DOI:
10.1039/C7FO01569K
(Paper)
Food Funct., 2018,
9, 491-501
Aggregates of octenylsuccinate oat β-glucan as novel capsules to stabilize curcumin over food processing, storage and digestive fluids and to enhance its bioavailability†
Received
9th October 2017
, Accepted 22nd November 2017
First published on 28th November 2017
Abstract
Self-aggregates of octenylsuccinate oat β-glucan (AOSG) have been verified as nanocapsules to load curcumin, a representative of hydrophobic phytochemicals. This study primarily investigated the stability of curcumin-loaded AOSGs over food processing, storage and digestive fluids. Curcumin in AOSGs showed better stability over storage and thermal treatment than its free form. Curcumin loaded in AOSGs stored at 4 °C in the dark exhibited higher stability than that at higher temperatures or exposed to light. Approximately 18% of curcumin was lost after five freeze–thaw cycles. Curcumin in AOSG was more stable than its free form in mimetic intestinal fluids, attesting to the effective protection of AOSG for curcumin over digestive environments. When curcumin-loaded AOSG travelled across mimetic gastric and intestinal fluids, curcumin was tightly accommodated in the capsule, while it rapidly escaped as the capsule reached the colon. Interestingly, the curcumin loaded in AOSG generated higher values of Cmax and area under the curve than did its free counterpart. These observations showed that AOSG is a powerful vehicle for stabilizing hydrophobic phytochemicals in food processing and storage, facilitating their colon-targeted delivery and enhancing their bioavailability.
Introduction
Strategies for improving colon health have recently risen in importance, most likely because more than one million new cases of colorectal cancer (CRC) are diagnosed worldwide each year.1 The majority (approximately 80%) of CRC cases have been linked to such risk factors as chronic bowel inflammation, environmental and food-borne mutagens, and specific intestinal commensals and pathogens, rather than heritable genetic changes.2 In inflammatory bowel diseases (IBD), such as Crohn's disease and ulcerative colitis, interactions between gut microbiota and the host's immune system can be easily disturbed by suboptimal diets.3 Certainly, IBD patients are at an increased risk of colon cancer. Moreover, the colon can be exposed to a high level of reactive oxygen species (ROS) when fried and barbecued foods are consumed. ROS are definitively related to the generation of free radicals, DNA damage and the initiation of carcinogenesis.4
Numerous studies have provided evidence that bioactive compounds (BCs), including flavonoids, sterols and carotenoids, benefit the health of the colon, and their mechanisms have been ascribed to their abilities to inhibit pathogenic bacteria and inactivate ROS.5,6 Thus, a sufficient concentration of these BCs in the colon may be effective in preventing and treating CRC. However, common BCs are extremely sensitive to light, heat, and oxidation. In the meantime, BCs often suffer serious degradation during their travel in the human gastrointestinal (GI) tract.7 Thus, challenges in fabricating colon-specific foods with BCs lie in stabilizing them in food processing and the GI tract. In this context, a protective system for BCs should be put in place when designing a colon-specific functional food, and the system should satisfy the standards listed as follows.8 First, the system should be able to withstand the harsh conditions encountered in food processing and food digestion in the GI tract without showing significant damage or degradation. Second, the system should have a high capacity for accommodating the specific BC as cargo, especially for lipophilic molecules with poor water solubility. Third, the system should retain the cargo or only present a limited release rate when it travels through the stomach and small intestine and finally disintegrate in the colon and fully release the cargo.
In developing various food-grade delivery systems for BCs, proteins, starches and lipids have been selected as common material for building the vesicle.9–11 Usually, in these systems, BCs are loaded into vesicles through non-covalent interactions occurring between the cargo and polymer molecules, such as hydrophobic interactions, hydrogen bonds and van der Waals forces.12 However, due to the digestibility of food proteins, starches and lipids, delivery systems based on them are liable to disintegrate in the upper GI and fail to deliver the cargo into the colon. In this regard, oat β-glucan is an indigestible polysaccharide with high bioactivity and high fermentability in the colon by gut flora.13 It was hypothesized that oat β-glucan would be a perfect candidate polymer in the construction of food-grade, colon-specific delivery vesicles for BCs.
Currently, amphiphilic polysaccharides are thought to have the ability to form aggregates in aqueous solution and could be applied as vesicles for carrying or delivering hydrophobic BCs. Curcumin is a commonly used representative of hydrophobic BCs, possessing poor water solubility (11 ng mL−1) but diverse bioactivity, including such properties as anti-inflammation, anticancer, and antimicrobiology.14 Considering that starches and gum acacia modified with succinic groups are commercially available and permitted in the food industry, octenylsuccination was adopted to prepare amphiphilic oat β-glucan. Octenylsuccinate oat β-glucan (OSG) was synthesized, and the resultant molecules could spontaneously assemble to form nanoscale aggregates, which were demonstrated to possess a good capacity in solubilizing and carrying curcumin.14,15 Furthermore, the encapsulation by OSG aggregates (AOSG) could stabilize β-carotene upon thermal treatment, freeze–thaw and storage.16 As a subsequent work following these results, the feasibility of using AOSGs as colon-specific delivery capsules of lipophilic BCs was tested in the present study by evaluating their storage, thermal and freeze–thaw stabilities, in vitro stability and release profiles in various mimetic digestive fluids and in vivo bioavailability in rats.
Materials and methods
Chemicals and reagents
Commercial oat β-glucan (80 wt% β-glucan content) was purchased from Zhangjiakou Yikang Biological Technology Co., Ltd (Zhangjiakou, China). 2-Octen-1-ylsuccinic anhydride (Product No. 416487) was purchased from Sigma Chemical Co. (Saint Louis, MO, USA). Curcumin (bis-(4-hydroxy-3-methoxyphenyl)-1,6-heptadiene-3,5-dione, 98% purity) was supplied by Adamas Reagent Co., Ltd (Shanghai, China). Carmellose sodium (CMC-Na) of analytical grade was obtained from Kelong Chemical Reagent Co. (Chengdu, China). Chromatography grade methanol, acetonitrile and formic acid were also used. All chemicals were used as received without further purification.
Preparing curcumin-loaded AOSG
Oat β-glucan was octenylsuccinated according to a previously proposed method, and its degree of substitution was determined by elemental analysis using an Elementar CHNS analyzer (Vario EL III, Elementar Analysensysteme GmbH, Germany).14 β-Glucan with a weight-average molecular weight of 16.8 × 104 g mol−1 was applied as a substrate to synthesize OSG with a 0.0199 degree of substitution.15 Freeze-dried OSG powder (51.4 mg) was dissolved in 20 mL of distilled water by heating at 100 °C for 2 min with continuous stirring. After complete cooling at room temperature, the volume of the resultant solution was brought to 20 mL with distilled water. The pH of the OSG solution was adjusted to 5.9. Curcumin powder (15 mg) was introduced into the solution, and the suspension was homogenized in a homogenizer (IKA T18 Basic, IKA-Werke GmbH & Co., Staufen, Germany). The resultant homogenate was continuously stirred at 37.5 °C with a stirrer input power of 4.4 W for 24 h to enable the transfer of curcumin into AOSG. After that time elapsed, the suspension was centrifuged at 5600g for 10 min to remove the free solid-state curcumin. Due to the very poor water solubility of curcumin, the curcumin mass in the resultant supernatant was regarded as the curcumin taken up by AOSG, which was determined to be 21.16 ± 0.66 μg mL−1. Upon freeze-drying, the level of curcumin in the final product was 8.46 ± 0.26 μg mg−1. Consequently, the AOSG-entrapped curcumin completely dissolved in water giving a clear and well-dispersed solution with curcumin's natural colour. In contrast, free curcumin remained undissolved and presented as visibly microscopic flakes in the water.
