DOI:
10.1039/C7NJ02574B
(Paper)
New J. Chem., 2018,
42, 380-387
Quaternized poly(styrene-co-vinylbenzyl chloride) anion exchange membranes: role of different ammonium cations on structural, morphological, thermal and physio-chemical properties†
Received
15th July 2017
, Accepted 21st November 2017
First published on 22nd November 2017
Abstract
Different ammonium cation containing anion exchange membranes are investigated in this study. Anion exchange polymers have been prepared from styrene and vinylbenzyl chloride monomers by free radical polymerization, followed by a quaternization process. Amines such as trimethylamine (TMA), tris(trimethylsilyl)amine (TTSA), hexamine (HMA), N,N,N′,N′-tetramethyl hexanediamine (TMHDA), and N,N,N′,N′-tetramethyl ethylenediamine (TMEDA) are used in this study. FT-IR and 13C-NMR results confirm the successful quaternization of the co-polymers with different amines. TGA results reveal that the thermal stability of the anion exchange polymers is as high as 165 °C. SEM images show that the membrane morphological features are tuned by the different ammonium cations. The ionic conductivity, ion-exchange capacity and water uptake values of the membranes also change with the different ammonium cations, which reveal the distinctive roles of the different amines used. Among the membranes, the membrane containing the trimethylammonium (TMA+) cation demonstrates the highest ionic conductivity, ion-exchange capacity and water uptake. On the other hand, the hexaminium (HMA+) containing anion exchange membrane displays the lowest ionic conductivity, ion-exchange capacity and water uptake due to the highly entangled structure of the polymer caused by the cross-linking of the polymer units.
1. Introduction
Electrolytes/separators play a vital role in electrochemical processes due to their inherent properties such as passage of ions and separation of electronic counterparts. On account of the leakage and transportation issues associated with liquid electrolytes, solid polymer electrolytes are more efficient for electrochemical processes and devices.1,2 In the 1960s, Nafion®, a proton exchange membrane (PEM), was developed and has been explored as an ideal electrolyte material in many electrochemical applications.3–9 Due to their highly acidic and corrosive nature, PEM based membranes require noble metal catalysts such as expensive platinum metal. To address these issues, alkaline anion exchange membranes (AAEMs) are considered as promising electrolyte materials.10–14 In the case of AAEMs, there is an enormous opportunity to use non-noble metal catalysts like Ni, Ag, and Pd.15,16 Nowadays, the development of AAEMs has a significant impact on electrochemical processes, and the fabrication of AAEMs with good conductivity and stability is a challenging one. The diffusion co-efficient of hydroxide ions is much lower than protons, which implies that AAEMs will have a lower ionic conductivity than their PEM counterparts.17,18 Among the anion exchange membranes reported in the literature, quaternary ammonium based membranes have been well studied and widely used in electrochemical systems. In the preparation of AAEMs, quaternization of membranes is the most important step for achieving better conductivity, since it decides the amount of exchangeable cationic groups. The quaternization process causes the formation of fixed cationic and movable anionic groups within the membrane.19,20 The properties of AAEMs mostly depend on the nature of fixed cationic ammonium groups attached with the polymer backbone. It is reported that ammonium groups which are far away from the polymer backbone may not alter the polymer backbone and there is an improvement in alkaline stability.21,22 The increase in the stability of AAEMs is attributed to the slower hydroxide diffusion within the membranes, which causes a lower effective hydroxide concentration in the vicinity of the ammonium cations.23 On the other hand, quaternary ammonium groups having high alkyl chain lengths reduce the ion-exchange capacity and increase the electrical resistance.24
In order to evaluate the properties of anion exchange membranes based on cationic groups (nitrogen functionality, chain length and structure), this study is devoted to the preparation of membranes containing different ammonium cation groups. In our previous work, we have prepared poly(styrene-co-vinylbenzyl chloride) (ST-co-VBC) based anion exchange membranes and investigated them in water electrolyzer systems.25 Accordingly, a poly(ST-co-VBC) copolymer is synthesized and used as a polymer backbone in this study. Amines such as trimethyl amine (TMA), tris(trimethyl silyl)amine (TTSA), hexamine (HMA), N,N,N′,N′-tetramethyl hexanediamine (TMHDA), and N,N,N′,N′-tetramethyl ethylenediamine (TMEDA) are used for quaternization and their roles in the structural and morphological behavior of the membranes are investigated. The resulting membranes are characterized using FT-IR, NMR, TGA and FE-SEM techniques. The membranes’ physio-chemical properties such as ionic conductivity, ion-exchange capacity and water uptake are also evaluated. The chemical structures of the different amines used in this study are shown in Fig. 1.
