Bifenthrin-induced neurotoxicity in rats: involvement of oxidative stress
Received
23rd July 2017
, Accepted 4th October 2017
First published on 12th October 2017
Abstract
Extensive use of synthetic pyrethroids has resulted in serious human health issues. Induction of oxidative stress is an important mechanism of action of most pesticides including pyrethroids. In the present study, we have elucidated the possible role of oxidative stress in bifenthrin-induced neurotoxicity. Adult male Wistar rats were administered bifenthrin (3.5 and 7 mg per kg body weight p.o.) for 30 days. Behavioral studies were conducted on a set of randomly selected rats from each treatment group after completion of treatment. Neurochemical parameters were assessed 24 h after the last dose was administered. The selected behavioral and neurochemical endpoints were also assessed 15 days after cessation of exposure to reveal whether the neurobehavioral changes produced by bifenthrin were temporary or permanent. Deficits in motor activity, motor incoordination, and cognitive impairment were observed after exposure to bifenthrin. Levels of biogenic amines viz. dopamine (DA) and its metabolites, i.e. 3,4-dihydroxyphenylacetic acid (DOPAC) and homovanillic acid (HVA), epinephrine (EPN), norepinephrine (NE), and serotonin (5-HT) altered in the frontal cortex, corpus striatum, and hippocampus of bifenthrin-treated rats. A decrease in the activity of acetylcholinesterase (AChE) occurred in all regions of the brain. Both doses of bifenthrin significantly induced lipid peroxidation (LPO) and increased protein carbonyl levels in the frontal cortex, corpus striatum, and hippocampus of rats. The activities of antioxidant enzymes, i.e. catalase, superoxide dismutase, and glutathione peroxidase, were also suppressed in all selected regions of the brain. A trend of recovery was, however, observed in all the behavioral and neurochemical endpoints 15 days after withdrawal of exposure. Oxidative stress seems to play an important role in bifenthrin-induced neurotoxicity. Our study suggests that long-term exposure to these compounds can produce detrimental effects.
Introduction
Bifenthrin (2-methyl-3-phenyl phenyl methyl (1S,3S)-3-(Z)-2-chloro-3,3,3-trifluoroprop-1-enyl 2,2-dimethylcyclopropane-1-carboxylate) is a third generation pyrethroid insecticide.1 Unlike earlier pyrethroids, bifenthrin is characterized by greater photostability and higher insecticidal activity.2 WHO classifies it under toxicity class II moderately hazardous pesticide.3 Bifenthrin is a type I synthetic pyrethroid widely used for agricultural and public health applications.4,5 It also finds extensive applications in the control of residential pests such as termites in urban areas. Type I pyrethroids devoid of the alpha-cyano group are characterized by aggressive sparring, increased sensitivity to external stimuli, tremors, and prostration referred to as T syndrome, whereas the type II compounds possess the alpha-cyano moiety and produce the CS syndrome, eliciting symptoms such as pawing, burrowing, profuse salivation, and coarse tremors progressing to choreoathetosis and clonic seizures.6,7 Bifenthrin is unique among pyrethroids; it does not contain the alpha moiety yet produces CS-type acute toxicity signs.8
Synthetic pyrethroids are established neurotoxicants; their primary site of action in mammals is the voltage-gated sodium channels in the central nervous system.9 Studies have documented that these compounds induce neurobehavioral effects in various animal species including humans. Pyrethroids have been reported to disturb the functioning of the brain by affecting the dopaminergic, cholinergic, and serotonergic systems.10–14
A number of environmental pollutants, including pesticides, are known to cause imbalance between the formation and removal of free radicals, thus leading to oxidative stress. Various cellular mechanisms are involved in alleviating the oxidative stress and repairing the damaged macromolecules. The mode of action of most pesticides involves induction of oxidative stress, causing damage to membrane lipids, proteins, and DNA.15 Involvement of free radicals in neurodegenerative diseases, such as Parkinson's disease and Alzheimer's diseases, is well established.16–18 Pyrethroids are known to stimulate the production of reactive oxygen species (ROS), resulting in oxidative damage in the organisms exposed to them.15,19,20
Although neurotoxicity of Type II pyrethroids is well-characterized, information regarding Type I compounds is limited.21,22 Extensive commercial and domestic applications of bifenthrin have raised concerns about its adverse effects on public health. Since both humans and animals are exposed to bifenthrin owing to its injudicious and extensive use, neurotoxic effects likely to be elicited need to be explored. Therefore, the purpose of the present study is to elucidate the toxic effects of bifenthrin on the antioxidant defense system that cause neurobehavioral alterations in adult male rats after sub-acute exposure to the pesticide.
Materials and methods
Chemicals
All reagents used were pure and of analytical grade. Bifenthrin (purity >98%) was procured from Sigma-Aldrich (St Louis, MO, USA).
Animals and treatment
Male wistar rats weighing 140–150 g and about 60 days old were used. Animals were obtained from the Indian Veterinary Research Institute (IVRI), Bareilly, India. They were maintained on the standard pellet diet (Ashirwad Industries, Chandigarh, India) and water ad-libitum in an air-cooled vivarium at 25 ± 2 °C with a 12 h light/dark cycle under standard hygienic conditions. The study was approved by the Institutional Animal Ethics Committee, and all experiments were carried out in accordance with the guidelines of the Committee for the Purpose of Control and Supervision of Experiments on Animals (CPCSEA), Ministry of Environment Forest and Climate Change (Government of India), New Delhi, India.
Experimental animals were divided into three groups. Bifenthrin was dissolved in corn oil at two dose levels (3.5 and 7 mg per kg body weight) and administered orally once daily for 30 days to the animals in the first two groups. The selected doses of bifenthrin correspond to 1/10 of LD50 (7 mg kg−1) and 1/20 of LD50 (3.5 mg kg−1).23,24 The third group was administered corn oil similarly and served as the control. The animals were observed daily and weighed at regular intervals.
A set of rats was randomly selected from each treatment group for behavioral studies after completion of the treatment. For neurochemical studies, a separate set of animals from each group was sacrificed 24 h after the last dose was administered. Brains were immediately removed, washed in ice cold saline, dissected into the frontal cortex, corpus striatum, and hippocampus, and processed for biochemical assays.25 To explore whether the neurobehavioral changes produced by bifenthrin were temporary or permanent, a set of animals from each treatment group was maintained as such for 15 days, and selected behavioral and neurochemical endpoints were then assessed.
Behavioural studies
Rotarod performance.
The motor coordination and balance of animals were studied using a rotarod (IMCORP, Ambala, India) by following a previously described procedure.26 A set of animals from each treatment group was trained to stay on the rotating rod until it achieved the criterion of staying on the rod for 60 s. Final observations were conducted by placing the rats on the rotating rod (25 rpm) with 180 s as the cut-off time. The time of fall from the rotating rod was determined as a measure of motor coordination, and scoring was carried out by a person blind to the treatment condition. Each rat was subjected to three consecutive trials after a gap of 5 min.
Open-field test.
