in the middle piece of the sperm, which produces energy to move the flagellum and propel the spermatozoa through the genital tract. In this way, a reduction in its function may compromise sperm mobility and consequently hinder fertilization.77 Alterations in the group receiving the higher dose (15 μg AgNPs per kg) were previously demonstrated by our group,21 and this was included in this study to represent the highest dosage in the dose–response curve. Thus, it was possible to infer a LOAEL (Lowest Observed Adverse Effect Level) of 7.5 μg AgNPs per kg and a NOAEL (No Observed Adverse Effect Level) of 3.75 μg AgNPs per kg for spermatic function.
Taking all these results together, some differences were observed among the AgNP-treated groups. The groups receiving 1.875 and 3.75 μg AgNPs per kg did not present any alteration in the functional parameters analyzed, but only in the analysis at the molecular level. The group receiving 1.875 μg AgNPs per kg was the only group to display increased serum concentrations of FSH and testosterone. The group receiving 3.75 μg AgNPs per kg was the only group to present increased transcript expression of Gnrh1 and increased LH serum concentrations. The group receiving 7.5 μg AgNPs per kg had delayed puberty onset, reduced mitochondrial activity, decreased hypothalamic expression of Esr1, increased expression of Inhbb and elevated serum concentrations of estradiol. The group receiving 15 μg AgNPs per kg presented delayed puberty onset and decreased sperm functionality, yet all of the other parameters were unaffected. These differences may be related to the levels of dose–response in the HPT axis, and help in understanding the meaning of the modulation of the studied genes and the functional parameters. At lower doses (1.875 and 3.75 μg AgNPs per kg), the modulation of the genes in the HPT axis avoid disturbances in its function. At higher dose (15 μg AgNPs per kg), the genes are not being modulated but the function is compromised.
Finally, humans may be exposed to AgNPs by everyday consumer products which are nano-functionalized13 and for which the safety limits of exposure are still under evaluation.16 It is known that AgNP-impregnated toothbrushes release Ag, which may cause human and environmental exposure to AgNps.14 Quadros et al.15 tested the amount of AgNPs leached by infant products (plush toys, baby blankets, and sippy cups), and found that 43.8% of the AgNPs leached from a sippy cup (spout cover), representing 0.93 ± 0.02 mg kg−1 product per day.15 The same study was also evaluated by probabilistic methods to assess the relative uncertainty and potential risks of exposure of infants to AgNPs and it was concluded that the leach from these infant products exhibited a minimal risk.78 The relevance of human exposure to low doses of AgNPs is a controversial issue and in this sense it is necessary to perform more risk assessment studies.
Experimental
Materials and methods
Characterization of AgNPs.
A suspension of silver nanoparticles 60 nm in diameter was purchased from Sigma-Aldrich (catalog number 730815, Sigma-Aldrich Co., Seelze, Germany). Dilution with water was performed just before the suspension was administered to the animals according to our previous study.21 To evaluate whether diluting the AgNP solution affected the nanoparticle stability, we analyzed the mean particle size and polydispersity index by dynamic light scattering (DSL) (BIC 90 plus – Brookhaven Instruments Corp., USA) at a scattering angle of 90° and a temperature of 25 °C, with the help of ZetaPlus Particle Sizing Software Version 5.34 (Brookhaven Instruments Corp., USA). The particle size of AgNPs was evaluated in simulated gastric fluid. For this, AgNPs were diluted in ultra-purified water or acidic conditions (simulated gastric fluid, SGF, without pepsin, pH 1.2; USP 201279) to a final concentration of 0.004 mg mL−1 and kept in a platform shaker at 200 rpm and 37 °C. The samples were taken after 0, 30, 60 and 120 minutes and analyzed by DLS under the same conditions as those described above.
Experimental design.
The experimental design was based on a protocol suggested by Stoker et al.56 from the Endocrine Disrupting Screening and Testing Advisory Committee (EDSTAC) and includes the evaluation of endocrine-disrupting chemical effects in prepuberty and puberty. Sixty newly weaned male Wistar rats (Rattus norvegicus var. albinus) were collected from pregnant female rats that had been monitored from the 17th day of pregnancy to determine the exact day of birth. On postnatal day 4 (PND4), 10 litters were culled to 8 pups (4 males/4 females) per female and kept at this size until weaning (PND21). On PND23, the male offspring were divided into five groups containing 12 animals each, and received either 0 (control), 1.875, 3.75, 7.5 or 15 μg of AgNPs per kg of body weight (BW) from PND23 to PND60. The highest dose of AgNPs for the dose–response curve used in this study was based on the experimental toxicological values for the lowest observable adverse effect level (LOAEL) for sperm parameters (15 μg kg−1),21 and corresponds to a dose that is 2000-fold lower than the NOAEL for liver toxicity.17,57 The AgNP water suspension was administered every other day at a volume of 0.25 ml per 100 g BW by gavage. The control group received only water. During all experiments, the animals were kept in groups of four, housed in polypropylene cages (43 × 43 × 20 cm) with a 5 cm layer of wood shavings, fed commercial feed (Nuvilab CR-1, Nuvital, PR, Brazil) and were allowed access to water ad libitum, under a 12
:
12 hour dark/light cycle in a temperature-controlled room (23 ± 1 °C). All procedures were performed in accordance with the Conselho Nacional de Controle de Experimentação Animal (CONCEA) and were approved by the Universidade Estadual do Centro-Oeste Ethical Committee for Animal Research (protocol 013/2015).