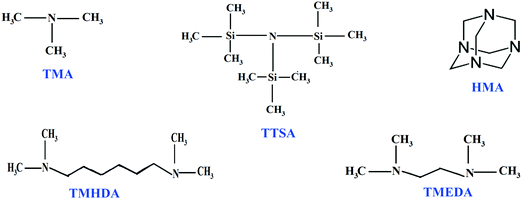 |
| Fig. 1 Chemical structures of tertiary amines. | |
2. Experimental
2.1. Materials
Styrene – 99% (Acros Organics), vinylbenzyl chloride – 99.9% (Acros Organics), and azobisisobutyronitrile (AIBN) – 0.2 M in toluene (Sigma-Aldrich) reagents were purchased and used in copolymer synthesis. The amines TMA – 50 wt% in water (Acros Organics), TTSA – 98% (Sigma-Aldrich), HMA – 99% (Otto Chemie), TMHDA – 99% (Acros Organics), and TMEDA – 99% (Acros Organics) were purchased and used as such in the amination process.
Solvents such as dimethylformamide (Acros Organics), N-methyl pyrrolidone (Avra Synthesis), toluene (RANKEM), chloroform LR (RANKEM) and methanol (SRL Ltd) were purchased and used as received. Potassium hydroxide, sodium hydroxide, and hydrochloric acid were of analytical grade and used as received. De-ionised water was used throughout the study.
2.2. Preparation of poly(ST-co-VBC) anion exchange membranes
2.2.1. Preparation of a poly(ST-co-VBC) copolymer.
A poly(ST-co-VBC) copolymer with styrene to vinylbenzyl chloride in a molar ratio of 1
:
0.33 was synthesized using free radical polymerization.25,26 The procedure is briefed as follows: styrene (8.8 g; 0.084 mol) and vinylbenzyl chloride (4.4 g; 0.028 mol) were taken in toluene and stirred. N2 gas was passed with vigorous stirring for 30 min. Then, the AIBN initiator was added to the above solution. The reaction mixture was stirred at 60 °C for 18 h. After completion of the reaction, the solution was cooled, and further addition of methanol yielded precipitation of the poly(ST-co-VBC) copolymer. The poly(ST-co-VBC) precipitate was dissolved in chloroform and re-precipitated with methanol. This process was repeated twice to obtain the poly(ST-co-VBC) copolymer with high purity. The obtained copolymer was dried in an oven at 70 °C.
2.2.2. Quaternization of the poly(ST-co-VBC) copolymer.
Quaternization of the poly(ST-co-VBC) copolymer was performed using the synthesized copolymer and various tertiary amines (Fig. 2). For this, 1 g of the poly(ST-co-VBC) copolymer was dissolved in 20 mL of DMF and 2 g of amine was added. The solution was stirred at 40 °C for 12 h. The solvent and unreacted amine present in the solution was evaporated using a rotary evaporator. Finally, the quaternized poly(ST-co-VBC) copolymer was collected as a “rubbery solid”, which implies that the quaternized polymer appears viscous (rubbery) in nature as it absorbs moisture readily from the atmosphere. Using the above procedure, quaternized poly(ST-co-VBC) copolymer containing different quaternary ammonium groups were synthesized using various amines (e.g., TMA, TTSA, HMA, TMHDA and TMEDA).
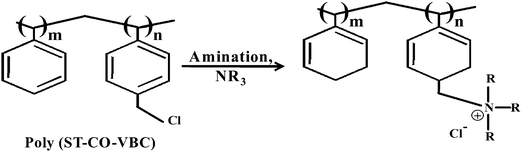 |
| Fig. 2 Synthesis scheme of quaternization of the poly(ST-co-VBC) copolymer with different amines, where NR3 = TMA, TTSA, HMA, TMHDA and TMEDA. | |
2.2.3. Preparation of membranes.
The quaternized poly(ST-co-VBC) copolymer was dissolved in NMP solvent and stirred for 1 h to obtain a homogeneous solution. The viscous polymer solution was cast on a glass plate by a solution casting method and dried at 135 °C overnight. After cooling, the membrane was peeled off from the glass substrate by the addition of de-ionised water. Then, the membrane was stored in de-ionised water for further measurements.
2.3. Physio-chemical properties
The physio-chemical properties such as ionic conductivity, ion-exchange capacity, water uptake and dimensional swelling were measured using standard procedures. For this, the Cl− form of the membrane was converted into the OH− form by immersing the membrane in 0.5 M KOH solution for 24 h.