The motor activity of rats was assessed using an open-field according to the method described by Soni et al. (2011) with slight modifications.27 The open-field apparatus consisted of a 60 × 60 cm2 field with 15 cm high walls. On the floor, 64 squares, each 7.5 × 7.5 cm, were made using black paint. For the observations, each animal was individually placed at the centre of the apparatus. Over a testing period of 5 minutes, the following behavioural parameters were noted: locomotion frequency (total number of sectors/squares entered by the animal with all four paws), rearing frequency (number of times the animal rose onto its hind paws), grooming frequency (number of times the animal touched or rubbed its snout with its paws), and the number of entries into the central region (4 central most squares). These behaviours were scored using a hand-operated counter and stop watch.
Spatial memory test – Morris water maze.
The Morris water maze (MWM) test was used to assess spatial learning in rats following the procedure described by Chen et al. (2010) with slight modification.28 The maze consists of a large circular tank, (150 cm diameter, 50 cm height) filled with water (28 ± 2 °C) and placed in the center of a large room with extra-maze cues. The water tank was divided into four equal quadrants: south-west (SW), north-west (NW), north-east (NE), and south-east (SE). A square platform (10 cm2) was submerged 2 cm below the water surface, located in the center of one of the quadrants. Rats were given four training trials each day for 4 consecutive days. The trials began on the 26th day of treatment, and for the withdrawal part of the study, the training session began on the 11th day following withdrawal of exposure. For each training trial, the rats were placed in water facing the pool wall at one of four positions (at the north, south, east or west pole) in a different order each day and were allowed to swim until they reached the platform. After 4 days of training, the platform was removed from the tank, and a 120 s spatial memory retention test was conducted. The animal uses the extra-maze cues as a reference to navigate and reach the platform. When the platform is removed from the tank, the animal utilizes the spatial information acquired during the training task and traverses the platform site. The spatial memory of animals was manifested by the time spent in the quadrant where the platform was placed.
Neurochemical studies
Biogenic amines and metabolites in brain regions.
Estimations of dopamine (DA), norepinephrine (NE), epinephrine (EPN), serotonin (5-HT), 3,4-dihydroxyphenylacetic acid (DOPAC), and homovanillic acid (HVA) in the frontal cortex, corpus striatum, and hippocampus were carried out by reversed phase high performance liquid chromatography (HPLC) using an electrochemical detector following the method of Kim et al. (1987) with minor modifications.29 The HPLC system (Waters, Melford, USA) consisted of a high-pressure isocratic pump (515 HPLC Pump), a sample injector valve, a C-18 reverse phase column (250 mm × 4 mm, particle size 5 μm), and an electrochemical detector (464 Pulsed electrochemical detector). Briefly, brain regions were homogenized in 0.1 M perchloric acid containing 3,4-dihydroxybenzylamine, an internal standard, at a final concentration of 25 ng ml−1 followed by centrifugation at 36
000g for 10 min. The supernatant obtained was then filtered through 0.25 mm nylon filters (Millipore, USA) and used for the determination of the levels of biogenic amines. After this, 20 μl of sample was injected into the injector port. An amperometric electrochemical detector with a glassy carbon and silver nitrate electrode was operated at a potential of +0.800 V with the sensitivity of 2 nA at ambient temperature. Data are obtained and analyzed via Empower 2 software (Waters, Melford, USA), and results are expressed as ng per g tissue weight. The HPLC facility at CSIR-IITR, Lucknow, was used.
Acetylcholinesterase activity in brain regions.
The activity of acetylcholinesterase was assayed following the method described by Ellman et al. (1961) using acetylthiocholine iodide as a substrate and 5,5′-dithiobis-2 nitrobenzoic acid (DTNB) as the coloring agent.30 The degradation of acetylthiocholine iodide has been measured at 412 nm, and the results are expressed as μmol ACTI hydrolyzed per min per mg protein.
Assay of lipid peroxidation and protein carbonyl levels.
As a measure of malondialdehyde (MDA) formation, levels of thiobarbituric acid reactive substances (TBARS) were estimated following the method of Ohkawa et al. (1979).31 The color produced by the reaction of TBA with malondialdehyde (MDA) was measured spectrophotometrically at 532 nm. The values are expressed as μmol per hour per mg protein. Protein carbonyl content in the brain regions was measured spectrophotometrically following the method of Levine et al. (1990) using 2,4-dinitrophenylhydrazine (DNPH) as the substrate.32 Carbonyl content (C) was calculated using a molar extinction coefficient (e) of 22.0 mM−1 cm−1 for aliphatic hydrazones.
SOD, CAT, and GPx activity in brain regions.
The activity of catalase in the brain regions was assayed spectrophotometrically following the method of Aebi (1984) using hydrogen peroxide (H2O2) as the substrate.33 The values have been expressed in μmole per min per mg protein. The superoxide dismutase assay was carried out by the pyrogallol autoxidation method following the procedure of Marklund and Marklund (1974).34 The results are expressed as unit per mg protein. The activity of glutathione peroxidase was measured using the method of Paglia and Valentine (1967).35 The values are expressed as nmol GSH oxidised per mg protein.
Protein estimation.
Protein content was assayed by the method of Lowry et al. (1951) using bovine serum albumin as a reference standard.36
Statistical analysis.
The data were analyzed using one way analysis of variance followed by Newman–Keuls test to compare all pairs of columns between groups. All values are expressed as mean ± SE. Values up to p < 0.05 have been considered to be significant, and p < 0.01 have been considered to be to be highly significant.
Results
Body and brain weight
Rats treated with bifenthrin for 30 days showed no overt sign of toxicity. The body weight of rats treated with bifenthrin was slightly reduced as compared to that of controls. No significant change occurred in the brain weight of the treated animals, and there was no gross abnormality in the brains of bifenthrin-administered rats.
Rotarod performance
The motor coordination of rats was assessed using a rotarod. Animals treated with bifenthrin exhibited impaired performance on the rotarod. The latency of fall from the rotating rod was reduced by 23% and 42% in the low and high dose groups, respectively, as compared to that of the controls. The animals, however, showed a trend of recovery 15 days after withdrawal of exposure (Fig. 1).
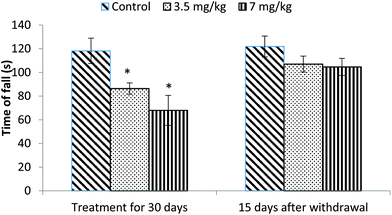 |
| Fig. 1 Effect on rotarod performance of rats following exposure to bifenthrin for 30 days and 15 days after withdrawal of exposure. Data were analyzed by one-way analysis of variance followed by Newman–Keuls test. Values are mean ± SEM of six animals in each group. * Significant difference from controls (p < 0.05); ** significant difference from controls (p < 0.01). | |
Open field
Exposure to bifenthrin for 30 days had a marked effect on the motor activity. As compared to the controls, rats of both treated groups (3.5 mg kg−1 and 7 mg kg−1) exhibited significant reduction (30%, p < 0.05 and 43%, p < 0.01) in the number of squares traversed in the open field (Fig. 2). A significant decrease in rearing frequency (20% and 48%) was observed in rats exposed to bifenthrin (3.5 mg kg−1 and 7 mg kg−1) as compared to that in the controls (Fig. 3). Both locomotion and rearing activities showed a trend of recovery following withdrawal of exposure. The entry of treated animals into the central region of the open field did not vary from that of the controls (Fig. 4). Similarly, the grooming frequency of bifenthrin-exposed animals remained similar to that of the controls (Fig. 5).