Puberty and body growth.
An evaluation of the separation of the preputial membrane and the externalization of the glands of the penis (balanopreputial separation, PPS) was used to determine the age at the onset of puberty.54 This evaluation was performed on PND33 and was continued every other day throughout the balanopreputial separation period by gentle tissue manipulation. The animals were weighed every other day from PND23 to PND60 to determine body growth.
Tissue collection.
At PND60, animals were euthanized by decapitation under deep anesthesia with ketamine and xylazine. Blood was collected by cardiac puncture, immediately centrifuged at 205g (II Excelsa BL 206, Sao Paulo, SP, Brazil) for 15 min, and the serum was maintained at −80 °C until further analysis of hormonal dosages of LH, FSH, testosterone and estradiol. The hypothalamus, the pituitary and testes were immediately excised, frozen in liquid nitrogen and kept at −80 °C until further analysis of gene expression by RT-qPCR. The testes, epididymis (caput, corpus and cauda), seminal vesicles and seminal fluid were weighed and converted to mg of tissue per 100 mg of body weight.
Assessment of sperm functionality.
The sperm was collected from the cauda epididymis by making an incision with a scalpel blade and squeezing out the fluid. Twenty microliters of the drained fluid containing spermatozoa were suspended in 200 μl of a seminal extender (328.8 mM Tris, 91.3 mM fructose, and 115.8 mM citric acid) at 37 °C. The samples were then immediately subjected to functional analysis of the acrosome integrity, plasma membrane integrity, mitochondrial activity and sperm morphology, as previously described.21,80
The acrosome integrity was evaluated by using a single-stain solution containing 1% (w/v) rose bengal (cat. number 330000, Sigma-Aldrich Co, MO, USA), 1% (w/v) fast green FCF (cat. number F7252, Sigma-Aldrich Co, MO, USA), and 40% ethanol in 200 mM disodium phosphate buffer containing 100 mM citric acid at pH 7.2. Five microliters of each sample were incubated with 5 μl of the single-stain solution for 1 min at 37 °C. The total volume (10 μl) was then pipetted onto a slide and smeared with another slide, air-dried at 37 °C and analyzed by light microscopy (1000×). Two hundred spermatozoa were counted per slide and were classified as having intact or damaged acrosomes.
The plasma membrane integrity was evaluated using eosin–nigrosin stain [1% eosin Y (Sigma-Aldrich, MO, USA) and 10% nigrosin (Sigma-Aldrich, MO, USA) in distilled water]. Five microliters of each sample were mixed with 5 μl of the stain, and the total volume was pipetted onto a slide, smeared with another slide, and air-dried at room temperature. Two hundred spermatozoa were counted per slide by light microscopy (1000×) and were classified as having intact or damaged membranes.
The mitochondrial activity was evaluated by measuring the enzyme activity of cytochrome c oxidase in the intermembrane space of the spermatozoa using DAB (3,3′-diaminobenzidine, Sigma-Aldrich, MO, USA). DAB was diluted in PBS (2.7 mM KCl, 137 mM NaCl, 8 mM NaHPO4, and 1.4 mM KPO4, pH 7.4) to a final concentration of 1 mg ml−1 and frozen until use. Ten microliters of each sample were incubated with 300 μl of a DAB solution pre-heated to 37 °C for 1 h at 37 °C in the dark. Ten microliters of each sample were pipetted onto a slide, smeared with another slide, and air-dried at 37 °C in the dark. The slide samples were then incubated for 10 min in a fixative solution containing 10% formaldehyde (F8775, Sigma-Aldrich Co, MO, USA) in PBS and allowed to air-dry at 37 °C in the dark. Two hundred spermatozoa from each sample were analyzed by phase-contrast microscopy at a magnification of 400× and then classified based on the degree of staining of the intermediate piece as follows: DAB I (completely stained), DAB II (>50%), DAB III (<50%) and DAB IV (unstained).
The sperm morphology was evaluated as a wet preparation by phase contrast microscopy (400× magnification) in diluted samples in buffered formalin–saline solution (34.72 mM Na2HPO4·2H2O, 18.68 mM KH2PO4, 92.4 mM NaCl, and 12.5% (v/v) formaldehyde).
Sperm production, sperm reserves and sperm transit time.