Determining food processing and storage stabilities
Due to the poor water solubility of curcumin, dimethyl sulfoxide (DMSO)–water (5
:
95, v/v) was used as a medium to prepare the solution of free curcumin with the same curcumin concentration equivalent as that in the AOSG suspension (21 μg mL−1). Curcumin retention (%) was applied as the main index to scale the stability, which was defined as the percentage of curcumin remaining in the suspension after treatment. After the specific treatment, the suspension was extracted with methanol and the resultant supernatant was subjected to HPLC for curcumin determination as described later. In addition, the storage stability was reflected by the changes in intensity-based mean size and the polydispersity index (PDI) of AOSG. The intensity-based mean size and PDI of AOSG were determined based on dynamic light scattering (DLS) using a Zetasizer Nano ZS90 system (Malvern Instruments Ltd, UK) equipped with a He–Ne laser (633 nm) and 90° collecting optics.
To determine the thermal stability, portions of curcumin-loaded AOSG were placed in transparent glass bottles. The intensity of thermal treatment was achieved using different temperature–time combinations. The temperature variables included 50 °C, 60 °C, 70 °C, 80 °C, or 90 °C while the time variables were 10 min, 20 min or 30 min. In total, fifteen combinations were tested. The control sample, i.e., the solution of free curcumin, was treated at 50 °C, 60 °C, 70 °C, 80 °C, or 90 °C for 10 min
To determine the freeze–thaw stability, portions of curcumin-loaded AOSG were placed in centrifuge tubes. Each freeze–thaw cycle was defined as placing the sample in a freezer at −18 °C for 2 h and thawing at room temperature (25 °C) for 2 h. At the end of each freeze–thaw cycle, three tubes were withdrawn to determine curcumin retention.
To determine the storage stability, portions of curcumin-loaded AOSG were placed in transparent glass bottles. The portions were evenly sorted into three groups with each group containing 15 glass bottles. The first and second groups were stored at 25 °C with or without natural light exposure, respectively. The third was kept in the dark at 4 °C. The test lasted for 20 days. The free contrast was stored at 25 °C with natural light exposure.
Determining stabilities over mimetic gastric and intestinal fluids17,18
The base of the mimetic gastric fluid (MGF) was prepared by mixing 2.0 g of NaCl, 7.0 mL of 37% HCl and 900 mL of double-distilled water, while the base of the mimetic intestinal fluid (MIF) consisted of 6.8 g of KH2PO4 dissolved in 650 mL of double-distilled water plus 190 mL of 0.2 N NaOH.19 The final pH values of the MGF and MIF bases were adjusted to 1.2 ± 0.1 and 7.2 ± 0.1, respectively. Prior to use, pepsin (3.2 g, in MGF base) or pancreatin (10.0 g, in MIF base) was added and the volume of the base was brought to 1000 mL with double-distilled water to produce MGF and MIF, respectively. Due to the difference in the salt concentration between the tested samples and MGF or MIF, AOSG suspension and free curcumin dissolved in 25% (v/v) aqueous DMSO with the same concentration of salt, as in mimetic fluids, were prepared. In detail, brine with a doubled concentration of salts, as in MGF or MIF, was prepared and later mixed with the above-mentioned AOSG suspension in an equivalent volume. The resultant suspension containing 10.58 μg mL−1 curcumin was directly applied as the sample suspension in the following release tests. To determine its stability in MGF or MIF, the above-obtained sample AOSG suspension or the aqueous DMSO solution of free curcumin was mixed with the MGF or MIF medium (1
:
4, v/v) and incubated in a 37 °C water bath with 120 rpm agitation. At predetermined time points, 1 mL of the solution was sampled to determine curcumin by HPLC as described later.
The in vitro release of curcumin from AOSG in MGF or MIF was determined using a dialysis bag with a molecular weight cut-off of 12
000 Da. To retrieve the released curcumin and prevent its sedimentation in bags, MGF- or MIF-containing 10% (v/v) Tween-80 was used as the liquid outside the dialysis bag. To perform dialysis, the dialysis bag was filled with 3 mL of the sample suspension and then immersed in 50 mL of Tween 80-incorporated digestive fluid in a 500 mL glass breaker. The system was incubated at 37 °C with continuous stirring at 50 rpm. Next, 1 mL aliquots of the solution outside the bags were withdrawn to determine the curcumin amount at predetermined time intervals, and the same amount of the releasing media was replaced immediately upon withdrawal. The curcumin released (%) was defined as the percentage of curcumin in the solution outside the dialysis bag.
Determining curcumin released from AOSG over mimetic colonic fluid
In preparing mimetic colonic fluid (MCF), the collection of faecal samples, the preculture of human faecal microbiota and the preparation of the growth medium were performed according to a previous report.20 Fresh faecal samples were collected from three healthy, male, young, IBD-free students (23–28 years old) in our laboratory. To determine curcumin released from AOSG, the preculture, growth medium, and curcumin-loaded AOSG suspension were mixed in a ratio of 2
:
2
:
1 (v/v/v). An aliquot of the mixture was introduced into a sterile tube under anaerobic conditions. After being vortexed for 1 min, the tube was filled with N2, sealed and then incubated at 37 °C on a shaking table at 120 rpm. After the required incubation time elapsed, the tube was cooled to room temperature and centrifuged at 3000g for 5 min. The resultant supernatant was subjected to HPLC to determine the curcumin retained in AOSG, as described later. To recognize the effect of faecal microbiota on the release of curcumin from AOSG, a contrast test with a previously autoclaved preculture (121 °C, 15 min) was simultaneously conducted.