The ionic conductivity (IC) of the membrane was measured by a two probe electrochemical AC impedance method. The OH− form of the membrane was rinsed with de-ionised water and clamped between two copper electrodes and the resistance of the membrane was measured. The IC (S cm−1) of the membrane was calculated using the equation:
where L = thickness of the membrane (cm), R = resistance of the membrane (ohms) and A = area of the membrane (cm2).
The ion-exchange capacity (IEC) of the membrane was calculated by a back titration method. The OH− form of the membrane was washed with de-ionised water and immersed in 0.1 M HCl for 24 h. Then, the solution was titrated against 0.1 M NaOH solution. The IEC (mmol g−1) of the membrane was calculated using the equation:
IEC = [N1(HCl) − N2(HCl)]/Wdry |
where N1(HCl) = strength of HCl before titration, N2(HCl) = strength of HCl after titration, and Wdry = dry weight of the membrane (g).
For water uptake measurements, the OH− form of the membrane was immersed in distilled water for 24 h. The surface water was removed carefully using tissue paper and the wet weight of the membrane was taken. Further, the membrane was dried in an oven at 90 °C overnight and then the dry weight was obtained. The water uptake of the membrane was calculated using the equation:27
Water uptake (%) = [(Wwet − Wdry)/Wdry] × 100 |
where
Wwet and
Wdry are the weights of the membrane in the wet and dry states in grams, respectively.
The dimensional swelling of the membrane was measured in a lengthwise direction in its OH− form. The dimensions of the membrane in the wet state were measured after removing the water from the surface of the membrane. Then the membrane was dried in an oven at 90 °C overnight and the dimensions of the membrane in the dry state were measured. The swelling of the membrane was calculated using the equation:27
Swelling (%) = [(Lwet − Ldry)/Ldry] × 100 |
where Lwet and Ldry are the lengths of the membrane in the wet and dry states in cm, respectively.
2.4. Characterization
FT-IR spectra of the synthesized poly(ST-co-VBC) copolymer and quaternized polymers were recorded in KBr pellets using a Bruker 27 Tensor FT-IR spectrophotometer with a resolution of 0.125 cm−1. Solid state 13C-NMR analysis of the polymerisation and quaternized products was performed on a Bruker Avance 400 MHz NMR instrument in Magic Angle Spinning (13C-MAS) mode.
The thermal degradation and stability of the prepared membranes were investigated using a simultaneous TGA/DTA analyser (Model: SDT Q600, TA Instruments) under a nitrogen atmosphere at a heating rate of 5 °C min−1 from 25 to 700 °C. The surface morphological features of the membranes were analysed using a scanning electron microscope (Model: TESCAN, Zeiss-Supra-55VP).
3. Results and discussion
3.1. NMR analysis
The chemical compositions of the various amines and the polymer precursor, poly(ST-co-VBC), were identified using 1H-NMR analysis and spectra are shown in Fig. S1–S6 (ESI†). The 1H-NMR spectra exclusively revealed the chemical compositions of the amines and the polymer precursor. Since the quaternized polymers show poor solubility in most of the NMR solvents, solid state 13C-NMR studies were performed to identify the anion exchange polymers. The solid state 13C-NMR spectra of the poly(ST-co-VBC) copolymer and quaternized poly(ST-co-VBC) polymers using different amines are shown in Fig. 3. From the NMR data, a poly(ST-co-VBC) spectrum shows peaks at chemical shifts of 39.3, 45.2, 87.9, 124.6, 141.0 and 166.4 ppm corresponding to aliphatic –CH, –CH2, –CH2Cl, aromatic –CH, aromatic C-4° (attached with CH–CH2) and aromatic C-4° (attached with CH2Cl), respectively. Upon quaternization with different amines, there are significant shifts in the peak positions of CH2Cl and aromatic C-4° (attached with CH2Cl) groups noticed, whereas the other peaks remain almost in the same positions. The CH2Cl peaks shifted to chemical shifts of 77.9, 81.0, 76.5, 76.8, and 76.7 ppm for the TMA, TTSA, HMA, TMHDA and TMEDA quaternized polymers, respectively. This shows that the CH2Cl group of poly(ST-co-VBC) that appeared at 87.9 ppm is shifted upfield after quaternization. Here, the CH2Cl peak positions of the HMA, TMHDA and TMEDA quaternized polymers are almost the same, since these amines contain multi-nitrogen groups. However, the peaks of the TMA and TTSA functionalized polymers appear at different chemical shifts due to the different structures and chemical environments of the two mono-amines. In contrast, the aromatic C-4° (attached with CH2Cl) group of poly(ST-co-VBC) that appeared at 166.4 ppm shifted downfield to 176.2, 172.1, 176.2, 176.0, and 176.1 ppm for the TMA, TTSA, HMA, TMHDA and TMEDA quaternized polymers, respectively. It is appropriate to mention here that our attempt to quantify the unreacted and quaternized CH2Cl groups was unsuccessful, since the CH2Cl peak of quaternized poly(ST-co-VBC) emerged as a broader peak in the solid state 13C-NMR spectra.
