DOI:
10.1039/C7BM00811B
(Paper)
Biomater. Sci., 2018,
6, 179-188
Coordination-driven assembly of catechol-modified chitosan for the kidney-specific delivery of salvianolic acid B to treat renal fibrosis†
Received
6th September 2017
, Accepted 9th November 2017
First published on 9th November 2017
Abstract
Kidney-specific delivery is critically important for the treatment of renal fibrosis with drugs such as salvianolic acid B (Sal B). Here we report a kidney-specific nanocomplex formed by the coordination-driven assembly of catechol-modified low molecular weight chitosan (HCA-Chi), calcium ions and Sal B. The prepared HCA-Chi-Ca-Sal B (HChi-Ca-Sal B) nanocomplex reversed the TGF-β1-induced epithelial–mesenchymal transition (EMT) in HK-2 cells. In vivo imaging demonstrated a kidney-specific biodistribution of the nanocomplex. The anti-fibrosis effect of HChi-Ca-Sal B was tested in a mouse model of unilateral ureteral obstruction (UUO). Significant attenuation of the morphological lesions and the levels of extracellular matrix (ECM) proteins in the tubulointerstitium was observed in mice treated with HChi-Ca-Sal B, suggesting that the nanocomplex was able to prevent fibrosis better than the treatment with free Sal B. It was concluded that the HChi-Ca-Sal B nanocomplex showed a specific renal targeting capacity and could be utilized to enhance Sal B delivery for treating renal fibrosis.
Introduction
Renal fibrosis is a common manifestation of progressive renal diseases, leading to the functional deterioration and eventual loss of renal function, irrespective of the nature of the initial renal injury.1,2 Epithelial–mesenchymal transition (EMT), accumulation of extracellular matrix (ECM) and inflammatory stimulation all contribute to renal fibrosis.3–5 Ineffective treatment of renal fibrosis can lead to end-stage renal failure, a devastating disorder which ultimately necessitates dialysis or kidney transplantation.6 Unfortunately, there is no effective method to directly treat or reverse renal fibrosis.7 Several active ingredients from traditional Chinese herbs have recently shown positive effects in treating kidney fibrosis.8 Salvianolic acid B (Sal B, Fig. S1†) is a major water-soluble component extracted from the danshen plant. Sal B is well recognized as an anti-oxidative agent and a free radical scavenger which is involved in the protection of various cells.9–11 It has been reported that Sal B can prevent TGF-β1 signaling, which induces tubular EMT in vivo.12,13 However, its non-specific biodistribution in vivo greatly limits the potential of Sal B to be used as an anti-fibrosis agent. To our knowledge, few effective carriers have yet been developed for Sal B delivery. Thus, a satisfactory drug delivery system is urgently needed for the kidney-targeted transportation of Sal B to achieve the successful treatment of renal fibrosis.
Anatomical barriers between the circulation and the proximal tubular cells, such as glomerular filtration barriers, can limit the penetration of carriers into the renal tissue.14 Carriers with a relatively low molecular weight, such as low molecular weight proteins, folate conjugates and polymeric carriers, have been used as drug carriers for kidney targeting.15–19 For the preparation of drug–carrier conjugates, the commonly applied approach is to couple the drug covalently to the side chain groups on the carrier. However, this might lead to the risk of drug inactivation and inefficient drug release.20 Recently, a novel approach has been described, namely the linking of drugs to carriers via coordination chemistry. The functionality and flexibility of carriers based on metal–ligand bonding have opened up new perspectives in a variety of fields including smart drug delivery.21 Carriers based on coordination complexes have many properties that make them suitable for drug delivery such as high stability under physiological conditions and smart release due to the pH-responsive ability.22–25 Furthermore, compared with covalent polymer–drug conjugates, coordination assemblies are capable of minimizing incomplete drug release and reducing cumulative drug toxicity.