The sperm production, reserves and transit time were determined as previously detailed by Romano et al.,81 and according to the method described by Robb et al.82 Briefly, the testes were weighed, the tunica albuginea was removed and the testicular parenchyma was homogenized in 5 ml of a saline solution containing 0.5% Triton X-100, followed by sonication at 12 kHz for 30 seconds (Ultrasonic homogenizer DES500; Unique, Brazil), and the resistant spermatids were counted using a hemocytometer. Daily sperm production was obtained by dividing the sperm production by 6.1 [spermatids counted in this method represent 48% of one cycle of the seminiferous epithelium which has a duration of 12.75 days for rats (12.75 × 0.48 = 6.1)]. Sperm reserves were calculated from epididymis segments (caput, corpus and cauda) that were weighed and minced, homogenized in 5 mL of 0.9% NaCl containing 0.5% Triton X-100 followed by sonication at 12 kHz for 30 seconds (Ultrasonic homogenizer DES500; Unique, Brazil) and the spermatozoa were counted using a hemocytometer. Sperm transit time was calculated by dividing the number of spermatozoa in each segment by the daily sperm production of the corresponding testis.
Reverse transcription followed by real-time quantitative PCR (RT-qPCR).
The hypothalamus, the pituitary and testes were pulverized in liquid nitrogen and total RNA was extracted by the guanidine–phenol–chloroform method,83 using Trizol reagent (Life Technologies, Carlsbad, USA) according to the manufacturer's instructions. The hypothalamus was evaluated for the transcript expression of Gnrh1, Ar, Esr1 and Esr2 relative to the expression of the ribosomal protein L19 (Rpl19). The pituitary was evaluated for the transcript expression of Gnrhr, Lhb, Fshb, Ar, Esr1 and Esr2 relative to the expression of Rpl19. The testes were evaluated for the transcript expression of Lhcgr, Fshr, Inhbb, Ar, Esr1, Esr2, Gper and Cyp19a1 relative to the expression of Rpl19. Two and a half micrograms of total RNA were reverse transcribed using oligo(dT) primer with the help of a GoScript Reverse Transcription System kit (Promega, Madison, USA) according to the manufacturer's instructions. Real-time PCR was carried out with an Applied Biosystems StepOnePlus Real-time PCR System (Applied Biosystems, Singapore) using a SYBR Green qPCR Platinum SuperMix-UDG with the ROX (Life Technologies, Carlsbad, USA) kit under the following cycling conditions: 50 °C (2 min), 95 °C (2 min) and 40 cycles of 95 °C (15 s) and 60 °C (30 s). At the end of the reaction, a melting curve was generated and analyzed to confirm the specificity of the amplification. The average cycle threshold (Ct) was automatically determined using StepOne Software v2.3 (Applied Biosystems), and quantification was performed using the 2−ΔΔCt method, as previously described.84 The primer pairs are shown in ESI Table 2.†
Hormone measurements.
Estradiol and testosterone serum concentrations were analyzed by colorimetric competitive enzyme immunoassay using a commercial Testosterone ELISA kit and a 17β-estradiol ELISA kit (Enzo Life Sciences Inc., Farmingdale, NY, USA). The serum LH and FSH concentrations were determined by a chemiluminescent immunoassay using Luminex xMAP technology (Milliplex MAP rat pituitary panel; Billerica, MA, USA). The intra-assay coefficients of variation were <10% for testosterone, <8% for estradiol, <6% for LH and <4.5% for FSH.
Statistical analysis.
The variables were first subjected to the Kolmogorov–Smirnov test for normality and the Bartlett test for homoscedasticity. The analysis of body growth was performed by multi-way analysis of variance for repeated measures (MANOVA) using a general linear model (GLM). The day of PPS was compared among the groups using nonparametric analyses with the Kruskal–Wallis method followed by the post hoc Dunn test. All other parameters were analyzed by ANOVA followed by the post-test of Dunnett, comparing treated groups only with the control group. Serum concentrations of estradiol and the Cyp19a1 relative expression were compared by Pearson's correlation. All analyses were performed with Statistica 7.0 (StatSoft Inc., Tulsa, OK). Statistical differences were considered significant when P was less than 0.05.
Conclusions
In this study, it was observed that AgNPs modulate the expression of genes related to the control of the HPT axis and spermatogenesis with repercussions in the production of serum testosterone and estradiol concentrations only in the group receiving the lowest dosage of AgNPs, while functional alterations of the sperm were observed in the group receiving the higher dosages. This is suggestive of a disruption of the HTP axis by AgNPs during the prepubertal and pubertal periods, which are the most susceptible windows for the endocrine-disrupting chemical activity.
Conflicts of interest
There are no conflicts to declare.
Acknowledgements
This study was supported by the Coordenação de Aperfeiçoamento de Pessoal de Nivel Superior, Brazil [23038.009865/2013-32] and Fundacao Araucaria, Brazil [516/2014].
Notes and references
- A. Ingle, A. Gade, S. Pierrat, C. Sonnichsen and M. Rai, Mycosynthesis of silver nanoparticles using the fungus Fusarium acuminatum and its activity against some human pathogenic bacteria, Curr. Nanosci., 2008, 4, 141–144 CrossRef CAS.