Determining the bioavailability of AOSG-entrapped curcumin
Thirty male Sprague-Dawley rats, weighing 250 ± 26 g, were purchased from Chongqing Tengxin, Inc. (Chongqing, China). The rats were individually housed in stainless steel screen-bottomed cages in a room maintained at 23 ± 2.0 °C with approximately 50% relative humidity. The room was illuminated from 8
:
00 am to 20
:
00 pm. The rats were acclimated by feeding them a commercial normal diet (Chongqing Tengxin Inc., Chongqing, China) for 2 weeks. Before the drug administration, the rats were fasted overnight. The ones in the entrapped curcumin group were administered 200 mg per kg bw of freezing-dried curcumin-loaded AOSG (equivalent to 1.69 mg per kg bw entrapped curcumin), while the rats in the free curcumin group were given 250 mg per kg bw of free curcumin. The application of free curcumin in such a high dosage was due to its low oral bioavailability.21 The preliminary experiments suggested its necessity in guaranteeing the presence of curcumin in animal blood. Owing to the poor water solubility of free curcumin and to prevent insoluble particles from blocking the gavage needle, it was suspended in 0.2% (w/v) aqueous CMC-Na solution at a concentration of 250 mg mL−1. A previous study reported that CMC-Na did not show any absorption enhancement to curcumin.22 In parallel, freeze-dried curcumin-loaded AOSG was suspended in the same media at a concentration of 200 mg mL−1. The drug suspensions were orally administered at a dosage of 1.0 mL per rat by direct stomach intubation. Prior to drug administration, thirty rats were randomly given a serial number from 1 to 30. The rats were evenly assigned into ten groups according their serial number sequence. The first and last five groups were respectively applied in free and entrapped curcumin tests corresponding to five post-administration time points (30 min, 60 min, 120 min, 180 min and 300 min). Then, the rats in each group were sequentially administered with the drug at a 5 min interval. The rat was executed immediately after its pre-set time elapsed and the blood sample was collected in a heparinized micro-centrifuge tube (100 IU heparin per mL of blood). The plasma sample was obtained by centrifuging the tube at 3000g for 10 min at −40 °C. A 200 μL aliquot of plasma was mixed with 200 μL acetonitrile, vortexed for 1 min and centrifuged at 5600g for 10 min23 The resultant acetonitrile layer was directly injected to the HPLC system for curcumin determination. The animal study complied with all institutional and national guidelines, as per the national standard “Laboratory Animal-Requirements of Environment and Housing Facilities” (GB 14925-2010). The protocol was approved by the Institutional Animal Care and Use Committee of Southwest University.
Determining curcumin by HPLC
In the present study, curcumin in the above-mentioned samples was determined according to a previously proposed HPLC method.24 The sample was mixed with methanol in a volume ratio of 1
:
4 in a centrifugation tube. After being tightly capped, the tube was intensively shaken for 2 min. Then, the mixture was centrifuged at 5600g for 10 min. The obtained supernatant was filtered through a 0.45 μm nylon membrane filter and the filtrate was applied onto the HPLC column for curcumin quantification. A Shimadzu LC-20A series HPLC system (Shimadzu Co., Kyoto, Japan) was used, which was equipped with a model LC-20AT pump, an SPD-M20A diode array detector (set at 420 nm) and a Thermo BDS C18 column (250 × 4.6 mm i.d., 5 μm). The column was maintained at 35 °C. The mobile phases were composed of 0.2% (v/v) aqueous formic acid (A) and acetonitrile (B). The gradient conditions were as follows: 0–10 min, 35–65% B; 10–15 min, 65–70% B; 15–20 min, 70–35% B. The analysis was conducted at a flow rate of 0.7 mL min−1 with an injection volume of 10 μL. In this case, curcumin was eluted at 16.11 min and its concentration (y, ng mL−1) was accurately quantified using its peak area (x) by using an external standard method (y = −7.4 × 105 + 2.3 × 105x, R2 = 0.9875).
Statistical analysis
All measurements were performed at least in triplicate. The data were subjected to an analysis of variance (ANOVA), and Tukey's HSD test was carried out to compare the means. Differences were considered to be significant at p < 0.05. Statistical computations and analyses were conducted using SPSS19.0.
Results and discussion
Thermal stability
Polymeric aggregate has attracted increasing attention in the food industry and has been widely explored as a novel strategy to improve both nutritional and technical functionalities of food ingredients.25 Apparently, the stability of the active substance loaded in polymeric aggregates during food processing and storage is highly crucial to their application in food products. Thermal treatment is a widely used technique in processing many foods with varying objects, such as sterilization, blanching, evaporation and concentration. In realizing its intended goals, thermal treatment often does some harmful impacts to the product, such as browning, deteriorating flavour, destabilizing food structure and even degrading thermally sensitive compounds.26 Obviously, when curcumin-loaded AOSG was used as an ingredient of a thermally treated food, the structural integrity of AOSG and stability of curcumin against thermal treatment are fateful in realizing its functionality. With these concerns, the thermal stability of curcumin-loaded AOSG was assessed as a function of thermal time and temperature (Table 1).
Table 1 The thermal stability of curcumin loaded in the aggregates of octenylsuccinate oat β-glucan (AOSG)a
Heating time (min) |
Temperature (°C) |
Curcumin retention (%) |
Size (nm) |
PDI |
In AOSG |
Free |
The stability of curcumin was expressed as its retention percentage upon thermal treatment with free curcumin in 5% DMSO as the control, while the stability of OSG aggregates was expressed by the changes in the average aggregate size and its polydispersity index (PDI) upon thermal treatment. Values are expressed as mean ± standard deviation of triplicate analysis.
a–eValues bearing different superscript lowercase letters within the same column with the same heating time are significantly different (p < 0.05). A–CValues bearing different superscript capital letters within the same column under the same temperature are significantly different (p < 0.05). *Data in the free column bearing the superscript asterisk are significantly different from their corresponding values in the In AOSG column (p < 0.05). ND, not determined. |
— |
— |
100 |
288.5 ± 8.3 |
0.185 ± 0.012 |
10 |
50 |
98.9 ± 1.1aA |
96.7 ± 1.2a |
292.3 ± 9.9bA |
0.188 ± 0.018cA |
60 |
92.9 ± 1.2bA |
85.8 ± 1.5b* |
302.1 ± 12.5bA |
0.186 ± 0.016cA |
70 |
84.3 ± 1.6cA |
75.4 ± 1.4c* |
319.6 ± 20.1bA |
0.193 ± 0.022bcA |
80 |
69.4 ± 1.3dA |
60.0 ± 2.1d* |
331.2 ± 18.8bB |
0.258 ± 0.028bB |
90 |
51.3 ± 1.1eA |
44.6 ± 2.2e* |
397.9 ± 24.5aB |
0.327 ± 0.031aC |
20 |
50 |
98.2 ± 1.6aA |
ND |
303.5 ± 10.2bA |
0.192 ± 0.014cA |
60 |
90.9 ± 2.9bAB |
ND |
328.5 ± 11.7bA |
0.193 ± 0.011cA |
70 |
80.1 ± 1.3cA |
ND |
342.1 ± 18.2bA |
0.213 ± 0.027cA |
80 |
62.4 ± 1.8dB |
ND |
388.3 ± 19.3abA |
0.313 ± 0.018bAB |
90 |
43.3 ± 1.5eB |
ND |
435.2 ± 22.2aB |
0.411 ± 0.026aB |
30 |
50 |
98.4 ± 1.1aA |
ND |
312.5 ± 10.2dA |
0.198 ± 0.016cA |
60 |
85.9 ± 1.9bB |
ND |
343.7 ± 10.6cdA |
0.205 ± 0.018cA |
70 |
71.1 ± 2.3cB |
ND |
366.2 ± 15.2bcA |
0.226 ± 0.021cA |
80 |
52.4 ± 2.2dC |
ND |
408.1 ± 15.3bA |
0.353 ± 0.024bA |
90 |
38.2 ± 2.2eB |
ND |
509.6 ± 26.1aA |
0.477 ± 0.033aA |