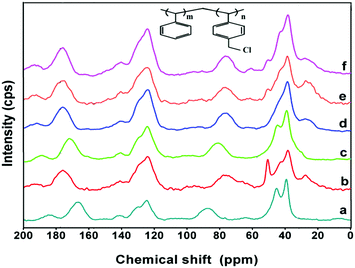 |
| Fig. 3
13C-NMR spectra of (a) poly(ST-co-VBC) copolymer; and quaternized poly(ST-co-VBC) with (b) TMA, (c) TTSA, (d) HMA, (e) TMHDA and (f) TMEDA. | |
3.2. FT-IR analysis
Fig. 4 shows the FT-IR spectra of the poly(ST-co-VBC) copolymer and quaternized polymers containing different ammonium cations. In the poly(ST-co-VBC) spectra, the peak at around 685 cm−1 is assigned to C–Cl stretching and the peak at 1258 cm−1 is due to –CH2Cl wagging,28 both resulting from the CH2Cl group present in VBC. In the case of the quaternized polymers, a new peak that appeared at ∼1072 cm−1 shows the presence of C–N stretching, which confirms the amination of the poly(ST-co-VBC) polymer with tertiary amines. It should be noted that the spectra of the quaternized polymers still show a C–Cl stretching peak, which implies that the quaternisation of the CH2Cl group is not 100%. In the case of the TMA and TTSA based quaternized polymers, the number of unreacted CH2Cl groups is high as can be seen from the intense C–Cl peak in their spectra. On the other hand, the amount of unreacted –CH2Cl is low (the C–Cl peak intensity is small) in the case of the HMA, TMHDA and TMEDA polymers. This is mainly due to the diverse structures and nitrogen contents of the different amines used. The mono-amines TMA and TTSA possess a single nitrogen in their structures and can quaternize only one CH2Cl group. On the other hand, the amines HMA, TMHDA and TMEDA have multi-nitrogen in their structures and can quaternize a higher number of CH2Cl groups. The broad peak present at ∼ 3400 cm−1 in all quaternized polymers represents the –OH stretching vibrations. Hence, the FT-IR results provide information about the extent of quaternization with the different tertiary amines.
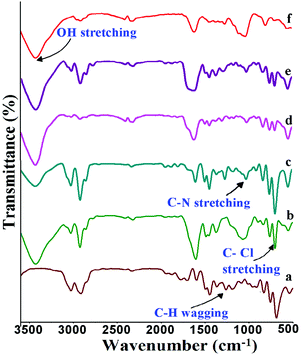 |
| Fig. 4 FT-IR spectra of (a) poly(ST-co-VBC) copolymer; and quaternized poly(ST-co-VBC) with (b) TMA, (c) TTSA, (d) HMA, (e) TMHDA and (f) TMEDA. | |
3.3. Morphological studies
The surface morphologies of the anion exchange membranes were studied using scanning electron microscopy, in order to observe and compare the surface uniformities of the different amine functionalized anion exchange polymers. Fig. 5 illustrates the SEM surface images of different ammonium cation functionalized anion exchange membranes taken at low and high magnifications (inset images). The images show that the HMA functionalized anion exchange membrane (Fig. 5a) has a rough surface with an entangled morphology. On the other hand, the TMA functionalised membrane (Fig. 5b) shows a rather smooth surface with a few surface impurities (shown in the inset). Nevertheless, the TMHDA and TMEDA functionalised membranes (Fig. 5c and d) reveal smooth surfaces. The membrane morphological features are mainly tuned by the structures of the different amines and the membrane fabrication process itself.