- J. S. Kim, E. Kuk, K. N. Yu, J. H. Kim, S. J. Park, H. J. Lee, S. H. Kim, Y. K. Park, Y. H. Park, C. Y. Hwang, Y. K. Kim, Y. S. Lee, D. H. Jeong and M. H. Cho, Antimicrobial effects of silver nanoparticles, Nanomedicine, 2007, 3, 95–101 CrossRef CAS PubMed.
- J. Chen, C. M. Han, X. W. Lin, Z. J. Tang and S. J. Su, [Effect of silver nanoparticle dressing on second degree burn wound], Zhonghua waike zazhi, 2006, 44, 50–52 Search PubMed.
- M. S. Cohen, J. M. Stern, A. J. Vanni, R. S. Kelley, E. Baumgart, D. Field, J. A. Libertino and I. C. Summerhayes, In vitro analysis of a nanocrystalline silver-coated surgical mesh, Surg. Infect., 2007, 8, 397–403 CrossRef PubMed.
- A. B. Lansdown, Silver in health care: antimicrobial effects and safety in use, Curr. Probl. Dermatol., 2006, 33, 17–34 CAS.
- H. Y. Lee, H. K. Park, Y. M. Lee, K. Kim and S. B. Park, A practical procedure for producing silver nanocoated fabric and its antibacterial evaluation for biomedical applications, Chem. Commun., 2007, 2959–2961, 10.1039/b703034g.
- A. Dhawan and V. Sharma, Toxicity assessment of nanomaterials: methods and challenges, Anal. Bioanal. Chem., 2010, 398, 589–605 CrossRef CAS PubMed.
- N. Durán, P. D. Marcato, R. D. Conti, O. L. Alves, F. T. M. Costa and M. Brocchi, Potential use of silver nanoparticles on pathogenic bacteria, their toxicity and possible mechanisms of action, J. Braz. Chem. Soc., 2010, 21, 949–959 CrossRef.
- R. Garcia-Contreras, L. Argueta-Figueroa, C. Mejia-Rubalcava, R. Jimenez-Martinez, S. Cuevas-Guajardo, P. A. Sanchez-Reyna and H. Mendieta-Zeron, Perspectives for the use of silver nanoparticles in dental practice, Int. Dent. J., 2011, 61, 297–301 CrossRef PubMed.
- M. Horie, H. Kato, K. Fujita, S. Endoh and H. Iwahashi, In vitro evaluation of cellular response induced by manufactured nanoparticles, Chem. Res. Toxicol., 2012, 25, 605–619 CrossRef CAS PubMed.
- N. R. Savithramma, M. L. Rao, K. Rukmini and P. S. Devi, Antimicrobial activity of silver nanoparticles synthesized by using medicinal plants, Int. J. ChemTech Res., 2011, 3, 9 Search PubMed.
-
W. W. I. C. f. S. WWICS, Project on Emerging Nanotechnologies: Nanotechnology, http://www.nanotechproject.org/, (accessed 09/06/2016, 2016).
- E. McGillicuddy, I. Murray, S. Kavanagh, L. Morrison, A. Fogarty, M. Cormican, P. Dockery, M. Prendergast, N. Rowan and D. Morris, Silver nanoparticles in the environment: Sources, detection and ecotoxicology, Sci. Total Environ., 2017, 575, 231–246 CrossRef CAS PubMed.
- A. Mackevica, M. E. Olsson and S. F. Hansen, The release of silver nanoparticles from commercial toothbrushes, J. Hazard. Mater., 2017, 322(Part A), 270–275 CrossRef CAS PubMed.
- M. E. Quadros, R. Pierson, N. S. Tulve, R. Willis, K. Rogers, T. A. Thomas and L. C. Marr, Release of Silver from Nanotechnology-Based Consumer Products for Children, Environ. Sci. Technol., 2013, 47, 8894–8901 CrossRef CAS PubMed.
-
OECD, Series on the Safety of Manufactured Nanomaterials, 74, Exposure assessment of nano-silver (AgNP): case study, 2016, pp. 36 Search PubMed.
-
S. C. o. E. a. N. I. H. R. SCENIHR, Nanosilver: safety, health and environmental effects and role in antimicrobial resistance, 2014, pp. 103 Search PubMed.
- R. M. Silva, D. S. Anderson, J. Peake, P. C. Edwards, E. S. Patchin, T. Guo, T. Gordon, L. C. Chen, X. Sun, L. S. Van Winkle and K. E. Pinkerton, Aerosolized Silver Nanoparticles in the Rat Lung and Pulmonary Responses over Time, Toxicol. Pathol., 2016, 44, 673–686 CrossRef CAS PubMed.
- M. Almansour, Q. Jarrar, A. Battah and B. Jarrar, Morphometric Alterations Induced by the Toxicity of Variable Sizes of Silver Nanoparticles, Int. J. Morphol., 2015, 33, 544–552 CrossRef.
- E. J. Park, J. Yi, Y. Kim, K. Choi and K. Park, Silver nanoparticles induce cytotoxicity by a Trojan-horse type mechanism, Toxicol. in Vitro, 2010, 24, 872–878 CrossRef CAS PubMed.