As shown in Fig. S1,† there are multiple phenolic hydroxyl and carboxyl groups in the structure of Sal B, suggesting that it has the capability to coordinate metal ions. Inspired by this, we developed a kidney-specific delivery system for Sal B transportation based on coordinate bonding. Low molecular weight chitosan (LMWC), which has been previously shown to have kidney-specific targeting ability, is a superior delivery carrier for renal fibrosis treatment.20,26 Furthermore, it has been reported that the catechol group in the hydrocaffeic acid (HCA) structure possess the property of coordination with metal ions.27,28 We therefore conjugated LMWC with HCA to prepare the carrier HCA-Chi. As shown in Fig. 1, the coordination nanocomplex (HChi-metal-Sal B) was prepared through a two-step solvothermal method under a pH gradient. We predicted that the ternary nanocomplex would be stable in the blood circulation (pH 7.4) and would specifically target renal tissues. Once endocytosed into epithelial cells, the nanocomplex would be partially disrupted and Sal B would be released in lysosomes (pH 4.5–5.0) as a result of the pH-stimulated cleavage of the coordinate bond. The physicochemical properties, biodistribution and anti-fibrosis activity of the nanocomplexes in vitro and in vivo were studied. The results suggested that the coordination nanocomplex was a promising drug delivery system for the improved treatment of renal fibrosis with Sal B.
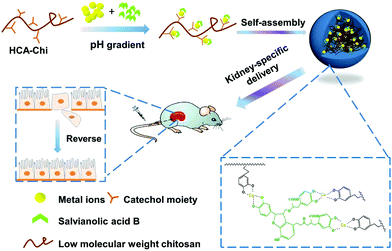 |
| Fig. 1 Schematic diagram of the coordination-driven assembly of catechol-modified chitosan for the kidney-specific delivery of salvianolic acid B. | |
Methods
Materials
Low molecular weight chitosan (LMWC) with a number-average molecular weight (Mn) of 5000 (85% deacetylated) was purchased from Jinan Haidebei Marine Bioengineering Company (Jinan, China). Hydrocaffeic acid (HCA) was purchased from Alfa Aesar (Shanghai, China). N-Hydroxysuccinimide (NHS) and 1-ethyl-3(3-dimethylaminopropyl)carbodiimide (EDC) were purchased from Aladdin Reagent Database Inc. (Shanghai, China). Salvianolic acid B (Sal B) was provided by Nanjing Zelang Pharmaceuticals Inc. (Nanjing, China). Calcium chloride, copper nitrate, and ferric chloride were provided by Sinopharm Chemical Reagent Co., Ltd (Shanghai, China). Recombinant Human TGF-β1 was purchased from PeproTech (USA).
Coordination interaction between metal ions and ligands
The formation, cleavage, and pH dependence of the nanocomplex systems formed between the ligands (Sal B or HCA-Chi) and metal ions were studied by UV-Vis spectroscopy. Briefly, mixtures of ligands and metal salts were prepared by adding an equal volume of a metal salt (ferric chloride, copper nitrate, or calcium chloride) in distilled water to Sal B or HCA-Chi solution in water under stirring until the equilibrium was reached. The real-time UV-Vis spectra of the resultant mixtures were recorded at different pH values. The pH was adjusted with dilute HCl or NaOH.
Preparation and characterization of HChi-Ca-Sal B
The HChi-Ca-Sal B nanocomplex was prepared by using a two-step solvothermal method. In brief, 0.2 ml Sal B solution (2 mg ml−1) was diluted to 3 ml by the addition of distilled water; then calcium chloride in distilled water was added dropwise to the Sal B solution, followed by pH adjustment to 6 using 0.1 M NaOH. The solution was incubated at 37 °C for 1 h under continuous agitation. The mixture was then added to the HCA-Chi solution (2 mg ml−1), adjusted to pH 6.6 using dilute NaOH and incubated at 37 °C for 2 h to spontaneously form the HChi-Ca-Sal B nanocomplex. The resulting mixture was purified by dialysis against deionized water for 12 h to remove free Sal B. The Sal B content in the prepared nanocomplex was measured by using the HPLC assay. The result showed that the loading efficiency was 6.16% in this nanocomplex system.
The size distribution of the nanocomplex was measured using a dynamic light scattering (DLS) system (Brookhaven BI9000AT, Brookhaven Instruments Co., USA) and the morphology was observed by transmission electron microscopy (TEM, Hitachi H-7650, Japan). For elemental analysis and mapping, energy-dispersive spectroscopy (EDS) was performed at 200 kV with nitrogen as a marker for location. The structural characteristics of the nanocomplex were also determined by WAXD spectroscopy.