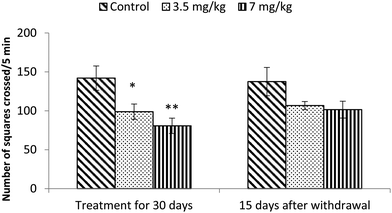 |
| Fig. 2 Effect on locomotion of rats in the open field following exposure to bifenthrin for 30 days and 15 days after withdrawal of exposure. Data were analyzed by one-way analysis of variance followed by Newman–Keuls test. Values are mean ± SEM of six animals in each group. * Significant difference from controls (p < 0.05); ** significant difference from controls (p < 0.01). | |
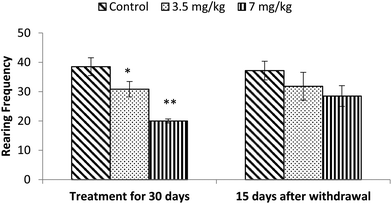 |
| Fig. 3 Effect on rearing of rats in the open field following exposure to bifenthrin for 30 days and 15 days after withdrawal of exposure. Data were analyzed by one-way analysis of variance followed by Newman–Keuls test. Values are mean ± SEM of six animals in each group. * Significant difference from controls (p < 0.05); ** significant difference from controls (p < 0.01). | |
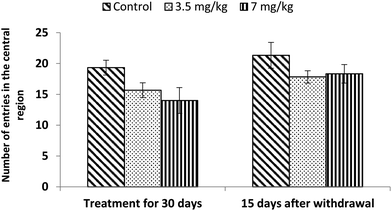 |
| Fig. 4 Effect on central area entries in the open field following exposure to bifenthrin for 30 days and 15 days after withdrawal of exposure. Data were analyzed by one-way analysis of variance followed by Newman–Keuls test. Values are mean ± SEM of six animals in each group. * Significant difference from controls (p < 0.05); ** significant difference from controls (p < 0.01). | |
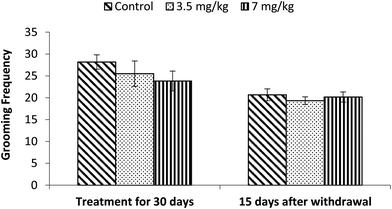 |
| Fig. 5 Effect on grooming of rats in the open field following exposure to bifenthrin for 30 days and 15 days after withdrawal of exposure. Data were analyzed by one-way analysis of variance followed by Newman–Keuls test. Values are mean ± SEM of six animals in each group. * Significant difference from controls (p < 0.05); ** significant difference from controls (p < 0.01). | |
Spatial memory
Acquisition and retention of spatial memory was tested using a Morris water maze. Both doses (3.5 mg kg−1 and 7 mg kg−1) of bifenthrin caused significant impairment of the spatial memory (29%, p < 0.05 and 49%, p < 0.01) of rats as the time spent in the target quadrant was found to be much less than that of controls. However, the observations conducted 15 days after withdrawal of exposure indicated a trend of recovery as the values remained similar to those of controls and no significant variation occurred (Fig. 6).
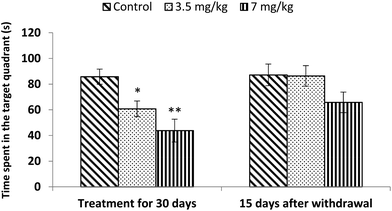 |
| Fig. 6 Effect on spatial memory of rats in the Morris water maze following exposure to bifenthrin for 30 days and 15 days after withdrawal of exposure. Data were analyzed by one-way analysis of variance followed by Newman–Keuls test. Values are mean ± SEM of six animals in each group. * Significant difference from controls (p < 0.05); ** significant difference from controls (p < 0.01). | |
Levels of biogenic amines
Exposure of rats to bifenthrin for 30 days had marked effects on the levels of biogenic amines in different regions of the brain. In the frontal cortex of rats exposed to bifenthrin at 3.5 and 7 mg kg−1, significant reductions in the levels of EPN (20% and 33%), DA (23% and 42%), DOPAC (27% and 43%), and 5-HT (10% and 30%) were observed as compared to the case of controls. However, NE and HVA levels were found to be significantly higher than those of the controls. The levels of all biogenic amines were, however, comparable to those of controls 15 days after cessation of exposure (Table 1).
Table 1 Levels of biogenic amines and metabolites in the frontal cortex of rats following treatment with bifenthrin for 30 days and 15 days after withdrawal of exposure
Brain region |
Treatment for 30 days |
15 days after withdrawal of exposure |
Frontal cortex |
Control |
3.5 mg kg−1 |
7 mg kg−1 |
Control |
3.5 mg kg−1 |
7 mg kg−1 |
Values are represented as mean ± SEM of five animals in each group. Values are expressed as ng per g tissue weight. Data have been analyzed by one-way analysis of variance followed by Newman–Keuls test. * Significant difference from controls (p < 0.05); ** significant difference from controls (p < 0.01). |
Norepinephrine |
256.6 ± 14.41 |
294.6 ± 27.95 |
468.9 ± 45.97** |
252 ± 25.69 |
288.1 ± 9.15 |
335.4 ± 33.85 |
Epinephrine |
365.1 ± 24.56 |
292.73 ± 20.82* |
243.02 ± 17.4** |
338.2 ± 24.45 |
262.42 ± 24.87 |
253.4 ± 21.79 |
Dopamine |
1127 ± 86.27 |
872.7 ± 52.37* |
657.3 ± 99.87** |
980.1 ± 53.53 |
856.12 ± 41.98 |
770.74 ± 66.72 |
3,4 Dihydroxyphenylacetic acid |
491.9 ± 35.4 |
357.7 ± 18.67* |
277.7 ± 22.18** |
468 ± 29.92 |
392.73 ± 11.82 |
358.43 ± 32.51 |
Homovanillic acid |
134.3 ± 7.26 |
154.21 ± 16.84 |
204.3 ± 12.54* |
124 ± 12.1 |
148 ± 16.88 |
160.2 ± 10.19 |
Serotonin |
1007 ± 51.44 |
909.51 ± 88.5 |
700.44 ± 66.8* |
1152 ± 46.51 |
1014 ± 55.19 |
945.7 ± 72.9* |
In the hippocampus region, bifenthrin dose-dependently reduced the levels of DA by 21% and 35%, those of DOPAC by 18% and 30%, those of 5-HT by 10% and 40%, and those of EPN by 18% and 36% of the control values. The levels of HVA (27% and 82%) and NE (28% and 43%) were found to be increased in the hippocampus of treated rats as compared to those of controls. A trend of recovery was obtained 15 days after withdrawal of exposure, and no significant variations occurred (Table 2).