- F. T. Mathias, R. M. Romano, M. M. Kizys, T. Kasamatsu, G. Giannocco, M. I. Chiamolera, M. R. Dias-da-Silva and M. A. Romano, Daily exposure to silver nanoparticles during prepubertal development decreases adult sperm and reproductive parameters, Nanotoxicology, 2015, 9, 64–70 CrossRef CAS PubMed.
- H. K. Sleiman, R. M. Romano, C. A. Oliveira and M. A. Romano, Effects of prepubertal exposure to silver nanoparticles on reproductive parameters in adult male Wistar rats, J. Toxicol. Environ. Health, Part A, 2013, 76, 1023–1032 CrossRef CAS PubMed.
- S. A. Bidgoli, M. Mahdavi, S. M. Rezayat, M. Korani, A. Amani and P. Ziarati, Toxicity assessment of nanosilver wound dressing in Wistar rat, Acta Med. Iran., 2013, 51, 203–208 Search PubMed.
- M. Korani, S. M. Rezayat and S. Arbabi Bidgoli, Sub-chronic Dermal Toxicity of Silver Nanoparticles in Guinea Pig: Special Emphasis to Heart, Bone and Kidney Toxicities, Iran. J. Pharm. Res., 2013, 12, 511–519 CAS.
- C. Recordati, M. De Maglie, S. Bianchessi, S. Argentiere, C. Cella, S. Mattiello, F. Cubadda, F. Aureli, M. D'Amato, A. Raggi, C. Lenardi, P. Milani and E. Scanziani, Tissue distribution and acute toxicity of silver after single intravenous administration in mice: nano-specific and size-dependent effects, Part. Fibre Toxicol., 2016, 13, 12 CrossRef PubMed.
- H. Guo, J. Zhang, M. Boudreau, J. Meng, J. J. Yin, J. Liu and H. Xu, Intravenous administration of silver nanoparticles causes organ toxicity through intracellular ROS-related loss of inter-endothelial junction, Part. Fibre Toxicol., 2016, 13, 21 CrossRef PubMed.
- C. Carlson, S. M. Hussain, A. M. Schrand, L. K. Braydich-Stolle, K. L. Hess, R. L. Jones and J. J. Schlager, Unique cellular interaction of silver nanoparticles: size-dependent generation of reactive oxygen species, J. Phys. Chem. B, 2008, 112, 13608–13619 CrossRef CAS PubMed.
- N. Hadrup, K. Loeschner, A. Mortensen, A. K. Sharma, K. Qvortrup, E. H. Larsen and H. R. Lam, The similar neurotoxic effects of nanoparticulate and ionic silver in vivo and in vitro, Neurotoxicology, 2012, 33, 416–423 CrossRef CAS PubMed.
- N. Asare, C. Instanes, W. J. Sandberg, M. Refsnes, P. Schwarze, M. Kruszewski and G. Brunborg, Cytotoxic and genotoxic effects of silver nanoparticles in testicular cells, Toxicology, 2012, 291, 65–72 CrossRef CAS PubMed.
- P. V. AshaRani, G. Low Kah Mun, M. P. Hande and S. Valiyaveettil, Cytotoxicity and Genotoxicity of Silver Nanoparticles in Human Cells, ACS Nano, 2009, 3, 279–290 CrossRef CAS PubMed.
- L. Xu, A. Shao, Y. Zhao, Z. Wang, C. Zhang, Y. Sun, J. Deng and L. L. Chou, Neurotoxicity of Silver Nanoparticles in Rat Brain After Intragastric Exposure, J. Nanosci. Nanotechnol., 2015, 15, 4215–4223 CrossRef CAS PubMed.
- T. T. Win-Shwe and H. Fujimaki, Nanoparticles and neurotoxicity, Int. J. Mol. Sci., 2011, 12, 6267–6280 CrossRef CAS PubMed.
- L. Braydich-Stolle, S. Hussain, J. J. Schlager and M. C. Hofmann, In vitro cytotoxicity of nanoparticles in mammalian germline stem cells, Toxicol. Sci., 2005, 88, 412–419 CrossRef CAS PubMed.
- L. K. Braydich-Stolle, B. Lucas, A. Schrand, R. C. Murdock, T. Lee, J. J. Schlager, S. M. Hussain and M. C. Hofmann, Silver nanoparticles disrupt GDNF/Fyn kinase signaling in spermatogonial stem cells, Toxicol. Sci., 2010, 116, 577–589 CrossRef CAS PubMed.
- C. Castellini, S. Ruggeri, S. Mattioli, G. Bernardini, L. Macchioni, E. Moretti and G. Collodel, Long-term effects of silver nanoparticles on reproductive activity of rabbit buck, Syst. Biol. Reprod. Med., 2014, 60, 143–150 CrossRef CAS PubMed.
- U. B. Kaiser, A. Jakubowiak, A. Steinberger and W. W. Chin, Differential Effects of Gonadotropin-Releasing Hormone (GnRH) Pulse Frequency on Gonadotropin Subunit and GnRH Receptor Messenger Ribonucleic Acid Levels in Vitro 1, Endocrinology, 1997, 138, 1224–1231 CrossRef CAS PubMed.