The results of a 10 min thermal treatment revealed that thermal treatment at 50 °C only resulted in a trivial loss of free curcumin (1.1%). Regarding curcumin entrapped in AOSG, a minor loss as well as a high retention (>98.0%) was observed with the treatment at 50 °C, and no significant difference in curcumin retention was found among the treatments with times of 10, 20 and 30 min. This attested to curcumin remaining stable when the heating temperature was below 50 °C. However, all the thermal treatments with a heating temperature beyond 60 °C seriously damaged the free and AOSG-entrapped curcumin. For these treatments, the curcumin loss was sharply increased with temperature. However, prolonging the treatment time from 10 min to 20 min and even 30 min did not produce significant effects on the loss of AOSG-entrapped curcumin on heating at 50 °C. For the treatments at 60 °C and 70 °C, 10 min and 20 min trials gave comparable values in curcumin retention, while the 30 min trial presented lower values than the 10 min trial. For the treatments at 80 °C, significant decreases in curcumin retention were observed by extending treatment times either from 10 min to 20 min or from 20 min to 30 min. For the treatments at 90 °C, the curcumin retention of the 10 min trial was higher than those of the 20 min and 30 min trials, but the values of the 20 min and 30 min trials were approaching each other. Even for the trial with the lowest curcumin retention, no yellowish sediment was observed with the AOSG-entrapped curcumin suspension. That means the thermal treatment did not cause significant leakage of curcumin from AOSG. Based on these facts, the following results could be obtained: (1) curcumin is sensitive to thermal treatment, especially with temperatures over 50 °C and (2) the higher temperature always led to more rapid damage of AOSG-entrapped curcumin, especially at the early stage of the thermal treatment. The present result is in line with the previously reported findings that the thermal stability of curcumin in aqueous media is temperature dependent.27,28 It was widely accepted that the thermal degradation of curcumin occurs via an autoxidative process.29 At higher temperatures, intramolecular hydrogen bonding was weakened, which led to the exposure of polar groups and hence was responsible for the dissolution of curcumin.28 Then, the dicarbonyl bridge, the most vulnerable structural site in the curcumin molecule, was attacked by O2 and mainly resulted in vanillin, vanillic acid, and protocatechuic acid as end products.30 It must be mentioned that, besides temperature, the pH of the aqueous media played a crucial role in curcumin decomposition, which became faster with increasing pH.31 In this context, the rapid degradation of curcumin observed in the present study could be partially ascribed to the neutral nature of the medium. Furthermore, the data on the treatments for 10 min revealed that AOSG-entrapped curcumin presented significantly higher retention than its free counterpart at any temperature over 60 °C. This attested that AOSG could provide protection for curcumin against thermal degradation. Similarly, a recent study confirmed that curcumin loaded in ω-methoxy poly (ethylene glycol)-b-(N-(2-benzoyloxypropyl) methacrylamide) polymeric micelles and in Triton X-100 micelles was approximately 300–500 times more stable than that in a 50
:
50 (v/v) mixture of phosphate buffer/methanol (pH = 8.0, 37 °C, 2.5 h).32 This was interpreted by the very hydrophobic nature of the micellar core, which stabilized curcumin against oxidative degradation.
To reflect the thermal stability of AOSG, the changes in its intensity-based mean size and PDI were recorded (Table 1). With the increase in treating temperature or time, both increasing tendencies in the mean particle size and PDI were observed. In detail, the treatments corresponding to the time–temperature (min–°C) combinations of 10–90, 20–90, 30–70, 30–80 and 30–90 resulted in a larger intensity-based mean size than their specific counterparts at 50 °C. When curcumin-loaded AOSG was treated at 50 °C, 60 °C or 70 °C, the extension in treating time from 10 min to 30 min had an insignificant impact on its intensity-based mean size (p > 0.05). However, substantial changes were encountered with the cases under treatment temperatures of 80 °C or 90 °C. For the effects of thermal treatment on the PDI of curcumin-loaded AOSG, similar facts were observed as with intensity-based mean size. In this regard, the swelling of AOSG was observed over thermal treatments, especially those having a higher temperature or longer treating time. Interestingly, an inconsistent and even conflicting result was obtained in our previous study that showed β-carotene-loaded AOSG shrank upon thermal treatment but had a constant PDI.16 In this scenario, the stability of a laden micelle is drug cargo specific. After a careful comparison of the two cases, the inconsistency was mainly caused by the very large difference in the nature (polarity) and retention rate of the loaded drug. First, β-carotene (13.5) has a much higher reference value for hydrophobic parameters than curcumin (3.2),33 which suggests that the core of β-carotene-loaded AOSG may be more compact than that of the curcumin-loaded one. Second, the retention values of curcumin and β-carotene upon thermal treatment differed tremendously. Although the lowest drug retentions in both systems were found with the treatment at 90 °C for 30 min, their retention rates were quite different, that is, 76.9% and 38.2%. It is widely known that the propensity for micelles to swell is related to the cohesion of the hydrophobic core.34 For drug-loaded micelles, a hydrophobic drug cargo may stabilize the micelle through additional hydrophobic interactions between the micelle core and the drug cargo.35 Accordingly, the swelling of curcumin-loaded AOSG upon thermal treatment could be first ascribed to the serious degradation of curcumin. Via this degradation, the non-polar curcumin was converted into weak polar degradation products, such as vanillin, vanillic acid, and protocatechuic acid. It is mostly likely that this degradation weakened the hydrophobic nature of the micelle core, and thus substantially decreased the hydrophobic interactions between OSG and drug molecules. The results of Yang et al. (2008) demonstrated that a higher polymer–drug hydrophobic interaction in the micelle core was eventually accompanied by a lower drug release.36 Thus, the interaction between the polymer and the drug could not only enhance the structural stability of the micelles but also prevent drug leakage from the micelles.37 As illustrated by the diagrams from DLS analysis, the thermally treated curcumin-loaded AOSG still exhibited a unimodal size distribution pattern (ESI 1†).
Freeze–thaw stability
Freezing is a common technique applied in the food industry, either to improve the shelf life or as a necessary part of processing a certain food product. Prior to consumption, the majority of frozen foods should be totally thawed. Thus, knowledge of the influence of freeze–thaw treatment on the stability of curcumin-loaded AOSG is required considering its possible incorporation in frozen foods. The data in Table 2 indicate that freeze–thaw-induced curcumin degradation and micelle swelling occurred with thermal treatment but to a much lesser extent. As mentioned above, this should be partially attributed to the milder curcumin degradation caused by freeze–thaw treatments and their lower temperatures would be responsible for this benefit. Only 4.8% of the curcumin was lost in the first freeze–thaw cycle and its total loss was determined as 17.9% upon five successive freeze–thaw cycles. As assumed, the curcumin damage would be primarily triggered by the elevated oxygen concentration in the micelle suspension upon freezing. After five freeze–thaw cycles, the intensity-based mean size of the curcumin-loaded AOSG increased from 288.5 nm to 375.8 nm, which was accompanied by an increase in the PDI from 0.185 to 0.402. As discussed previously, the impairment of freeze–thawing on the micelle's stability mainly came from the freeze side rather than the thaw side. Upon freezing, liquid water was separated from the micelle suspension as ice crystals, which certainly resulted in a higher concentration of the remaining suspension. This significantly favoured the contact of adjacent micelles and facilitated their coalescence and even merging.38 In addition, the inter- and intra-molecular hydrophobic interactions of the drug and OSG molecules were of an endothermic nature.39 Therefore, these interactions decreased upon cooling and thus definitively allowed the relaxation of compact micelles.