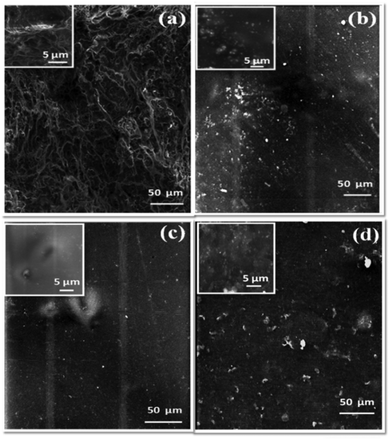 |
| Fig. 5 Scanning electron microscopy (SEM) surface images of the anion exchange membranes using (a) HMA, (b) TMA, (c) TMHDA and (d) TMEDA. | |
During the fabrication of membranes via a solution casting process, the TMA functionalized polymer results in a homogeneous solution due to the absence of cross linking within the polymer units. On the other hand, TMHDA and TMEDA having two tertiary nitrogen groups may undergo cross-linking in a controlled manner and the polymer solution also be uniform. However, HMA having four equivalent tertiary nitrogens can undergo uncontrolled cross-linking and form a heterogeneous solution. Thus, the polymer solutions that form membranes ground the difference in morphological features.
3.4. Thermal studies
The thermal stability of the anion exchange membranes plays a vital role in their application in electrochemical systems. The thermal properties of the anion exchange polymer depend on the chemical and structural nature of the polymer backbone and the cationic ion exchange groups. The thermal properties of the quaternized poly(ST-co-VBC) polymers are evaluated using a TGA/DTA analyser. Fig. 6 shows the thermogravimetric curves of the anion exchange polymers prepared using the different amines. From the TG curves, all the polymers show 3 stage degradation typical of anion exchange polymers, reflecting the “thermostable” behavior of these polymers.29 The first degradation at around 165 °C is due to the degradation of quaternary ammonium groups, the second at around 300 °C is due to the degradation of the main polymer backbone and the third above 500 °C is due to carbonization. The degradation temperature increases in the following order for the different amine functionalized polymers, TTSA < TMA < TMHDA < TMEDA < HMA. The observed trend is explained as follows: The TTSA functionalised polymer exhibits low thermal stability due to the presence of a bulky ammonium group compared to the TMA functionalized polymer. In the case of TMHDA, TMEDA, HMA functionalized anion exchange polymers, the high thermal stability is due to the presence of multi-nitrogen in their structures, which can cause cross-linking within the polymer units. HMA that possesses four tertiary nitrogens in its structure can have a higher degree of cross-linking than the other ammonium cations and reveals the highest thermal stability among all polymers.
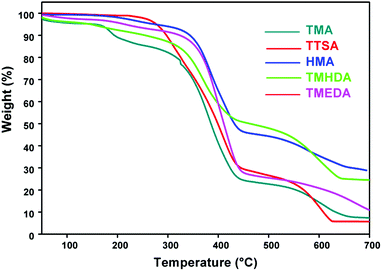 |
| Fig. 6 Thermogravimetric (TG) curves of the quaternized poly(ST-co-VBC) polymers using the different tertiary amines. | |
Differential scanning calorimetry (DSC) is a tool which can provide information about the phase transition such as the Tg, melting and crystallisation behavior of polymers. Since the TGA results (Fig. 6) reveal the degradation behavior of anion exchange polymers, it is important to see the micro-structural changes that occurred with temperature changes. Fig. 7 shows the DSC curves of the anion exchange polymers prepared with the different amines. From the DSC curves, all the polymers (except TTSA) reveal a characteristic endothermic peak with an onset at ∼380 °C. An exception is that the TTSA polymer shows an endothermic peak with an onset at 300 °C, due to the bulky anion exchange groups present in its structure. These results show that the polymer degradation occurs above 300 °C, which is consistent with the TGA results. Despite the fact that the DSC results do not show any sharp transitions, it confirmed the degradation steps. The unusual behavior of the DSC curve observed with an exothermic peak at ∼400 °C solely in the TMEDA polymer may be due to some post-cross-linking within the polymer30 or some instrumental error during the DSC analysis.