- L. Nagirnaja, K. Rull, L. Uusküla, P. Hallast, M. Grigorova and M. Laan, Genomics and genetics of gonadotropin beta-subunit genes: unique FSHB and duplicated LHB/CGB loci, Mol. Cell. Endocrinol., 2010, 329, 4–16 CrossRef CAS PubMed.
-
K.-H. Jeong and U. B. Kaiser, in Knobil and Neill's Physiology of Reproduction, ed. J. D. Neill, T. M. Plant, D. W. Pfaff, J. R. G. Challis, D. M. de Kretser, J. S. Richards and P. M. Wassarman, Academic Press, St Louis, 3rd edn, 2006, vol. 1, pp. 1635–1701 Search PubMed.
- A. E. O'Connor and D. M. De Kretser, Inhibins in normal male physiology, Semin. Reprod. Med., 2004, 22, 177–185 CrossRef PubMed.
-
L. O'Donnell, S. J. Meachem, P. G. Stanton and R. I. McLachlan, in Knobil and Neill's Physiology of Reproduction, ed. J. D. Neill, T. M. Plant, D. W. Pfaff, J. R. G. Challis, D. M. de Kretser, J. S. Richards and P. M. Wassarman, Academic Press, St Louis, 3rd edn, 2006, vol. 1, pp. 1017–1069 Search PubMed.
- A. Chimento, R. Sirianni, I. Casaburi and V. Pezzi, Role of estrogen receptors and g protein-coupled estrogen receptor in regulation of hypothalamus-pituitary-testis axis and spermatogenesis, Front. Endocrinol., 2014, 5, 1 Search PubMed.
- R. J. Aitken, Sperm function tests and fertility, Int. J. Androl., 2006, 29, 69–75 CrossRef CAS PubMed ; discussion 105–108.
- H. Rodriguez-Martinez, Laboratory semen assessment and prediction of fertility: still utopia?, Reprod. Domest. Anim., 2003, 38, 312–318 CrossRef CAS PubMed.
- T. Rijsselaere, A. Van Soom, S. Tanghe, M. Coryn, D. Maes and A. de Kruif, New techniques for the assessment of canine semen quality: a review, Theriogenology, 2005, 64, 706–719 CrossRef CAS PubMed.
- B. Lucas, C. Fields and M. C. Hofmann, Signaling pathways in spermatogonial stem cells and their disruption by toxicants, Birth Defects Res., Part C, 2009, 87, 35–42 CrossRef CAS PubMed.
- H. Kato, M. Suzuki, K. Fujita, M. Horie, S. Endoh, Y. Yoshida, H. Iwahashi, K. Takahashi, A. Nakamura and S. Kinugasa, Reliable size determination of nanoparticles using dynamic light scattering method for in vitro toxicology assessment, Toxicol. in Vitro, 2009, 23, 927–934 CrossRef CAS PubMed.
- L. Pinďáková, V. Kašpárková, K. Kejlová, M. Dvořáková, D. Krsek, D. Jírová and L. Kašparová, Behaviour of silver nanoparticles in simulated saliva and gastrointestinal fluids, Int. J. Pharm., 2017, 527, 12–20 CrossRef PubMed.
- M. van der Zande, R. J. Vandebriel, E. Van Doren, E. Kramer, Z. Herrera Rivera, C. S. Serrano-Rojero, E. R. Gremmer, J. Mast, R. J. B. Peters, P. C. H. Hollman, P. J. M. Hendriksen, H. J. P. Marvin, Ad A. C. M. Peijnenburg and H. Bouwmeester, Distribution, Elimination, and Toxicity of Silver Nanoparticles and Silver Ions in Rats after 28-Day Oral Exposure, ACS Nano, 2012, 6, 7427–7442 CrossRef CAS PubMed.
- G. Bachler, N. von Goetz and K. Hungerbühler, A physiologically based pharmacokinetic model for ionic silver and silver nanoparticles, Int. J. Nanomed., 2013, 8, 3365–3382 Search PubMed.
- Y. S. Kim, J. S. Kim, H. S. Cho, D. S. Rha, J. M. Kim, J. D. Park, B. S. Choi, R. Lim, H. K. Chang, Y. H. Chung, I. H. Kwon, J. Jeong, B. S. Han and I. J. Yu, Twenty-eight-day oral toxicity, genotoxicity, and gender-related tissue distribution of silver nanoparticles in Sprague-Dawley rats, Inhalation Toxicol., 2008, 20, 575–583 CrossRef CAS PubMed.
- A. P. Abreu and U. B. Kaiser, Pubertal development and regulation, Lancet Diabetes Endocrinol., 2016, 4, 254–264 CrossRef PubMed.
-
S. R. Ojeda and M. K. Skinner, in Knobil and Neill's Physiology of Reproduction, ed. T. M. Plant, D. W. Pfaff, J. R. G. Challis, D. M. d. Kretser, J. S. Richards and P. M. Wassarman, Academic Press, St Louis, 3rd edn, 2006, pp. 2061–2126. DOI:10.1016/B978-012515400-0/50043-9.