Table 2 The stability of curcumin loaded in the aggregates of octenylsuccinate oat β-glucan (AOSG) under varying freeze–thaw cyclesa
Freeze–thaw cycle |
Retention of curcumin (%) |
Size (nm) |
PDI |
The stability of curcumin was expressed as its retention percentage upon freeze–thaw, while the stability of OSG aggregates was expressed by the changes in the average aggregate size and its polydispersity index (PDI) upon thermal treatment. Values are expressed as mean ± standard deviation of triplicate analysis.
a–dValues bearing different superscript lowercase letters in the same column are significantly different (p < 0.05). |
— |
100 |
288.5 ± 8.3a |
0.185 ± 0.012a |
1st |
95.2 ± 3.1c |
308.5 ± 9.5b |
0.245 ± 0.022b |
2nd |
92.2 ± 2.2bc |
331.2 ± 15.6b |
0.286 ± 0.036c |
3rd |
89.1 ± 2.5b |
353.7 ± 14.2b |
0.312 ± 0.033c |
4th |
88.3 ± 2.7ab |
355.9 ± 17.2bc |
0.353 ± 0.038cd |
5th |
82.1 ± 4.1a |
375.8 ± 16.5c |
0.402 ± 0.037d |
Storage stability
The effects of temperature and light on the storage stability of curcumin-loaded AOSG were investigated (Table 3). The data on the storage at 25 °C with light exposure suggested that free curcumin degraded much more rapidly than its AOSG-entrapped counterpart. Under this circumstance, only 7.7% free curcumin remained at the end of 20-day storage, while the corresponding value of AOSG-entrapped curcumin was 54.7%. This fact strongly affirmed the protective effect of AOSG against curcumin decomposition during storage. Consistent with the present results, the complexation of curcumin with γ-cyclodextrin was demonstrated to protect curcumin from photodegradation.40 Furthermore, the encapsulation by baker's yeast (Saccharomyces cerevisiae) cells could protect curcumin against deleterious photochemical reactions during storage.41 However, the specific mechanisms underlying these facts remain unclear and are seldom discussed, which have been vaguely ascribed to the interactions of curcumin with encapsulating materials. Considering the previously evidenced fact that curcumin in organic solvents was highly sensitive to light and presented a half-life as low as 1.6 h,42 the protective effect of AOSG on curcumin may be, on the one hand, ascribed to the opacifying or filtering effect of the micelle shell. On the other hand, the AOSG entrapment possibly altered the absorption wavelength curcumin via its interactions with OSG molecules, and thus affected its photostability. It has been confirmed that a blueshift occurred with curcumin when encapsulated in glycerol monooleate-based nanoparticles and mPEG-zein micelles.43,44
Table 3 The storage stability of curcumin loaded in the aggregates of octenylsuccinate oat β-glucan (AOSG)a
Temperature/light |
Time (day) |
Retention of curcumin (%) |
Size (nm) |
PDI |
In AOSG |
Free |
The stability of curcumin was expressed as its retention percentage upon thermal treatment with free curcumin in 5% DMSO as the control, while the stability of OSG aggregates was expressed by the changes in the average aggregate size and its polydispersity index (PDI) upon thermal treatment. Values are expressed as mean ± standard deviation of triplicate analysis.
a–dValues bearing different superscript lowercase letters within the same column with the same temperature/light parameters are significantly different (p < 0.05). A–CValues bearing different superscript capital letters within the same column under the same storage are significantly different (p < 0.05). *Data in the free column bearing the superscript asterisk are significantly different from their corresponding values in the In AOSG column (p < 0.05). ND, not determined. |
— |
— |
100 |
288.5 ± 8.3 |
0.185 ± 0.012 |
25 °C/light |
5 |
84.1 ± 2.6aB |
54.1 ± 2.8a* |
273.2 ± 13.5aA |
0.189 ± 0.013aA |
10 |
70.2 ± 2.7bB |
30.2 ± 4.2b* |
249.3 ± 16.2bB |
0.226 ± 0.021aA |
15 |
61.7 ± 2.8cB |
14.7 ± 3.3c* |
220.7 ± 12.1bcB |
0.253 ± 0.022aA |
20 |
54.7 ± 3.3dC |
7.7 ± 3.9d* |
214.3 ± 15.3cB |
0.278 ± 0.019aA |
25 °C/dark |
5 |
97.3 ± 2.1aA |
ND |
298.9 ± 7.8aA |
0.198 ± 0.015aA |
10 |
92.3 ± 1.7abA |
ND |
299.2 ± 11.4aA |
0.224 ± 0.027aA |
15 |
86.2 ± 1.8bcA |
ND |
299.1 ± 12.7aA |
0.229 ± 0.018aA |
20 |
80.9 ± 2.2cA |
ND |
301.3 ± 13.2aA |
0.243 ± 0.023aA |
4 °C/dark |
5 |
97.5 ± 2.3aA |
ND |
298.8 ± 10.2aA |
0.189 ± 0.011aA |
10 |
94.2 ± 2.1abA |
ND |
297.6 ± 8.9aA |
0.199 ± 0.018aA |
15 |
91.8 ± 2.2abA |
ND |
299.4 ± 12.3aA |
0.203 ± 0.019aA |
20 |
88.9 ± 2.5bB |
ND |
302.7 ± 9.2aA |
0.226 ± 0.017aA |
For the effects of storage conditions on the intensity-based mean size and PDI of curcumin-loaded AOSG, only significant changes in the intensity-based mean size were observed for the samples stored at 25 °C with light exposure over 10 days. This indicated that micelle stability was independent of the storage temperature but relied highly on the presence or absence of light. Therefore, changes in the intensity-based mean size of curcumin-loaded AOSG during storage were certainly induced by the substantial photodegradation of curcumin. Similarly, curcumin-loaded poly lactic-co-glycolic acid nanoparticles could maintain a stable particle size up to a month when stored at 4 °C, while a significant aggregation occurred (from 158 nm to 194 nm) if they were stored at room temperature.45
In vitro stability
One of the major challenges in delivering curcumin into the colon lies in its instability and biodegradation in physiological environments. In an attempt to verify the protective function of AOSG on curcumin during its travel in the GI tract, free and AOSG-entrapped curcumin were incubated in MGF and MIF (Fig. 1), and curcumin retention was determined against incubation times. Both free and AOSG-entrapped curcumin exhibited excellent stability in MGF for up to 90 min of incubation at 37 °C (Fig. 1A). No significant loss was observed with free curcumin, while a significant but very limited (2.7%) loss occurred with AOSG-entrapped curcumin. We speculate this difference was related to the pH sensitivity of curcumin and the permeability of H+ across the corona of the micelles. Usually, curcumin degrades via a two-step process: deprotonation and autoxidation.46 The rate of curcumin degradation increases with the pH of the medium owing to the fact that curcumin is liable to be deprotonated from its hydroxyl groups under alkaline conditions.32 In this regard, the diffusion rate of H+ into the core of the micelles is the key factor determining the stability of loaded curcumin and it was highly controlled by the nature of the micelle corona. First, the high viscosity of the micelle corona certainly retarded the diffusion of H+ into the micelle core. Second, assuming that the pKa value of the carboxyl groups in OSG approaches that of OS-starch (4.8),47 the carboxyl groups of OSG molecules in the micelle core before being mixed with MGF were totally deprotonated (–COO−). Therefore, once they are mixed with MGF, the deprotonated carboxyl groups could neutralize the early comers of H+ and stabilize the pH of the micelle core. In this scenario, the AOSG-entrapped curcumin stayed longer at a neutral pH than free curcumin and underwent more serious deprotonation, which lasted until the end of incubation to initiate autoxidation.