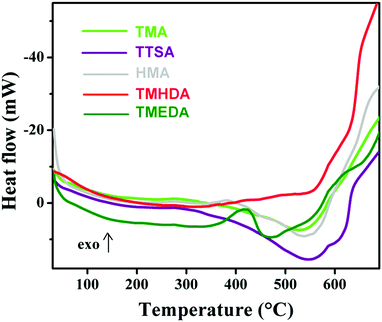 |
| Fig. 7 Differential scanning calorimetry (DSC) curves of the quaternized poly(ST-co-VBC) polymers with the different tertiary amines. | |
3.5. Physio-chemical properties
The membrane's physio-chemical properties such as ionic conductivity, ion-exchange capacity, water uptake and swelling ratio were evaluated in order to employ the membranes in water electrolysers. The physio-chemical properties of the anion exchange membranes are shown in Fig. 8. The conductivity results indicate that the anion exchange membrane using TMA shows high ionic conductivity ∼2.0 mS cm−1. However, the membranes using TTSA, HMA, TMHDA, and TMEDA show ionic conductivities in the 0.1–0. 3 mS cm−1 range. The difference in conductivity mainly arises from the structures and nitrogen groups of the different amines used. The TMA cation is smaller in size and can effectively form an ionic pathway for OH− conduction. On the other hand, TTSA, HMA, TMHDA, and TMEDA are larger in size and also most of them have multi-nitrogen in their configurations which form cross-linked structures. This will reduce the ionic conduction due to the densely packed polymer entangled structure. The ion-exchange capacity of the anion exchange membranes also follows a similar trend to the ionic conductivity results; however, their difference in IEC among various membranes is rather small. The ion-exchange capacity is in the 0.6–1.0 mmol g−1 range as shown in Table 1. Water uptake is one such property which reflects the presence of hydrophilic groups in the membrane. Moreover, the ionic conductivity of the membranes depends on the water uptake.
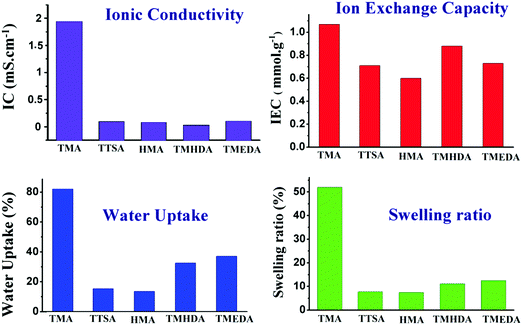 |
| Fig. 8 IC, IEC, water uptake and swelling ratio values of poly(ST-co-VBC) anion exchange membranes. | |
Table 1 Physio-chemical properties of the anion exchange membranes using the different amines
Membrane |
Ionic conductivity (mS cm−1) |
Ion-exchange capacity (mmol g−1) |
Water uptake (%) |
Swelling ratio (%) |
Membrane-1 (TMA) |
1.94 |
1.07 |
82.21 |
52.0 |
Membrane-2 (TTSA) |
0.1 |
0.71 |
15.36 |
7.7 |
Membrane-3 (HMA) |
0.1 |
0.60 |
13.60 |
7.4 |
Membrane-4 (TMHDA) |
0.08 |
0.88 |
32.62 |
11.1 |
Membrane-5 (TMEDA) |
0.12 |
0.73 |
37.08 |
12.5 |
Following a similar trend to IEC, the TMA functionalised membrane shows the highest water uptake of ∼80%. The TMHDA and TMEDA functionalised membranes show intermediate water uptake in the 32–37% range. This is mainly due to the crosslinked structure of the polymers in the case of the TMHDA and TMEDA membranes. It should be noted that the TTSA and HMA functionalized membranes show low water uptake in the 13–15% range, which reflects the highly hydrophobic behavior of the membranes. The HMA membrane with a high degree of cross-linking can reduce the water uptake significantly. The dimensional stability of the membrane is characterised using the swelling ratio and it reflects the mechanical properties of the membrane. The swelling results indicate that the TMA functionalised membrane shows the highest swelling, whereas the other membranes show low swelling degrees. The swelling mainly results from the presence of water in the membrane and it has a strong dependence on the water uptake capacity of the polymer. It is noteworthy that the TTSA membrane does not follow a general trend as the other membranes in terms of IC, water uptake and swelling ratio. Regardless of a mono-amine, TTSA is unique in its structure and chemical composition compared with the other amines. It may undergo slow amination reaction with chloromethylated polymers due to the presence of bulky alkyl groups. The direct amination of halogenated hydrocarbons or chloromethylated polymers using TTSA has scarcely been reported in the literature. However, Janik et al.31 reported the amination of monohaloboranes at high temperatures under reflux conditions. Since the degree of quaternization and the polymer structure can’t be controlled using TTSA, it may be complex to correlate its properties with the other amine functionalized polymers.