- D. Nesan and D. M. Kurrasch, Genetic programs of the developing tuberal hypothalamus and potential mechanisms of their disruption by environmental factors, Mol. Cell. Endocrinol., 2016, 438, 3–17 CrossRef CAS PubMed.
- C. Korenbrot, I. Huhtaniemi and R. Weiner, Preputial separation as an external sign of pubertal development in the male rat, Biol. Reprod., 1977, 17, 298–303 CrossRef CAS PubMed.
- R. S. Ge, G. R. Chen, Q. Dong, B. Akingbemi, C. M. Sottas, M. Santos, S. C. Sealfon, D. J. Bernard and M. P. Hardy, Biphasic effects of postnatal exposure to diethylhexylphthalate on the timing of puberty in male rats, J. Androl., 2007, 28, 513–520 CrossRef CAS PubMed.
- T. E. Stoker, L. G. Parks, L. E. Gray and R. L. Cooper, Endocrine-disrupting chemicals: prepubertal exposures and effects on sexual maturation and thyroid function in the male rat. A focus on the EDSTAC recommendations. Endocrine Disrupter Screening and Testing Advisory Committee, Crit. Rev. Toxicol., 2000, 30, 197–252 CrossRef CAS PubMed.
- Y. S. Kim, M. Y. Song, J. D. Park, K. S. Song, H. R. Ryu, Y. H. Chung, H. K. Chang, J. H. Lee, K. H. Oh and B. J. Kelman, Subchronic oral toxicity of silver nanoparticles, Part. Fibre Toxicol., 2010, 7, 1 CrossRef PubMed.
- H. Kanasaki, A. Oride, T. Mijiddorj, U. Sukhbaatar and S. Kyo, How is GnRH regulated in GnRH-producing neurons? Studies using GT1-7 cells as a GnRH-producing cell model, Gen. Comp. Endocrinol., 2017, 247, 138–142 CrossRef CAS PubMed.
- L. Hu, R. L. Gustofson, H. Feng, P. Ki Leung, N. Mores, L. Z. Krsmanovic and K. J. Catt, Converse Regulatory Functions of Estrogen Receptor-α and -β Subtypes Expressed in Hypothalamic Gonadotropin-Releasing Hormone Neurons, Mol. Endocrinol., 2008, 22, 2250–2259 CrossRef CAS PubMed.
- J. L. Temple, E. Laing, A. Sunder and S. Wray, Direct action of estradiol on gonadotropin-releasing hormone-1 neuronal activity via a transcription-dependent mechanism, J. Neurosci., 2004, 24, 6326–6333 CrossRef CAS PubMed.
- J. Lindzey, W. C. Wetsel, J. F. Couse, T. Stoker, R. Cooper and K. S. Korach, Effects of castration and chronic steroid treatments on hypothalamic gonadotropin-releasing hormone content and pituitary gonadotropins in male wild-type and estrogen receptor-alpha knockout mice, Endocrinology, 1998, 139, 4092–4101 CrossRef CAS PubMed.
- O. S. Rissland, The organization and regulation of mRNA-protein complexes, Wiley Interdiscip. Rev.: RNA, 2017, 8, e1369 CrossRef PubMed.
- P. Bargi-Souza, R. M. Romano, F. Goulart-Silva, E. L. Brunetto and M. T. Nunes, T(3) rapidly regulates several steps of alpha subunit glycoprotein (CGA) synthesis and secretion in the pituitary of male rats: Potential repercussions on TSH, FSH and LH secretion, Mol. Cell. Endocrinol., 2015, 409, 73–81 CrossRef CAS PubMed.
- K. De Gendt, J. V. Swinnen, P. T. Saunders, L. Schoonjans, M. Dewerchin, A. Devos, K. Tan, N. Atanassova, F. Claessens and C. Lécureuil, A Sertoli cell-selective knockout of the androgen receptor causes spermatogenic arrest in meiosis, Proc. Natl. Acad. Sci. U. S. A., 2004, 101, 1327–1332 CrossRef CAS PubMed.
- R.-S. Wang, S. Yeh, L.-M. Chen, H.-Y. Lin, C. Zhang, J. Ni, C.-C. Wu, P. A. di Sant'Agnese, K. L. deMesy-Bentley and C.-R. Tzeng, Androgen receptor in Sertoli cell is essential for germ cell nursery and junctional complex formation in mouse testes, Endocrinology, 2006, 147, 5624–5633 CrossRef CAS PubMed.
- S. Carreau, S. Lambard, C. Delalande, I. Denis-Galeraud, B. Bilinska and S. Bourguiba, Aromatase expression and role of estrogens in male gonad : a review, Reprod. Biol. Endocrinol., 2003, 1, 35 CrossRef PubMed.
-
L. O'Donnell, S. J. Meachem, P. G. Stanton and R. I. McLachlan, in Knobil and Neill's Physiology of Reproduction, ed. J. D. Neill, T. M. Plant, D. W. Pfaff, J. R. G. Challis, D. M. d. Kretser, J. S. Richards and P. M. Wassarman, Academic Press, St Louis, 3rd edn, 2006, pp. 1017–1069, DOI:10.1016/B978-012515400-0/50026-9.