Table 2 Levels of biogenic amines and metabolites in the hippocampus of rats following treatment with bifenthrin for 30 days and 15 days after withdrawal of exposure
Brain region |
Treatment for 30 days |
15 days after withdrawal of exposure |
Hippocampus |
Control |
3.5 mg kg−1 |
7 mg kg−1 |
Control |
3.5 mg kg−1 |
7 mg kg−1 |
Values are represented as mean ± SEM of five animals in each group. Values are expressed as ng per g tissue weight. Data have been analyzed by one-way analysis of variance followed by Newman–Keuls test. * Significant difference from controls (p < 0.05); ** significant difference from controls (p < 0.01). |
Norepinephrine |
294.2 ± 10.99 |
376.63 ± 30.77 |
421.8 ± 20.47* |
318.02 ± 23.77 |
327.93 ± 29.74 |
364.1 ± 24.42 |
Epinephrine |
281.4 ± 24.45 |
232.0 ± 17.45 |
178.8 ± 16.44** |
277.74 ± 24.6 |
253.3 ± 31.99 |
220.5 ± 14.49 |
Dopamine |
521.55 ± 37.8 |
413.6 ± 59.66 |
338.3 ± 33.7* |
501.55 ± 31.2 |
404.5 ± 32.99 |
371.9 ± 18.86* |
3,4 Dihydroxyphenylacetic acid |
47.06 ± 3.08 |
38.63 ± 4.35* |
32.9 ± 2.18* |
41.02 ± 3.23 |
39.04 ± 4.51 |
30.78 ± 1.7 |
Homovanillic acid |
31.25 ± 2.78 |
39.77 ± 4.61 |
56.87 ± 2.42** |
29.77 ± 3.43 |
30.39 ± 1.4 |
39.28 ± 6.89 |
Serotonin |
432.83 ± 20.76 |
387.8 ± 47.17 |
258.2 ± 19.86** |
507.61 ± 33.78 |
470 ± 29 |
409.11 ± 22.37 |
Levels of all biogenic amines were found to be altered by the pesticide in the corpus striatum of rats at both dose levels. Compared to those of controls, the levels of DA were reduced by 14% and 34%, the levels of DOPAC were reduced by 21% and 34%, the levels of EPN were reduced by 15% and 38%, and the levels of 5-HT were reduced by 16% and 30% in rats treated with bifenthrin at 3.5 mg kg−1 and 7 mg kg−1, respectively. However, the levels of HVA and NE were significantly (p < 0.05) higher than those of controls. The values observed 15 days after withdrawal of exposure indicated that the animals seemed to have recovered from the toxic effects of bifenthrin (Table 3).
Table 3 Levels of biogenic amines and metabolites in the corpus striatum of rats following treatment with bifenthrin for 30 days and 15 days after withdrawal of exposure
Brain region |
Treatment for 30 days |
15 days after withdrawal of exposure |
Hippocampus |
Control |
3.5 mg kg−1 |
7 mg kg−1 |
Control |
3.5 mg kg−1 |
7 mg kg−1 |
Values are represented as mean ± SEM of five animals in each group. Values are expressed as ng per g tissue weight. Data have been analyzed by one-way analysis of variance followed by Newman–Keuls test. * Significant difference from controls (p < 0.05); ** significant difference from controls (p < 0.01). |
Norepinephrine |
418.4 ± 42.69 |
471.7 ± 40.02 |
675 ± 31.6** |
384.6 ± 16.69 |
438.1 ± 29.28 |
476.2 ± 60.2 |
Epinephrine |
573.5 ± 62.46 |
486.1 ± 52.64 |
353.2 ± 17.85** |
605.3 ± 14.03 |
544.6 ± 54.16 |
513.4 ± 27.25 |
Dopamine |
5439 ± 269.7 |
4681 ± 265.2* |
3607 ± 140.3** |
5590 ± 268.3 |
5010 ± 120.6 |
4463 ± 286.3* |
3,4 Dihydroxyphenylacetic acid |
3462 ± 325.3 |
2731 ± 272.7* |
2300 ± 112.3** |
3398 ± 427.4 |
3050 ± 74.5 |
2622 ± 268.3 |
Homovanillic acid |
579.2 ± 45.68 |
764 ± 14.46* |
884.2 ± 28.1* |
610.72 ± 28.94 |
604.7 ± 39.95 |
813 ± 55.63 |
Serotonin |
929 ± 51.09 |
777.4 ± 55.4* |
650.5 ± 23.33** |
859.7 ± 53.32 |
844.6 ± 86.61 |
725.6 ± 74.37 |
Acetylcholinesterase activity
Compared to the case of controls, significant reductions in the activity of the acetylcholinesterase enzyme were found in the frontal cortex (14% and 18%, p < 0.01), hippocampus (16% and 24%, p < 0.01), and corpus striatum (14% and 26%, p < 0.05) of rats administered bifenthrin (3.5 mg kg−1 and 7 mg kg−1). However, the activity of this enzyme exhibited a trend of recovery 15 days after withdrawal of exposure (Fig. 7).
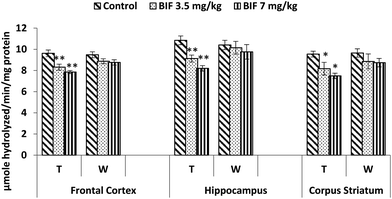 |
| Fig. 7 Acetylcholinesterase activity in the frontal cortex, hippocampus and corpus striatum of rats following exposure to bifenthrin for 30 days and 15 days after withdrawal of exposure. T = treatment for 30 days; W = 15 days after withdrawal of exposure. Data were analyzed by one-way analysis of variance followed by Newman–Keuls test. Values are represented as mean ± SEM of 5 rats in each group. * Significant difference from controls (p < 0.05), ** significant difference from controls (p < 0.01). | |
Lipid peroxidation and protein carbonyl levels
Bifenthrin-induced oxidative stress was evident by the increased lipid peroxidation and protein carbonyl content measured in the selected brain regions. Significant increases in the level of TBARS were observed in the frontal cortex (10%, p < 0.05 and 23%, p < 0.01), corpus striatum (19% and 26%, p < 0.01), and hippocampus (23%, p < 0.05 and 46%, p < 0.01) of bifenthrin-exposed rats as compared to the case of their respective controls. The TBARS level remained significantly higher in hippocampus (20%, p < 0.05) and corpus striatum (22%, p < 0.05) of rats treated with 7 mg kg−1 bifenthrin even after withdrawal of exposure; however, a general trend of recovery was seen (Fig. 8). Exposure to bifenthrin (3.5 mg kg−1 and 7 mg kg−1) for 30 days increased the protein carbonyl levels in the frontal cortex (24%, p < 0.05 and 42%, p < 0.01), hippocampus (20% and 42%, p < 0.01), and corpus striatum (15%, p < 0.05 and 35%, p < 0.01) as compared to the case of controls. Protein carbonyl levels remained significantly higher in the hippocampus and corpus striatum of rats administered the high dose of the test compound even after withdrawal of exposure (Fig. 9).