In vitro drug release and stability of the HChi-Ca-Sal B nanocomplex
Drug release from the nanocomplex was analyzed at pH 7.4, 6.8 and 5.0 using the dialysis method. Lyophilized HChi-Ca-Sal B powder containing 0.16 mg Sal B was reconstituted in 2 ml dissolution solution, placed in a 3500 MWCO dialysis bag, immersed in 50 ml release medium and gently shaken at 100 rpm in a water bath (37.0 ± 0.5 °C). At predetermined intervals, 1 ml dissolution sample was collected and the concentration of Sal B was measured by HPLC. To study the nanocomplex stability, lyophilized HChi-Ca-Sal B was suspended in 10 mM PBS containing 140 mM NaCl (pH 7.4), and incubated at 37 °C. At predetermined intervals, 2 ml solution was collected for the measurement of the size and polydispersity index (PDI) by DLS. In addition, the size distribution of the HCA-Chi solution and acidized HChi-Ca-Sal B nanocomplex (pH 5.0) was also determined by DLS.
Reversal of TGF-β1-induced EMT of HK-2 cells by Sal B and HChi-Ca-Sal B
1 × 104 HK-2 cells were seeded in 6-well plates. For the experiments, cells were divided into four groups as follows: a control group, a TGF-β1 (10 ng ml−1)-induced EMT control group, a group in which the cells were exposed to both TGF-β1 (10 ng ml−1) and free Sal B (0.1, 1, 10 and 100 mmol l−1), and a group in which the cells were exposed to both TGF-β1 (10 ng mL−1) and HChi-Ca-Sal B (at a Sal B dose of 0.1, 1, 10 and 100 μmol l−1). HK-2 cells were cultured for 24 h after seeding in DMEM/F12 + 10% FBS, then changed to fresh culture medium containing TGF-β1. After 72 h, the media were changed to fresh DMEM/F12 containing TGF-β1 plus free Sal B or HChi-Ca-Sal B at the different concentrations described above for a further 72 h. The positive control cells were treated with 10% FBS + TGF-β1 and the negative control cells were treated with 10% FBS. The morphology of HK-2 cells in each group was examined using an inverted microscope (EVOS, Thermo Fisher, USA). Length/width ratios were used to quantify morphological changes. For each group, 80 cells were measured using the ImageJ software. The levels of proteins were determined by western blot analysis.
In vivo fluorescence imaging analysis
To evaluate the in vivo biodistribution and kidney targeting efficacy of HChi-Ca-Sal B, the nanocomplex was prepared using Cy7-SE-labeled HCA-Chi. Male ICR mice were intravenously administered with 10 mg kg−1 (Cy7 dose) of Cy7-SE-labeled HChi-Ca-Sal B and sacrificed at 1, 2, 4, 8, 12 and 24 h after injection. Tissues were exposed to a Kodak FX PRO imaging system (Rochester, NY, USA) with excitation at 720 nm and emission at 790 nm. Major organs were also isolated and imaged. Cy7-SE was dissolved in DMSO and diluted with distilled water. Free Cy7 solution (10 mg kg−1) was also administered as a control, and the animals were sacrificed 8 h after injection. Major organs were also isolated and imaged.