- R. A. Hess, Estrogen in the adult male reproductive tract: A review, Reprod. Biol. Endocrinol., 2003, 1, 1–14 CrossRef.
- E. M. Eddy, T. F. Washburn, D. O. Bunch, E. H. Goulding, B. C. Gladen, D. B. Lubahn and K. S. Korach, Targeted disruption of the estrogen receptor gene in male mice causes alteration of spermatogenesis and infertility, Endocrinology, 1996, 137, 4796–4805 CrossRef CAS PubMed.
- T. F. Lucas, E. R. Siu, C. A. Esteves, H. P. Monteiro, C. A. Oliveira, C. S. Porto and M. F. Lazari, 17beta-estradiol induces the translocation of the estrogen receptors ESR1 and ESR2 to the cell membrane, MAPK3/1 phosphorylation and proliferation of cultured immature rat Sertoli cells, Biol. Reprod., 2008, 78, 101–114 CrossRef CAS PubMed.
- T. F. Lucas, C. Royer, E. R. Siu, M. F. Lazari and C. S. Porto, Expression and signaling of G protein-coupled estrogen receptor 1 (GPER) in rat sertoli cells, Biol. Reprod., 2010, 83, 307–317 CrossRef CAS PubMed.
- T. F. Lucas, M. F. Lazari and C. S. Porto, Differential role of the estrogen receptors ESR1 and ESR2 on the regulation of proteins involved with proliferation and differentiation of Sertoli cells from 15-day-old rats, Mol. Cell. Endocrinol., 2014, 382, 84–96 CrossRef CAS PubMed.
- G. Cacciola, T. Chioccarelli, S. Fasano, R. Pierantoni and G. Cobellis, Estrogens and Spermiogenesis: New Insights from Type 1 Cannabinoid Receptor Knockout Mice, Int. J. Endocrinol., 2013, 2013, 12 Search PubMed.
-
B. Robaire, B. T. Hinton and M.-C. Orgebin-Crist, in Knobil and Neill's Physiology of Reproduction, ed. D. N. Jimmy, M. P. Tony, W. P. Donald, R. G. C. John, M. d. K. David, S. R. JoAnne and M. Wassarman, Academic Press, St Louis, 3rd edn, 2006, pp. 1071–1148, DOI:10.1016/B978-012515400-0/50027-0.
- D. D. Ricker, The autonomic innervation of the epididymis: its effects on epididymal function and fertility, J. Androl., 1998, 19, 1–4 CAS.
- H. Woelders, Overview of in vitro methods for evaluation of semen quality, Reprod. Domest. Anim., 1991, 1, 145–164 Search PubMed.
- A. Amaral, B. Lourenco, M. Marques and J. Ramalho-Santos, Mitochondria functionality and sperm quality, Reproduction, 2013, 146, R163–R174 CrossRef CAS PubMed.
- C. Pang, D. Hristozov, A. Zabeo, L. Pizzol, M. P. Tsang, P. Sayre and A. Marcomini, Probabilistic approach for assessing infants’ health risks due to ingestion of nanoscale silver released from consumer products, Environ. Int., 2017, 99, 199–207 CrossRef CAS PubMed.
-
USA, United States Pharmacopoeia. The official compendia of standard, Arabswell, New York, USA, 30 edn, 2012 Search PubMed.
- R. M. Romano, S. N. Gomes, N. C. Cardoso, L. Schiessl, M. A. Romano and C. A. Oliveira, New insights for male infertility revealed by alterations in spermatic function and differential testicular expression of thyroid-related genes, Endocrine, 2017, 55, 607–617 CrossRef CAS PubMed.
- M. Romano, R. Romano, L. Santos, P. Wisniewski, D. Campos, P. Souza, P. Viau, M. Bernardi, M. Nunes and C. Oliveira, Glyphosate impairs male offspring reproductive development by disrupting gonadotropin expression, Arch. Toxicol., 2012, 86, 663–673 CrossRef CAS PubMed.
- G. Robb, R. Amann and G. Killian, Daily sperm production and epididymal sperm reserves of pubertal and adult rats, J. Reprod. Fertil., 1978, 54, 103–107 CrossRef CAS.
- P. Chomczynski and N. Sacchi, Single-step method of RNA isolation by acid guanidinium thiocyanate-phenol-chloroform extraction, Anal. Biochem., 1987, 162, 156–159 CrossRef CAS PubMed.
- K. J. Livak and T. D. Schmittgen, Analysis of relative gene expression data using real-time quantitative PCR and the 2(-Delta Delta C(T)) Method, Methods, 2001, 25, 402–408 CrossRef CAS PubMed.
Footnotes |
† Electronic supplementary information (ESI) available. See DOI: 10.1039/c7tx00236j |
‡ These authors contributed equally to the work. |
|
This journal is © The Royal Society of Chemistry 2018 |