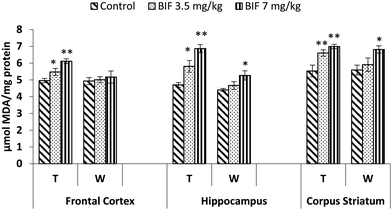 |
| Fig. 8 Effect on lipid peroxidation in the frontal cortex, hippocampus and corpus striatum of rats following exposure to bifenthrin for 30 days and 15 days after withdrawal of exposure. T = treatment for 30 days; W = 15 days after withdrawal of exposure. Data were analyzed by one-way analysis of variance followed by Newman–Keuls test. Values are represented as mean ± SEM of 5 rats in each group. * Significant difference from controls (p < 0.05), ** significant difference from controls (p < 0.01). | |
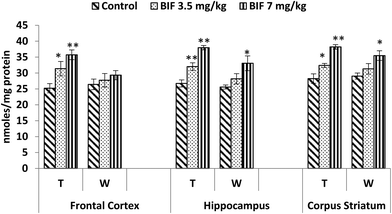 |
| Fig. 9 Effect on protein carbonyl levels in the frontal cortex, hippocampus and corpus striatum of rats following exposure to bifenthrin for 30 days and 15 days after withdrawal of exposure. T = treatment for 30 days; W = 15 days after withdrawal of exposure. Data were analyzed by one-way analysis of variance followed by Newman–Keuls test. Values are represented as mean ± SEM of 5 rats in each group. * Significant difference from controls (p < 0.05), ** significant difference from controls (p < 0.01). | |
CAT activity was found to be markedly affected by both doses of bifenthrin. CAT activity decreased by 12% (p < 0.05) and 26% (p < 0.01) in the frontal cortex, by 15% (p < 0.01) and 32% (p < 0.01) in the hippocampus, and by 18% (p < 0.01) and 24% (p < 0.01) in the corpus striatum as a result of exposure to 3.5 and 7 mg kg−1 bifenthrin, respectively, when compared with that of the vehicle-treated animals. The activity of this enzyme remained hampered in the hippocampus and corpus striatum even 15 days after cessation of exposure (Fig. 10). Activity of SOD was significantly impaired in the frontal cortex (32% and 37%, p < 0.01), corpus striatum (23% and 33%, p < 0.01), and hippocampus (21%, p < 0.05 and 38%, p < 0.01) following treatment with bifenthrin (3.5 and 7 mg kg−1) as compared to the case of controls. A trend of recovery was, however, seen in the SOD activity after withdrawal of exposure, but a significant decrease persisted in the frontal cortex of animals exposed to a high dose (Fig. 11). Administration of bifenthrin at 3.5 and 7 mg kg−1 decreased the GPx activity in the corpus striatum by 12% (p < 0.05) and 19% (p < 0.01), in the hippocampus by 16% (p < 0.05) and 23% (p < 0.01), and in the frontal cortex by 12% (p < 0.05) and 19% (p < 0.01), respectively, when compared with that of the control rats. The activity of GPx in bifenthrin-treated animals showed no variation from that of controls following withdrawal of exposure; this is indicative of a trend of recovery (Fig. 12).
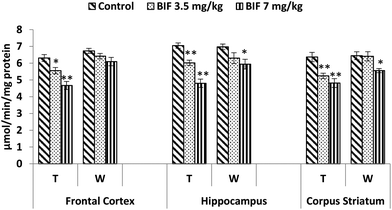 |
| Fig. 10 Effect on catalase activity in the frontal cortex, hippocampus and corpus striatum of rats following exposure to bifenthrin for 30 days and 15 days after withdrawal of exposure. T = treatment for 30 days; W = 15 days after withdrawal of exposure. Data were analyzed by one-way analysis of variance followed by Newman–Keuls test. Values are represented as mean ± SEM of 5 rats in each group. * Significant difference from controls (p < 0.05), ** significant difference from controls (p < 0.01). | |
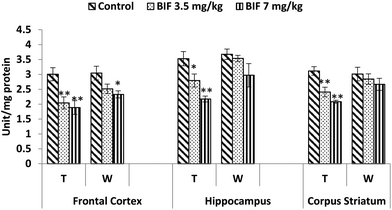 |
| Fig. 11 Effect on superoxide dismutase activity in the frontal cortex, hippocampus and corpus striatum of rats following exposure to bifenthrin for 30 days and 15 days after withdrawal of exposure. T = treatment for 30 days; W = 15 days after withdrawal of exposure. Data were analyzed by one-way analysis of variance followed by Newman–Keuls test. Values are represented as mean ± SEM of 5 rats in each group. * Significant difference from controls (p < 0.05), ** significant difference from controls (p < 0.01). | |
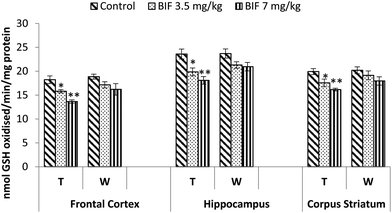 |
| Fig. 12 Effect on glutathione peroxidase activity in the frontal cortex, hippocampus and corpus striatum of rats following exposure to bifenthrin for 30 days and 15 days after withdrawal of exposure. T = treatment for 30 days; W = 15 days after withdrawal of exposure. Data were analyzed by one-way analysis of variance followed by Newman–Keuls test. Values are represented as mean ± SEM of 5 rats in each group. * Significant difference from controls (p < 0.05), ** significant difference from controls (p < 0.01). | |
Discussion
Restrictions on the use of organochlorine and organophosphate pesticides paved the way for the pyrethroid group of insecticides. Low mammalian toxicity and limited persistence in the environment led to the increased use of these compounds in agricultural and domestic settings. The toxicity of pyrethroids is mainly elicited by the parent compound, and their metabolism is considered as a detoxification mechanism.37 Pyrethroids are rapidly metabolized in the mammalian system; this results in the generation of reactive oxygen species (ROS). Oxidative and hydrolytic enzymes are involved in the metabolism of pyrethroids.37 Studies describing the oxidative stress mechanisms in pyrethroid-induced toxicity are limited. Few pyrethroids, such as cypermethrin, deltamethrin, fenvalerate, and lambda-cyhalothrin, have been demonstrated to induce oxidative stress.15,38–40 In the present study, we attempted to unveil the possible involvement of oxidative stress in bifenthrin-induced neurobehavioral alterations.
High metabolic rate and reduced capacity of cellular regeneration make the brain highly susceptible to damage by reactive oxygen species.41 Ample evidence provide an association between oxidative stress and neurodegeneration.42–44 Pyrethroids, being more hydrophobic than other classes of insecticides, easily attack the biological membranes.45
Dopamine, the principal neurotransmitter of the brain, mediates locomotion, motivated behavior, learning, and memory.46 The levels of DA and its metabolite DOPAC were found to be lowered by bifenthrin in a dose-dependent manner. The level of other DA metabolite HVA was, however, found to increase in all the regions of the brain. Drug-induced dopaminergic modulation may be detected by locomotion and rearing frequencies observed in an open-field.47 Administration of bifenthrin impaired the motor activity of rats, as evidenced by reduced locomotion and rearing frequencies in the open field, which may be correlated to dopaminergic neurodegeneration. Diminished frequency of these parameters indicates decreased exploratory and/or anxiogenic behaviour. Dopamine is known to be an anxiolytic neurotransmitter; low DA levels may, therefore, be the reason for the anxiety-like behavior observed in this study. Exposure to bifenthrin also impaired the performance of animals on the rotarod; this indicated motor incoordination. Numerous studies have previously reported impaired motor activity associated with dopaminergic neurodegeneration after exposure to pyrethroids.13,48,49 No significant differences occurred in the grooming frequencies of control and treated rats in this study. The time spent by the animals in the central region of the open-field arena also showed no treatment-related effect.