Pharmacodynamics in the unilateral ureter obstruction (UUO) model
The UUO procedure was performed in ICR mice weighing 22 ± 2 g, which were purchased from the Laboratory Animal Center at Yangzhou University. All animal study protocols were designed in accordance with guidelines set by the National Institute of Health Guide for the Care and Use of Laboratory Animals, and approved by the Animal Ethical Experimentation Committee of China Pharmaceutical University. Mice were anesthetized with chloral hydrate (400 mg kg−1, i.p.), and were handled under aseptic conditions during the entire surgical procedure. Briefly, the abdomen was opened and the left ureter was located and occluded with 5-0 silk. The abdomen was then sutured. After the operation, mice were returned to holding cages for recovery from anesthesia. Mice were randomly divided into four groups: the sham group (which underwent identical surgical procedures except that the ureter was not ligated, n = 5), the untreated UUO group (n = 5), the UUO group treated with the Sal B solution (at a Sal B dose of 10 mg per kg per day, n = 5), and the UUO group treated with the HChi-Ca-Sal B nanocomplex (at a Sal B dose of 10 mg per kg per day, n = 5). In the two Sal B treatment groups, mice were administered intravenously with the corresponding drug preparation starting 2 days before the induction of UUO until day 7 after the surgery once a day. Untreated UUO mice were given a daily intravenous injection of normal saline. Mice were sacrificed on the 8th day after the surgery; then the obstructed kidney and serum were harvested to evaluate the anti-fibrotic activity of Sal B and HChi-Ca-Sal B. Part of the kidney from each mouse was used for pathological analysis. Hydroxyproline (Hyp) content was determined using a Hyp assay kit and malondialdehyde (MDA) content in plasma was detected using a lipid peroxidation MDA assay kit. All procedures were performed according to the kit methods.
Histology and immunohistochemistry
Histological studies were performed in mice subjected to UUO, and sham-operated controls. After the mice were sacrificed, the obstructed kidney was removed, halved longitudinally and fixed in 4% buffered formalin for 24 h. Subsequently, the kidneys were dehydrated in an ascending ethanol series, cleared in xylene and embedded in paraffin. Kidney sections (3 μm) were stained with hematoxylin–eosin (H&E), Masson's trichrome and Periodic acid-Schiff stain (PAS) for light microscopy analysis.
For immunostaining, paraffin sections were first boiled in 10 mM Na-citrate solution (pH 6.0) for 10 min to retrieve antigens, and then stained with antibodies against TGF-β1, α-SMA and fibronectin for light microscopy analysis.
Statistical analysis
All experiments were performed at least three times. The data are expressed as mean ± standard deviation (SD). Statistical analysis was performed using the Sigma Stat software (Jandel Scientific Software, San Rafael, CA, USA). Comparisons between the groups were made using one-way ANOVA, followed by Student's t-test. Differences were considered to be statistically significant at p < 0.05.
Results
Synthesis and characterization of HCA-Chi
The 1H NMR spectrum of HCA-Chi contained a proton signal at 6.76 ppm, which belongs to the aromatic ring proton of hydrocaffeic acid (Fig. S2†). This confirms the success of the coupling reaction. The calculated degree of catechol substitution in the chitosan backbone was 5.55% (by 1H NMR, as the ratio to sugar units) and 6.88% (by UV-vis spectroscopy).
Coordination interaction between metal ions and ligands
As demonstrated in Fig. 2, when the pH increased, the intrinsic absorbance of the complex increased remarkably, which was evidence of the interaction between metals and ligands, and the formation of metal complexes. In details, a lower and broader pH-responsive range was observed in the presence of Fe3+ (3.4–7.4), and a narrower responsive range was found in complexes formed by Cu2+ and Ca2+ (4.5–7.0).
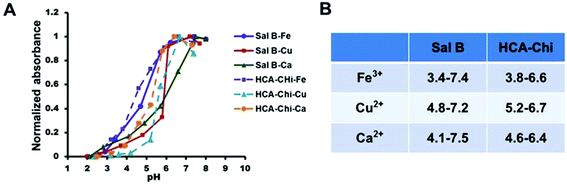 |
| Fig. 2 (A) UV-Vis spectra of various coordination complexes under different pH conditions. (B) Summary of the pH-responsive onsets for the coordination of metals and ligands. | |
Characterization of the HChi-Ca-Sal B ternary nanocomplex
TEM images of HChi-Ca-Sal B showed well-dispersed, spherical nanocomplexes (Fig. 3A and B). The average size was 66.8 ± 0.5 nm as determined by DLS. The existence of Ca2+ in the HChi-Ca-Sal B nanocomplex was confirmed via elemental mapping using field-emission SEM and EDS (Fig. 3C). The results showed that bright red dots (calcium) were dispersed throughout the entire sample region, indicating the uniform dispersion of Ca2+ in the nanocomplex particles. WAXD analysis was carried out to confirm the morphology of Sal B in the nanocomplex (Fig. S3†). It was evident that calcium chloride and Sal B showed significant crystal peaks whereas the blank HCA-Chi and the nanocomplex had no detectable peaks.