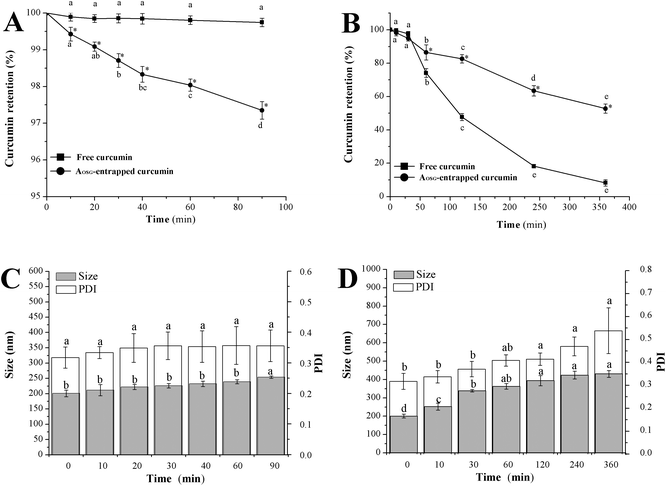 |
| Fig. 1 Curcumin and the aggregate stability of curcumin-loaded octenylsuccinate oat β-glucan aggregates after incubation in mimetic gastric fluid (A, C) and mimetic intestinal fluid (B, D). | |
Regarding the curcumin retention in MIF, both free and AOSG-entrapped curcumin underwent tremendous decreases. At the end of an incubation in MIF for up to 360 min, the loss of free and AOSG-entrapped curcumin reached 91.8% and 53.0%, respectively. These values were much higher than the corresponding ones in MGF. The present results of free curcumin were in line with the findings of Mohanty et al. (2010) in that free curcumin incubated in PBS (0.01 M, pH = 7.4) underwent rapid degradation and only 6% remained intact upon 6 h of incubation.43 On the basis of these data, it was concluded that: (1) both free and AOSG-entrapped curcumin degraded at much higher rates in MIF than in MGF, and (2) the entrapment in AOSG could retard the degradation of curcumin in MIF. According to the pH sensitivity of curcumin, the first conclusion could be ascribed to the higher pH of MIF (7.2) than that of MGF (1.2). Curcumin entrapped in the nanoparticles of glycerol monooleate, pluronic F-127, zein and soy protein also showed better stability in MGF than in MIF.43,48,49 For the second conclusion, similar results have been reported that in pH 7.4 PBS containing 10% (v/v) methanol, the stability of curcumin was enhanced approximately 6-fold when encapsulated in mPEG-zein micelles.44 The protective effects of AOSG entrapment on curcumin against the degradation in MGF could be interpreted in the following ways. First, the early permeated OH− in the micellar core was neutralized by the deprotonation of carboxyl groups of OSG via the reaction –COOH + OH− = –COO− + H2O. This certainly delayed the deprotonation of hydroxyl groups of curcumin and hence protected it from degradation. Second, curcumin molecules entrapped in AOSG were tightly surrounded by the hydrophobic moieties of OSG, and therefore were protected from direct contact with reactive substances, such as OH−.50
Formulations designed for an oral delivery system should resist pH changes and digestive enzymolysis and not undergo grievous physical aggregations of the delivery particles themselves in the GI tract.41 Obviously, the incubation in MGF at 37 °C for 60 min did not bring about any significant changes in the mean particle size of curcumin-loaded AOSG (Fig. 1C). However, upon incubation in MIF at 37 °C for 6 h, the intensity-based mean size (PDI) of curcumin-loaded AOSG significantly increased from 200.8 nm (0.317) to 430.8 nm (0.538). Fortunately, no aggregation or flocculation of curcumin-loaded AOSG was visibly observed upon the incubations both in MGF and MIF. Inconsistent with the present results, EGCG-loaded nanoparticles of the ovalbumin–dextran conjugate showed stable particle sizes in MGF or SIF at 37 °C for 2 h.51 In this regard, the stability of delivery particles in digestive fluids differed from case to case. In the present study, the significant swelling of curcumin-loaded AOSG upon the incubation in MIF may be related to the substantial degradation of curcumin.
In vitro release profile
The release of curcumin from AOSG in MGF and MIF is shown in Fig. 2A and B, respectively. Upon incubating in MGF for 90 min and in MIF for 6 h, only 1.1% and 7.6% of the loaded curcumin were released from AOSG, respectively. Regarding the profile of these releasing curves, a slow but sustained release persisted over the whole incubation either in MGF or in MIF. This was not in agreement with the previous studies. Usually, a biphasic release pattern was concluded, in which a burst release was followed by a slow and sustained release.43,52,53 The initial stage was due to the discharge of the surface-bound drug located in the micelle corona, while the latter stage corresponded to the partitioning of the drug present in the micelle core and it subsequently escaped from the micelle surface into the digestive fluids.54 In this view, it was speculated that only a negligible amount of curcumin was bound in the corona of AOSG due to its poor compatibility with the hydrophilic skeleton of OSG molecules.
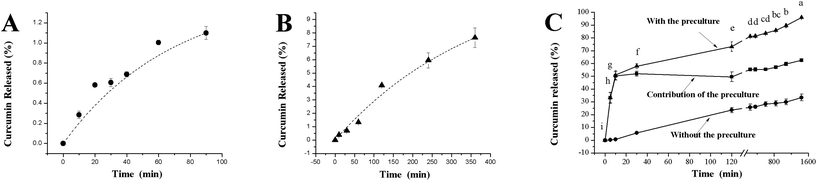 |
| Fig. 2
In vitro release profiles of curcumin from curcumin-loaded octenylsuccinate oat β-glucan aggregates in mimetic gastric fluid (A), mimetic intestinal fluid (B), and mimetic colonic fluid (C). | |
In contrast to the situations in MGF and MIF, a different pattern was observed for incubation in MCF (Fig. 2C, with the preculture), which consisted of a burst release up to 50.97% in the first 10 min of the incubation and a slow but sustained release in the remaining time. To clarify the underlying mechanism of this pattern, the release rate without the preculture was determined. Then, the contribution of the colonic flora was obtained by comparing the rates with and without the preculture. Without the preculture, a slow release occurred and the amount of released curcumin increased linearly with incubation times. In this case, only up to 33.42% curcumin was released at the end of incubation in MCF for 24 h, which was much less than that with the preculture (95.98%). In summary, the early burst release of curcumin in MCF was dominantly triggered by the preculture; i.e., gut flora. This was absolutely ascribed to the high fermentability of oat β-glucan by the colonic flora.13 The flora fermentation rapidly hydrolysed and broke down the skeleton of β-glucan, leading to a loss in micelle integrity. However, the following sustained release attested to AOSG not being fully disintegrated. Usually, octenylsuccinate starch could be divided into rapidly digestible, slowly digestible and resistant fractions according to its enzymatic hydrolysis profile.55 Enlightened by this, it was speculated that OSG consists of rapidly fermentable, slowly fermentable and resistant fractions. With this assumption, the early burst release was brought about by the degradation of the rapidly fermentable OSG fraction, while the latter sustained release was supported by its slowly fermentable and resistant fractions. Combined with the release profiles of curcumin from AOSG in MGF, MIF and MCF, it can be concluded that AOSG was capable of delivering curcumin into the colon via oral administration.