Bifenthrin caused dose-dependent decreases in the levels of 5-HT in the frontal cortex, hippocampus, and corpus striatum of rats. Several other studies have reported that pyrethroids modulate the release of serotonin.10,49,50 The NE levels were found to increase in all regions of the brain, and simultaneously, bifenthrin exposure lowered the levels of EPN.
The antioxidant enzymes CAT, GPx, and SOD play important roles in pyrethroid-induced oxidative stress.38,51,52 The activities of SOD, GPx, and CAT were significantly decreased at the tested dose levels of bifenthrin in comparison with those of the control. Our results are in concomitance with those reported by Yousef et al. (2006) and Ansari et al. (2012b), who showed significantly reduced activities of these antioxidant enzymes due to lambda-cyhalothrin and deltamethrin administration in rats.14,53 Reduction in the activities of these enzymes enhances the generation of superoxide and hydrogen peroxide radicals in the brain. This further leads to peroxidation of lipids in the membrane, resulting in the increased lipid peroxidation and protein carbonyl levels, as observed in this study.
TBARS is a major oxidation product of peroxidized polyunsaturated fatty acids.54 Being a hydrophobic compound, bifenthrin can accumulate in cell membranes and disrupt it. Elevated levels of TBARS in the brain indicate increased peroxidation of cell membranes and thus the presence of oxidative stress, which can lead to loss of membrane structure and function. Our findings are supported by previous studies reporting the toxicity of pyrethroids in various animal species.15,20,38 The tendency of bifenthrin to damage cellular proteins was apparent from the increased levels of protein carbonyls in the selected brain regions. These results are in agreement with those of some previous reports.14,55,56
The results illustrate that administration of bifenthrin results in inhibition of AChE activity in the hippocampus, frontal cortex, and corpus striatum. Numerous studies have previously reported that pyrethroids cause a decrease in AChE activity in the brains of exposed organisms.14,40 Decreased AChE might be associated with an increase in lipid peroxidation. Inhibition of AChE activity is known to decrease cellular metabolism and induce deformities of the cell membrane and disruption of metabolic and nervous activities.57
Decreases in learning and memory of rats were observed in this study. Oxidative stress has been implicated in cognitive impairment in both experimental animals and humans.58,59 Several workers have linked increased production of ROS to impaired learning and memory.58,60,61 Bifenthrin-induced oxidative stress in the hippocampus and other brain regions can be stipulated to be the mechanism involved in impaired learning and memory of rats in the Morris water maze. In the present study, decreased acetylcholinesterase activity can be associated with decreased learning response in bifenthrin-treated rats as anti-cholinesterases have been found to affect learning.62,63
A trend of recovery was observed 15 days after termination of the treatment. The animals seemed to recover from the toxic effects of bifenthrin. The changes in the behavioural and neurochemical endpoints assessed were transient and did not persist after withdrawal of exposure. The rapid metabolism of pyrethroids in mammals appears to be the reason for the transient toxicity of these compounds. It is the pyrethroid molecule that is mainly responsible for the toxic effects elicited, and metabolism is considered as a detoxification mechanism.37
A growing body of evidence supports the fact that free radicals are the most likely candidates responsible for producing neuronal changes mediating the behavioral deficits. Various environmental contaminants, such as metals, pesticides, and other pollutants, linked with etiology of neurodegeneration display a common feature, which is their ability to produce an increased amount of ROS. Impairment in motor activity, learning, and memory in bifenthrin-treated rats in this study could also be attributed to increased oxidative stress. The results further suggest that intense neurotoxic outcomes may be manifested if chronic exposure to bifenthrin occurs.
In conclusion, bifenthrin has the potential to manifest neurotoxicity. Enhanced oxidative stress evidenced by increased lipid peroxidation, protein carbonyl content, and perturbations in various antioxidant enzymes appears to be the mechanism involved in bifenthrin-induced neurotoxicity. Consequently, it may be suggested that proper care and precautions must be taken to avoid exposure to pyrethroids.
Conflicts of interest
There are no conflicts to declare.
Acknowledgements
Financial support provided by the Department of Science & Technology (DST-Women Scientist Scheme-A; Grant No. SR/LS-357/WOS-A/2011), New Delhi, India, for carrying out the study is thankfully acknowledged.
References
- S. Gupta, R. K. Sharma, R. K. Gupta, S. R. Sinha, R. Singh and V. T. Gajhbhiye, Persistence of new insecticides and their efficacy against insect pests of okra, Bull. Environ. Contam. Toxicol., 2009, 82, 243–247 CrossRef CAS PubMed.
- L. E. Mokrey and K. D. Hoagland, Acute toxicities of five synthetic pyrethroid insecticides to Daphnia magna and Ceriodaphnia dubia, Environ. Toxicol. Chem., 1989, 9, 1045–1051 CrossRef.
-
W.H.O., The WHO recommended classification of pesticides by hazard and guidelines to classification, World Health Organization, Geneva, 2009 Search PubMed.
- H. T. Nguyen, P. I. Whelan, M. S. Shortus and S. P. Jacups, Evaluation of bifenthrin applications in tires to prevent Aedes mosquito breeding, J. Am. Mosq. Control Assoc., 2009, 25, 74–82 CrossRef CAS PubMed.
- J. L. Domagalski, D. P. Weston, M. Zhang and M. Hladik, Pyrethroid insecticide concentrations and toxicity in streambed sediments and loads in surface waters of the San Joaquin Valley, California, USA, Environ. Toxicol. Chem., 2010, 29, 813–823 CrossRef CAS PubMed.
- S. A. Burr and D. E. Ray, Structure-activity and interaction effects of 14 different pyrethroids on voltage-gated chloride ion channels, Toxicol. Sci., 2004, 77(2), 341–346 CrossRef CAS PubMed.
- C. B. Breckenridge, L. Holden, N. Sturgess, M. Weiner, L. Sheets, D. Sargent, D. M. Soderlund, J. S. Choi, S. Symington, J. M. Clark, S. Burr and D. Ray, Evidence for a separate mechanism of toxicity for the Type I and the Type II pyrethroid insecticides, Neurotoxicology, 2009,(Suppl 1), S17–S31 CrossRef CAS PubMed.
- D. M. Soderlund, J. M. Clark, L. P. Sheets, L. S. Mullin, V. J. Picirillo, D. Sargent, J. T. Stevens and M. L. Weiner, Mechanisms of pyrethroid neurotoxicity: implications for cumulative risk assessment, Toxicology, 2002, 171, 3–59 CrossRef CAS PubMed.
- D. M. Soderlund, Molecular mechanisms of pyrethroid insecticide neurotoxicity: recent advances, Arch. Toxicol., 2012, 86(2), 165–181 CrossRef CAS PubMed.
- M. R. Martínez-Larrañaga, A. Anadón, M. A. Martínez, M. Martínez, V. J. Castellano and M. J. Díaz, 5-HT loss in rat brain by type II pyrethroid insecticides, Toxicol. Ind. Health, 2003, 19, 147–155 CrossRef PubMed.