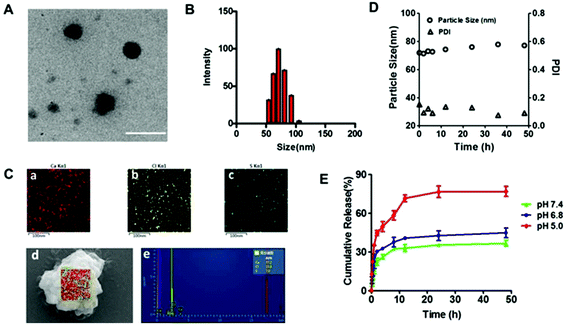 |
| Fig. 3 (A) TEM images of HChi-Ca-Sal B complexes. Scale bar is 100 nm. (B) Size distribution of HChi-Ca-Sal B complexes detected by DLS. (C) EDS mapping (a–d) and EDS area scanning profiling (e) of HChi-Ca-Sal B complexes. (D) In vitro release profiles of HChi-Ca-Sal B in PBS under different pH conditions. (E) Real-time changes in the diameter and PDI of HChi-Ca-Sal B under physiological conditions. | |
In vitro drug release and stability of the HChi-Ca-Sal B nanocomplex
The in vitro release profile of Sal B from the HChi-Ca-Sal B nanocomplex is shown in Fig. 3D. At pH 7.4, approximately 26% of Sal B was released within the first 4 h, and about 10% more was released after 48 h. In contrast, the release of Sal B was accelerated under acidic conditions; more than 45% of Sal B was released in the first 4 h at pH 5.0, and nearly 80% was released after 48 h. In addition, the stability of the nanocomplex was also determined (Fig. 3E). The particle size and PDI hardly changed at the normal physiological pH of 7.4, while at pH 5.0 (like the pH value found in lysosomes), the particle size increased to nearly 500 nm (Fig. S4†).
Cytotoxicity and uptake of the HChi-Ca-Sal B nanocomplex
The cytotoxicity of HChi-Ca-Sal B was determined by using the MTT assay in the commonly used HK-2 renal cell line, which is derived from human proximal tubular cells. The result (Fig. S5A†) showed that at a concentration of 200 μg ml−1 HCA-Chi in both HCA-Chi and HChi-Ca-Sal B, the cell viability was higher than 80%.
FITC-labeled HChi-Ca-Sal B was used to track the intracellular uptake. As shown in Fig. S5B,† a strong green fluorescent signal was observed in HK-2 cells after co-incubation with the nanocomplex for 2 h, indicating that the complexes were easily internalized into HK-2 cells. Furthermore, lysosomes in HK-2 cells were stained with Lyso-Tracker Red (Fig. S5C†). After incubation with the HChi-Ca-Sal B nanocomplex for 4 h, most of the red fluorescent endo/lysosomes overlapped with green fluorescent FITC, as judged by the presence of yellow fluorescent spots in the merged image.
Reversal of TGF-β1-induced EMT of HK-2 cells
Generally, HK-2 cells exhibit the typical cobblestone morphology of epithelial cells in normal culture medium. After treatment for 72 h with TGF-β1, the cells became disassociated from their neighbors and lost their cobblestone monolayer pattern (Fig. S6†). After treatment with TGF-β1 for another 72 h, profound morphological changes occurred (Fig. 4A), whereas co-incubation with the HChi-Ca-Sal B nanocomplex or free Sal B mostly reversed the morphology to that of the control group. Compared with cells maintained in a medium containing TGF-β1, a significant decrease in the length/width ratio was observed for cells treated with a high concentration of the nanocomplex (Fig. 4B, Table S1†). This result implies that the nanocomplex has a dose-dependent effect on EMT reversal. HK-2 cells treated with the nanocomplex at a Sal B concentration of 100 μmol l−1 had extensive cobblestone morphology, similar to that of the untreated control cells. It is worth noting that there is no difference between the HChi-Ca-Sal B nanocomplex-treated group and the free Sal B-treated group in cell morphology or length/width ratio.