To summarise, this work demonstrates a comparative study on different ammonium cation containing anion exchange membranes. The effect of mono-, di- and tetra-amines with different alkyl groups and chain lengths on the membrane properties have been studied. Previously, Sata et al.32 investigated mono-amines with different alkyl chain lengths and reported that the alkaline stability decreases as the chain length of the ammonium cations increased. On the other hand, Komkova et al.24 studied the role of di-amines with varying alkyl chain lengths and found that the membrane properties and alkaline stability improve with alkyl chain length. Nevertheless, this work reveals that short-chain mono-amine containing TMA membranes show superior physio-chemical properties to the other membranes. Still, the mono-amine TTSA functionalised membrane exhibited lower IC and IEC than the TMA functionalised membrane due to the presence of bulky alkyl groups in the former. The presence of bulky alkyl groups not only reduces the degree of quaternization, but also hinders the anionic mobility that can decrease the conductivity. In the case of di-amines (TMHDA and TMEDA), the amine with a shorter alkyl chain length showed somewhat higher IC than the amine with a longer chain length. The tetra-amine HMA functionalized membrane showed poor IC, IEC and water uptake compared to the other membranes which needs to be understood well. This anomalous behavior is due to the high cross-linking of the polymer units that can vastly reduce the water uptake and ionic mobility of the membrane.33,34
4. Conclusions
A series of poly(ST-co-VBC) anion exchange membranes with different quaternary ammonium groups were prepared in this study. The different ammonium groups play a vital effect on the structural, morphological, thermal and physio-chemical properties of the membranes. The morphological features of the membranes were tuned by introducing the different ammonium groups into the membrane, in which the tetra-amine (HMA) functionalized polymer revealed a cross-linked morphology with a rough surface. On the other hand, the mono (TMA) and di-amine (TMHDA and TMEDA) containing membranes showed smooth surfaces due to the absence or low degree of cross-linking. The thermal stability of the membranes increased with increase in the number of nitrogen groups present in the amines which can be attributed to the cross-linking. The thermal stabilities of the membranes follow the order: TTSA < TMA < TMHDA < TMEDA < HMA.
The mono-amine containing membrane showed superior physio-chemical properties to the other membranes. The TMA functionalised membrane showed the highest ionic conductivity of ∼2 mS cm−1 with an ion-exchange capacity of 1.07 mmol g−1. Also, the high water uptake (82.21%) of the membrane increased the swelling to 52%, which is detrimental to its mechanical properties. Among the membranes, the tetra-amine HMA functionalized membrane showed poor IC, IEC and water uptake compared to the other membranes. Despite the low ionic conductivity of our membranes compared with the literature reported values, this study stimulated a fundamental understanding on the role of cationic ammonium groups and their importance in designing anion exchange membranes with excellent properties.
Conflicts of interest
There are no conflicts of interest to declare.
Acknowledgements
The authors gratefully acknowledge DST-SERB for providing financial support through a grand-in-aid project (SB/FT/CS-097/2013). The authors also acknowledge Mr P. Murugesan, C&M lab, CSIR-CECRI, for carrying out TGA/DSC analysis and Dr S. Radhakrishnan, Central Instrumentation Facility, CSIR-CECRI, for extensive NMR studies.
References
-
T. Sata, Ion exchange membranes: preparation, characterization, modification and application, Royal Society of Chemistry, Cambridge, UK, 2004 Search PubMed
.
- K. D. Kreuer, J. Membr. Sci., 2001, 185, 29–39 CrossRef CAS
.
- B. C. H. Steele and A. Heinzel, Nature, 2001, 414, 345–352 CrossRef CAS PubMed
.
- N. H. Jalani, K. Dunn and R. Datta, Electrochim. Acta, 2005, 51, 553–560 CrossRef CAS
.
- N. Jia, M. C. Lefebvre, J. Halfyard, Z. Qi and G. Peter, Electrochem. Solid-State Lett., 2000, 3, 529–531 CrossRef CAS
.
- L. Hong and B. E. Logan, Environ. Sci. Technol., 2004, 38, 4040–4046 CrossRef
.
- S. A. Grigoriev, V. I. Porembsky and V. N. Fateev, Int. J. Hydrogen Energy, 2006, 31, 171–175 CrossRef CAS
.
- J. Xi, Z. Wu, X. Qiu and L. Chen, J. Power Sources, 2007, 166, 531–536 CrossRef CAS
.
- A. A. Gronowski and H. L. Yeager, J. Electrochem. Soc., 1991, 138, 2690–2697 CrossRef CAS
.
- G. Merle, M. Wessling and K. Nijmeijer, J. Membr. Sci., 2011, 377, 1–35 CrossRef CAS
.