- M. M. Hossain, T. Suzuki, I. Sato, T. Takewaki, K. Suzuki and H. Kobayashi, Neuromechanical effects of pyrethroids, allethrin, cyhalothrin and deltamethrin on the cholinergic processes in rat brain, Life Sci., 2005, 77(7), 795–807 CrossRef CAS PubMed.
- M. M. Hossain, T. Suzuki, N. Sato, I. Sato, T. Takewaki, K. Suzuki, E. Tachikawa and H. Kobayashi, Differential effects of pyrethroid insecticides on extracellular dopamine in the striatum of freely moving rats, Toxicol. Appl. Pharmacol., 2006, 217(1), 25–34 CrossRef PubMed.
- R. W. Ansari, R. K. Shukla, R. S. Yadav, K. Seth, A. B. Pant, D. Singh, A. K. Agarwal, F. Islam and V. K. Khanna, Involvement of dopaminergic and serotonergic systems in the neurobehavioral toxicity of lambda-cyhalothrin in developing rats, Toxicol. Lett., 2012, 211, 1–9 CrossRef CAS PubMed.
- R. W. Ansari, R. K. Shukla, R. S. Yadav, K. Seth, A. B. Pant, D. Singh, A. K. Agarwal, F. Islam and V. K. Khanna, Cholinergic dysfunctions and enhanced oxidative stress in the neurobehavioural toxicity of lambda-cyhalothrin in developing rats, Neurotoxic. Res., 2012, 22(4), 292–309 CrossRef CAS PubMed.
- H. Rehman, M. Ali, F. Atif, M. Kaur, K. Bhatia and S. Raisuddin, The modulatory effect of deltamethrin on antioxidants in mice, Clin. Chim. Acta, 2006, 369(1), 61–65 CrossRef CAS PubMed.
- M. A. Smith, C. A. Rottkamp, A. Nunomura, A. K. Raina and G. Perry, Oxidative stress in Alzheimer's disease, Biochim. Biophys. Acta, 2000, 1502(1), 139–144 CrossRef CAS.
- B. Halliwell, Role of free radicals in the neurodegenerative diseases: therapeutic implications for antioxidant treatment, Drugs Aging, 2001, 18(9), 685–716 CAS.
- O. Hwang, Role of oxidative stress in Parkinson's disease, Exp. Neurobiol., 2013, 22(1), 11–17 CrossRef PubMed.
- I. Sadowska-Woda, N. Wójcik, A. Karowicz-Bilińska and E. Bieszczad-Bedrejczuk, Effect of selected antioxidants in beta-cyfluthrin-induced oxidative stress in human erythrocytes in vitro, Toxicol. In Vitro, 2010, 24(3), 879–884 CrossRef CAS PubMed.
- F. M. El-Demerdash, Oxidative stress and hepatotoxicity induced by synthetic pyrethroids-organophosphate insecticides mixture in rat, J. Environ. Sci. Health, Part C: Environ. Carcinog. Ecotoxicol. Rev., 2011, 29(2), 145–158 CrossRef CAS PubMed.
- A. F. Godinho, S. L. Stanzani, F. C. Ferreira, T. C. Braga, M. C. Silva, J. L. Chaguri and C. A. Dias-Júnior, Permethrin chronic exposure alters motor coordination in rats: effect of calcium supplementation and amlodipine, Environ. Toxicol. Pharmacol., 2014, 37(2), 878–884 CrossRef CAS PubMed.
- J. Ahlbom, A. Fredriksson and P. Eriksson, Neonatal exposure to a type-I pyrethroid (bioallethrin) induces dose-response changes in brain muscarinic receptors and behaviour in neonatal and adult mice, Brain Res., 1994, 645(1–2), 318–324 CrossRef CAS PubMed.
-
U.S. EPA, Office of Pesticides and Toxic Substances, Fact Sheet No. 177 Bifenthrin, U.S. EPA, Washington, DC, 1988 Search PubMed.
-
A. Fecko, Environmental fate of bifenthrin, Environmental Monitoring and Pest Management Branch Department of Pesticide Regulation 830 K St, Sacramento, CA 95814, 1999 Search PubMed.
- J. Glowinski and L. L. Iversen, Regional studies of catecholamines in the rat brain. The disposition of 3H-norepinephrine 3H-dopamine and 3H-dopa in various regions of the brain, J. Neurochem., 1966, 13(8), 655–669 CrossRef CAS PubMed.
- F. Syed, L. P. Chandravanshi, V. K. Khanna and I. Soni, Beta-cyfluthrin induced neurobehavioral impairments in adult rats, Chem.-Biol. Interact., 2016, 243, 19–28 CrossRef CAS PubMed.
- I. Soni, F. Syed, P. Bhatnagar and R. Mathur, Perinatal toxicity of cyfluthrin in mice: Developmental and behavioral effects, Hum. Exp. Toxicol., 2011, 30(8), 1096–1105 CAS.
- Q. Chen, Y. Niu, R. Zhang, H. Guo, Y. Gao, Y. Li and R. Liu, The toxic influence of paraquat on hippocampus of mice: Involvement of oxidative stress, NeuroToxicology, 2010, 31, 310–316 CrossRef CAS PubMed.
- C. Kim, M. B. Speisky and S. N. Klarevla, Rapid and sensitive method for measuring norepinephrine, dopamine, 5HT and their major metabolites in rat brain by high performance liquid chromatography, J. Chromatogr., 1987, 386, 25–35 CrossRef CAS PubMed.
- G. L. Ellman, K. D. Courtney Jr., V. Andresand and R. M. Featherstone, A new and rapid colorimetric determination of acetylcholinesterase activity, Biochem. Pharmacol., 1961, 7, 88–95 CrossRef CAS PubMed.
- H. Ohkawa, N. Ohishi and K. Yagi, Assay for lipid peroxides in animal tissues bythiobarbituric acid reaction, Anal. Biochem., 1979, 95, 351–358 CrossRef CAS PubMed.
- R. L. Levine, D. Garland, C. N. Oliver, A. Amici, I. Climent, A. G. Lenz, B. W. Ahn, S. Shaltiel and E. R. Stadtman, Determination of carbonyl content in oxidatively modified proteins, Methods Enzymol., 1990, 186, 464–478 CAS.
-
H. Aebi, Catalase, in Methods of Enzymatic Analysis, ed. H. V. Bergmeyer, Acadamic press, 1974, vol. 2, pp. 674–684 Search PubMed.
- S. Marklund and G. Marklund, Involvement of the superoxide anion radical in the autoxidation of pyrogallol and a convenient assay for superoxide dismutase, Eur. J. Biochem., 1974, 47(3), 469–474 CrossRef CAS PubMed.
- D. E. Paglia and W. N. Valentine, Studies on the quantitative and qualitative characterization of erythrocyte glutathione
peroxidase, J. Lab. Clin. Med., 1967, 70, 158–169 CAS.
- O. H. Lowry, N. J. Rosenbrough, A. L. Farr and R. J. Randall, Protein measurement with the folin phenol reagent, J. Biol. Chem., 1951, 192, 265–275 Search PubMed.
- M. K. Ross, A. Borazjani, C. C. Edwards and P. M. Potter, Hydrolytic metabolism of pyrethroids by human and other mammalian carboxylesterases, Biochem. Pharmacol., 2006, 71(5), 657–669 CrossRef CAS PubMed.