In vivo absorption
After the oral administration of free and AOSG-entrapped curcumin, the plasma curcumin concentration of rats was checked, and the results are shown in Fig. 3. The peak concentration (Cmax) and its corresponding time (Tmax) were identified directly from the obtained curves. The area under the curve (integral) from 30 min to 300 min (AUC30–300) was calculated using Origin 9.0. The preliminary experiments revealed that, for free curcumin, a high dosage up to 250 mg per kg bw is a prerequisite for quantifying the curcumin in the blood. A previous report showed that, after a single oral dose of 10 g or 12 g of curcumin, the free form of curcumin was barely detected in human plasma.56 The corresponding administration dosage of curcumin-loaded AOSG was set as 200 mg per kg bw. With these dosages, the actual average intakes of curcumin for each rat in the free and entrapped curcumin groups were 62.5 mg and 0.42 mg, respectively. Even though the actual intake of the free curcumin was much higher than that of the entrapped one, the blood concentration–time curve of the free curcumin was mainly located under the curve of the entrapped one. Their corresponding values of Cmax were 208.9 μg mL−1 and 292.7 μg mL−1. Interestingly, both the Cmax values for free and entrapped curcumin were observed at 30 min after the oral administration. This may suggest that curcumin was mainly absorbed in the colon regardless of its form. Despite the large difference between the intakes of free (62.5 mg) and entrapped (0.42 mg) curcumin, the AUC30–300 value of entrapped curcumin (32
678.5 ng min mL−1) was much greater than that of free curcumin (24
148.1 ng min mL−1). Taken together, these results validated that AOSG entrapment could substantially improve the bioavailability of curcumin. This fact can be interpreted in different ways. First, as indicated above, AOSG could protect curcumin against harsh environs in the GI tract. The loading of curcumin into the hydrophobic cavity of AOSG certainly reduces its exposure to bacteria, as well as enzymatic degradation occurring in the GI tract. This finding preserves the active structural form of curcumin until its absorption. Second, the size of curcumin-loaded AOSG (approximately 200 nm) may allow for its efficient uptake in the lymphoid sections of the intestine.57 Finally, the mucoadhesive characteristic of β-glucan triggers the adhesion of entrapped curcumin onto the mucosal layer of the GI tract, which is helpful for the absorption of curcumin.58
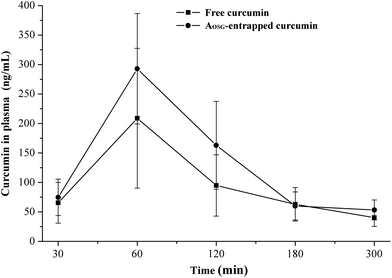 |
| Fig. 3 Blood concentration–time profiles of curcumin after oral administration to rats: free curcumin (■, 250 mg per kg bw) and curcumin-loaded octenylsuccinate oat β-glucan aggregates (●, 200 mg per kg bw, equivalent to 1.6 mg per kg bw curcumin). | |
In summary, AOSG can effectively protect curcumin against degradation in the upper digestive tract. The entrapped curcumin was released at rather slow rates from AOSG both in MGF and MIF, while it was released rapidly in MCF. This finding validates that AOSG is potentially suitable in colon-targeting delivery. More importantly, the results of the present study demonstrated the feasibility of improving the bioavailability of curcumin by AOSG entrapment. The results obtained in this study will be helpful in the design and development of new strategies for improving the stability of BCs both in food processing and in the human digestive tract.
Conflicts of interest
There are no conflicts to declare.
Acknowledgements
We gratefully acknowledge the financial supports provided by the National Key R&D Program of China (2016YFD0400204-2) and the National Natural Science Foundation of China (31771932).
References
- A. Tenesa and M. G. Dunlop, Nat. Rev. Genet., 2009, 10, 353–358 CrossRef CAS PubMed
.
- J. Terzic, S. Grivennikov, E. Karin and M. Karin, Gastroenterology, 2010, 138, 2101–2114 CrossRef CAS PubMed
.
- W. V. D. Ende, D. Peshev and L. D. Gara, Trends Food Sci. Technol., 2011, 22, 689–697 CrossRef
.
- W. M. Grady, Cancer Metastasis Rev., 2004, 23, 11–27 CrossRef CAS PubMed
.
- R. H. Liu, Am. J. Clin. Nutr., 2003, 78, 517S–520S CAS
.
- J. Sun, Y. F. Chu, X. Wu and R. H. Liu, J. Agric. Food Chem., 2002, 50, 7449–7454 CrossRef CAS PubMed
.
- L. Hooper and A. Cassidy, J. Sci. Food Agric., 2006, 86, 1805–1813 CrossRef CAS
.
- S. L. Kosaraju, Crit. Rev. Food Sci. Nutr., 2005, 45, 251–258 CrossRef CAS PubMed
.
- R. Liang, C. F. Shoemaker, X. Q. Yang, F. Zhong and Q. R. Huang, J. Agric. Food Chem., 2013, 61, 1249–1257 CrossRef CAS PubMed
.
- A. Mensi, P. Borel, A. Goncalves, M. Nowicki, B. Gleize, S. Roi, J. Chobert, T. Haertlé and E. Reboul, J. Agric. Food Chem., 2014, 62, 5916–5924 CrossRef CAS PubMed
.
- K. Oehlke, M. Adamiuk, D. Behsnilian, V. Graf, E. Mayer-Miebach, E. Walz and R. Greiner, Food Funct., 2014, 5, 1341–1359 CAS
.
- N. Bordenave, B. R. Hamaker and M. G. Ferruzzi, Food Funct., 2014, 5, 18–34 CAS
.
- C. Daou and H. Zhang, Compr. Rev. Food Sci. Food Saf., 2012, 11, 355–365 CrossRef CAS
.
- J. Liu, F. Chen, W. N. Tian, Y. Q. Ma, J. Li and G. Zhao, J. Agric. Food Chem., 2014, 62, 7532–7540 CrossRef CAS PubMed
.
- J. Liu, J. Li, Y. Q. Ma, F. Chen and G. Zhao, J. Agric. Food Chem., 2013, 61, 12683–12691 CrossRef CAS PubMed
.
- Y. Ma, J. Liu, F. Ye and G. Zhao, LWT–Food Sci. Technol., 2016, 65, 845–851 CrossRef CAS
.
- N. P. Aditya, M. Shim, I. Lee, Y. Lee, M. H. Im and S. Ko, J. Agric. Food Chem., 2013, 61, 1878–1883 CrossRef CAS PubMed
.