- C. M. Tuan and D. Kim, J. Membr. Sci., 2016, 511, 143–150 CrossRef CAS
.
- T. A. Sherazi, J. Y. Sohn, Y. M. Lee and M. D. Guiver, J. Membr. Sci., 2013, 441, 148–157 CrossRef CAS
.
- M. K. Cho, H. Y. Park, S. Choe, S. J. Yoo, J. Y. Kim, H. J. Kim, D. Henkensmeier, S. Y. Lee, Y. E. Sung, H. S. Park and J. H. Jang, J. Power Sources, 2017, 347, 283–290 CrossRef CAS
.
- C. W. Hwang, H. M. Park, C. M. Oh, T. S. Hwang, J. Shim and C. S. Jin, J. Membr. Sci., 2014, 468, 98–106 CrossRef CAS
.
- S. Gu, R. Cai, T. Luo, Z. W. Chen, M. W. Sun, Y. Liu, G. H. He and Y. S. Yan, Angew. Chem., Int. Ed., 2009, 48, 6499 CrossRef CAS PubMed
.
- J. R. Varcoe and R. C. T. Slade, Fuel Cells, 2005, 5, 187 CrossRef CAS
.
- C. Chen, Y. L. S. Tse, G. E. Lindberg, C. Knight and G. A. Voth, J. Am. Chem. Soc., 2016, 138, 991–1000 CrossRef CAS PubMed
.
- B. V. Merinov and W. A. Goddard III, J. Membr. Sci., 2013, 431, 79–85 CrossRef CAS
.
- J. Wang, S. Li and S. Zhang, Macromolecules, 2010, 43, 3890–3896 CrossRef CAS
.
- Y. Xiong, Q. L. Liu and Q. H. Zeng, J. Power Sources, 2009, 193, 541–546 CrossRef CAS
.
- C. G. Arges and V. Ramani, Proc. Natl. Acad. Sci. U.S.A., 2013, 110, 2490–2495 CrossRef CAS PubMed
.
- J. Pan, Y. Li, J. Han, G. W. Li, L. Tan, C. Chen, J. Lu and L. Zhuang, Energy Environ. Sci., 2013, 6, 2912–2915 CAS
.
- B. Bauer, H. Strathmann and F. Effenberger, Desalination, 1990, 79, 125–144 CrossRef CAS
.
- E. N. Komkova, D. F. Stamatialis, H. Strathmann and M. Wessling, J. Membr. Sci., 2004, 244, 25–34 CrossRef CAS
.
- S. Vengatesan, S. Santhi, S. Jeevanantham and G. Sozhan, J. Power Sources, 2015, 284, 361–368 CrossRef CAS
.
- T. Ameringer, F. Ercole, K. M. Tsang, B. R. Coad, X. Hou, A. Rodda, D. R. Nisbet, H. Thissen, R. A. Evans, L. Meagher and J. S. Forsythe, Biointerphases, 2013, 8, 16 CrossRef PubMed
.
- L. Sun, J. Guo, J. Zhou, Q. Xu, D. Chu and R. Chen, J. Power Sources, 2012, 202, 70–77 CrossRef CAS
.
-
D. L. Pavia, G. M. Lampman and G. S. Kriz, Introduction to spectroscopy, Thomson Learning, USA, 3rd edn, 2001 Search PubMed
.
- Y. C. Cao, X. Wang, M. Mamlouk and K. Scott, J. Mater. Chem., 2011, 21, 12910–12916 RSC
.
- M. Grochowicz, B. Gawdzik, M. Jaćkowsk and B. Buszewski, J. Therm. Anal. Calorim., 2013, 114, 955–961 CrossRef CAS
.
- J. Janik, C. K. Narula, E. G. Gulliver, E. N. Duesler and R. T. Paine, Inorg. Chem., 1988, 27, 1223–1227 Search PubMed
.
- T. Sata, M. Tsujimoto, T. Yamaguchi and K. Matsusaki, J. Membr. Sci., 1996, 112, 161–170 CrossRef CAS
.
- H. Y. Li and Y. L. Liu, J. Mater. Chem. A, 2013, 1, 1171–1178 CAS
.
- S. Vengatesan, S. Santhi, G. Sozhan, S. Ravichandran, D. J. Davidson and S. Vasudevan, RSC Adv., 2015, 5, 27365–27371 RSC
.
Footnote |
† Electronic supplementary information (ESI) available. See DOI: 10.1039/c7nj02574b |
|
This journal is © The Royal Society of Chemistry and the Centre National de la Recherche Scientifique 2018 |