- B. Giray, A. Gürbay and F. Hincal, Cypermethrin-induced oxidative stress in rat brain and liver is prevented by vitamin E or allopurinol, Toxicol. Lett., 2001, 118(3), 139–146 CrossRef CAS PubMed.
- F. M. El-Demerdash, M. I. Yousef, F. S. Kedwany and H. H. Baghdadi, Role of alpha-tocopherol and beta-carotene in ameliorating the fenvalerate-induced changes in oxidative stress, hemato-biochemical parameters, and semen quality of male rats, J. Environ. Sci. Health, Part B, 2004, 39(3), 443–459 CrossRef CAS.
- F. M. El-Demerdash, Lambda-cyhalothrin-induced changes in oxidative stress biomarkers in rabbit erythrocytes and alleviation effect of some antioxidants, Toxicol. In Vitro, 2007, 21(3), 392–397 CrossRef CAS PubMed.
- J. K. Andersen, Oxidative stress in neurodegeneration: cause or consequence?, Nat. Med., 2004, 10(Suppl), S18–S25 Search PubMed.
- X. Chen, C. Guo and J. Kong, Oxidative stress in neurodegenerative diseases, Neural Regener. Res., 2012, 7(5), 376–385 CAS.
- X. Wang, W. Wang, L. Li, G. Perry, H. G. Lee and X. Zhu, Oxidative stress and mitochondrial dysfunction in Alzheimer's disease, Biochim. Biophys. Acta, 2014, 1842(8), 1240–1247 CrossRef CAS PubMed.
- G. H. Kim, J. E. Kim, S. J. Rhie and S. Yoon, The Role of Oxidative Stress in Neurodegenerative Diseases, Exp. Neurobiol., 2015, 24(4), 325–340 CrossRef PubMed.
- F. Michelangeli, M. J. Robson, J. M. East and A. G. Lee, The conformation of pyrethroids bound to lipid bilayers, Biochim. Biophys. Acta, 1990, 1028, 49–57 CrossRef CAS.
- A. H. Tran, T. Uwano, T. Kimura, E. Hori, M. Katsuki, H. Nishijo and T. Ono, Dopamine D1 receptor modulates hippocampal representation plasticity to spatial novelty, J. Neurosci., 2008, 28(50), 13390–13400 CrossRef CAS PubMed.
- M. M. Bernardi, J. Palermo-Neto and H. De Souza, Effects of a single and long-term haloperidol treatment on open field behavior of rats, Psychopharmacology, 1981, 73(2), 171–175 CrossRef CAS PubMed.
- C. Nasuti, R. Gabbianelli, M. L. Falcioni, A. D. Stefano, P. Sozio and F. Cantalamessa, Dopaminergic system modulation, behavioral changes and oxidative stress after neonatal administration of pyrethroids, Toxicology, 2007, 229, 194–205 CrossRef CAS PubMed.
- A. K. Singh, M. N. Tiwari, O. Prakash and M. P. Singh, A current review of cypermethrin-induced neurotoxicity and nigrostriatal dopaminergic neurodegeneration, Curr. Neuropharmacol., 2012, 10(1), 64–71 CrossRef CAS PubMed.
- M. M. Hossain, T. Suzuki, J. R. Richardson and H. Kobayashi, Acute effects of pyrethroids on serotonin release in the striatum of awake rats: an in vivo microdialysis study, J. Biochem. Mol. Toxıcol., 2013, 27, 150–156 CrossRef CAS PubMed.
- M. Abdollahi, A. Ranjbar, S. Shadnia, S. Nikfar and A. Rezaie, Pesticides and oxidative stress: a review, Med. Sci. Monit., 2004, 10(6), 141–147 Search PubMed.
- E. Yarsan, A. Bilgili, M. Kanbur and S. Celik, Effects of deltamethrin on lipid peroxidation in mice, Vet. Hum. Toxicol., 2002, 44(2), 73–75 CAS.
- M. I. Yousef, T. I. Awad and E. H. Mohamed, Deltamethrin-induced oxidative damage and biochemical alterations in rat and its attenuation by vitamin E, Toxicol., 2006, 227(3), 240–247 CrossRef CAS PubMed.
- I. Celik and H. Suzek, Effects of subacute exposure of dichlorvos at sublethal dosages on erythrocyte and tissue antioxidant defense systems and lipid peroxidation in rats, Ecotoxicol. Environ. Saf., 2009, 72, 905–908 CrossRef CAS PubMed.
- H. Fetoui and R. Gdoura, Synthetic pyrethroid increases lipid and protein oxidation and induces glutathione depletion in the cerebellum of adult rats: ameliorative effect of vitamin C, Hum. Exp. Toxicol., 2012, 31(11), 1151–1160 Search PubMed.
- H. Fetoui, A. Feki, G. B. Salah, H. Kamoun, F. Fakhfakh and R. Gdoura, Exposure to lambda-cyhalothrin, a synthetic pyrethroid, increases reactive oxygen species production and induces genotoxicity in rat peripheral blood, Toxicol. Ind. Health, 2015, 31(5), 433–441 CrossRef CAS PubMed.
- A. Suresh, B. Sivaramakrishna, P. C. Victoriamma and K. Radhakrishnaiah, Comparative study on the inhibition of acetylcholinesterase activity in the freshwater fish Cyprinus carpio by mercury and zinc, Biochem. Int., 1992, 26(2), 367–375 CAS.
- K. Fukui, K. Onodera, T. Shinkai, S. Suzuki and S. Urano, Impairment of learning and memory in rats caused by oxidative stress and aging, and changes in antioxidative defense systems, Ann. N. Y. Acad. Sci., 2001, 928, 168–175 CrossRef CAS PubMed.
- M. Padurariu, A. Ciobica, L. Hritcu, B. Stoica, W. Bild and C. Stefanescu, Changes of some oxidative stress markers in the serum of patients with mild cognitive impairment and Alzheimer's disease, Neurosci. Lett., 2010, 469(1), 6–10 CrossRef CAS PubMed.
- M. M. Nicolle, J. Gonzalez, K. Sugaya, K. A. Baskerville, D. Bryan, K. Lund, M. Gallagher and M. McKinney, Signatures of hippocampal oxidative stress in aged spatial learning-impaired rodents, Neuroscience, 2001, 107, 415–431 CrossRef CAS PubMed.
- V. Bashkatova, J. Meunier, T. Maurice and A. Vanin, Memory impairments and oxidative stress in the hippocampus of in-utero cocaine-exposed rats, NeuroReport, 2005, 16(11), 1217–1221 CrossRef CAS PubMed.
- M. Farage-Elawar, Enzyme and behavioral changes in young chicks as a result of carbaryl treatment, J. Toxicol. Environ. Health, 1989, 26, 119–131 CrossRef CAS PubMed.
- S. Sarin and K. D. Gill, Biochemical and Behavioral Deficits in Adult Rat Following Chronic Dichlorvos Exposure, Pharmacol. Biochem. Behavior., 1988, 59(4), 1081–1086 CrossRef.
|
This journal is © The Royal Society of Chemistry 2018 |