- Z. Teng, Y. Li and Q. Wang, J. Agric. Food Chem., 2014, 62, 8837–8847 CrossRef CAS PubMed
.
- A. Maltais, G. E. Remondetto and M. Subirade, Food Hydrocolloids, 2009, 23, 1647–1653 CrossRef CAS
.
- J. L. Hu, S. P. Nie, C. Li, Z. H. Fu and M. Y. Xie, J. Agric. Food Chem., 2013, 61, 6092–6101 CrossRef CAS PubMed
.
- M. Gou, K. Men, H. Shi, M. Xiang, J. Zhang, J. Song, J. Long, Y. Wan, F. Luo, X. Zhao and Z. Qian, Nanoscale, 2011, 3, 1558–1567 RSC
.
- M. Sun, L. Zhao, C. Guo, F. Cao, H. Chen, L. Zhao, Q. Tan, X. Zhu, F. Zhu, T. Ding, Y. Zhai and G. Zhai, J. Nanopart. Res., 2012, 14(2), 1–13 Search PubMed
.
- L. Hu, Y. Jia, F. Niu, Z. Jia, X. Yang and K. Jiao, J. Agric. Food Chem., 2012, 60, 7137–7141 CrossRef CAS PubMed
.
- G. K. Jayaprakasha, L. J. M. Rao and K. K. Sakariah, J. Agric. Food Chem., 2002, 50, 3668–3672 CrossRef CAS PubMed
.
- M. Esmaili, S. M. Ghaffari, Z. Moosavi-Movahedi, M. S. Atri, A. Sharifizadeh and M. Farhadi, LWT–Food Sci. Technol., 2011, 44, 2166–2172 CrossRef CAS
.
-
A. Noomhorm, I. Ahmad and A. K. Anal, Functional foods and dietary supplements: Processing effects and health benefits (Chapter 11), Wiley-Blackwell, 2014 Search PubMed
.
- N. A. Siddiqui and J. Pak, Pharm. Sci., 2015, 28(1Suppl), 299–305 CAS
.
- R. Jagannathan, P. M. Abraham and P. Poddar, J. Phys. Chem. B, 2012, 116(50), 14533–14540 CrossRef CAS PubMed
.
- C. Schneider, O. N. Gordon, R. L. Edwards and P. B. Luis, J. Agric. Food Chem., 2015, 63, 7606–7614 CrossRef CAS PubMed
.
- T. Esatbeyoglu, K. Ulbrich, C. Rehberg, S. Rohn and G. Rimbach, Food Funct., 2015, 6, 887–893 CAS
.
- S. Mondal, S. Ghosh and S. P. Moulik, Soft Mater., 2015, 13, 118–125 CrossRef CAS
.
- O. Naksuriya, M. J. van Steenbergen, J. S. Torano, S. Okonogi and W. E. Hennink, AAPS J., 2016, 18, 777–787 CrossRef CAS PubMed
.
-
https: //pubchem.ncbi.nlm.nih.gov/
.
- S. C. Owen, D. P. Y. Chan and M. S. Shoichet, Nano Today, 2012, 7, 53–65 CrossRef CAS
.
- J. Y. Lee, E. C. Cho and K. Cho, J. Controlled Release, 2004, 94, 323–335 CrossRef CAS PubMed
.
- X. Yang, B. Zhu, T. Dong, P. Pan, X. Shuai and Y. Inoue, Macromol. Biosci., 2008, 8, 1116–1125 CrossRef CAS PubMed
.
- W. Zhou, C. Li, Z. Wang, W. Zhang and J. Liu, J. Nanopart. Res., 2016, 18, 275 CrossRef
.
- J. O'Regan and D. M. Mulvihill, Food Chem., 2010, 119, 182–190 CrossRef
.
- G. G. Palazolo, P. A. Sobral and J. R. Wagner, Food Hydrocolloids, 2011, 25, 398–409 CrossRef CAS
.
- M. L. R. del Castillo, E. López-Tobar, S. Sanchez-Cortes, G. Flores and G. P. Blanch, Vib. Spectrosc., 2015, 81, 106–111 CrossRef
.
- E. I. Paramera, S. J. Konteles and V. T. Karathanos, Food Chem., 2011, 125, 913–922 CrossRef CAS
.
- H. H. Tønnesen, J. Karlsen and G. B. van Henegouwen, Z. Lebensm.–Unters. Forsch., 1986, 183, 116–122 CrossRef
.
- C. Mohanty and S. K. Sahoo, Biomaterials, 2010, 31, 6597–6611 CrossRef CAS PubMed
.
- S. Podaralla, R. Averineni, M. Alqahtani and O. Perumal, Mol. Pharmaceutics, 2012, 9, 2778–2786 CrossRef CAS PubMed
.
- Y. M. Tsai, W. C. Jan, C. F. Chien, W. C. Lee, L. C. Lin and T. H. Tsai, Food Chem., 2011, 127, 918–925 CrossRef CAS PubMed
.
- C. Schneider, O. N. Gordon, R. L. Edwards and P. B. Luis, J. Agric. Food Chem., 2015, 63, 7606–7614 CrossRef CAS PubMed
.
- D. Lei, J. Liu, F. Ye, F. Chen and G. Zhao, Food Hydrocolloids, 2014, 41, 250–256 CrossRef CAS
.
- A. Patel, Y. Hu, J. K. Tiwari and K. P. Velikov, Soft Mater., 2010, 6, 6192–6199 RSC
.
- A. Tapal and P. K. Tiku, Food Chem., 2012, 13, 960–965 CrossRef
.
- B. Zheng, Z. Zhang, F. Chen, X. Luo and D. J. McClements, Food Hydrocolloids, 2017, 71, 187–197 CrossRef CAS
.
- Z. Li and L. Gu, J. Agric. Food Chem., 2014, 62, 1301–1309 CrossRef CAS PubMed
.
- W. J. Trickler, A. A. Nagvekar and A. K. Dash, AAPS PharmSciTech, 2008, 9(2), 486–493 CrossRef CAS PubMed
.
- R. Misra, S. Acharya, F. Dilnawaz and S. K. Sahoo, Nanomedicine, 2009, 4(5), 519–530 CrossRef CAS PubMed
.
- J. Shaikh, D. D. Ankola, V. Beniwal, D. Singh and M. N. Kumar, Eur. J. Pharm. Sci., 2009, 37(3–4), 223–230 CrossRef CAS PubMed
.
- J. He, J. Liu and G. Zhang, Biomacromolecules, 2008, 9, 175–184 CrossRef CAS PubMed
.
- S. K. Vareed, M. Kakarala and M. T. Ruffin, Cancer Epidemiol. Biomarkers Prev., 2008, 17(6), 1411–1417 CrossRef CAS PubMed
.
- D. D. Stuart and T. M. Allen, Biochim. Biophys. Acta, 2000, 146, 3219–3229 Search PubMed
.
- H. Zhang, Y. Ma and X. Sun, Med. Res. Rev., 2009, 30, 270–289 Search PubMed
.
Footnote |
† Electronic supplementary information (ESI) available. See DOI: 10.1039/c7fo01569k |
|
This journal is © The Royal Society of Chemistry 2018 |