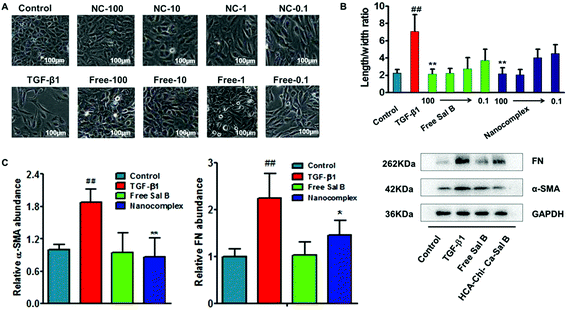 |
| Fig. 4 (A) Morphological images and (B) length/width ratio of HK-2 cells treated with the HChi-Ca-Sal B complex and free Sal B at different drug concentrations (n = 3). ##p < 0.01 vs. control group; **p < 0.01 vs. TGF-β1 group. (C) Western blot analysis of α-SMA and fibronectin levels in HK-2 cells treated with the HChi-Ca-Sal B nanocomplex and free Sal B at a Sal B dose of 100 μmol l−1. **p < 0.01 vs. TGF-β1 group. *p < 0.05 vs. TGF-β1 group. Control: 10% FBS; NC-100: 100 μmol l−1 HChi-Ca-Sal B + TGF-β1 (10 ng ml−1); NC-10: 10 μmol l−1 HChi-Ca-Sal B + TGF-β1 (10 ng ml−1); NC-1: 1 μmol l−1 HChi-Ca-Sal B + TGF-β1 (10 ng ml−1); NC-0.1: 0.1 μmol l−1 HChi-Ca-Sal B + TGF-β1 (10 ng ml−1). TGF-β1: 10% FBS + TGF-β1 (10 ng ml−1); Free-100: 100 μmol l−1 free Sal B + TGF-β1 (10 ng ml−1); Free-10: 10 μmol l−1 free Sal B + TGF-β1 (10 ng ml−1); Free-1: 1 μmol l−1 free Sal B + TGF-β1 (10 ng ml−1); Free-0.1: 0.1 μmol l−1 free Sal B + TGF-β1 (10 ng ml−1). | |
The western blot results are shown in Fig. 4C. We found that the levels of α-SMA (α-smooth muscle actin) and fibronectin were up-regulated after TGF-β1 treatment. However, when the cells were co-incubated with the nanocomplex and TGF-β1 for a further 72 h, the expression levels of the α-SMA protein (p < 0.01) and fibronectin (p < 0.05) were reduced markedly compared with those of the TGF-β1-treated EMT controls.
In vivo imaging analysis
As shown in Fig. 5A, the Cy7 fluorescent signals were observed in the kidney at 1 h after administration. The fluorescence intensity was strongest at 2 h and declined gradually with time. At 2 h post-injection, the mice were sacrificed by cervical dislocation, and the major organs, including heart, liver, spleen, lung and kidney, were excised for ex vivo imaging (Fig. 5B). The strongest Cy7 fluorescence was observed in the kidney. The free Cy7-SE solution was also injected into the mice as a comparison (Fig. S7†). Strong Cy7 fluorescent signals were observed in the liver but only weak signals were detected in the kidneys, which indicated that free Cy7 does not have renal targeting ability.
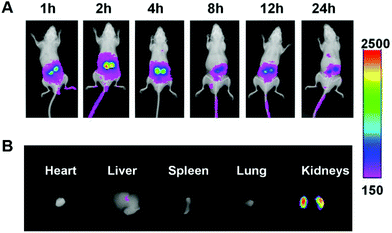 |
| Fig. 5 (A) NIRF images of ICR mice following IV injection of the Cy7-SE-labeled nanocomplex (0.1 mg kg−1 Cy7). (B) NIRF images of lung, heart, liver, spleen, intestine and kidney isolated 2 h post-injection of the nanocomplex. | |
Pharmacodynamics in the unilateral ureter obstruction (UUO) model
Histopathological examination of the kidney in the control group revealed a normal structure and no pathological changes (Fig. 6A, sham group). After UUO injury, there is extensive infiltration of inflammatory cells into the kidney, which shows marked histopathological changes, such as large fibrous areas. However, a large amount of fibrous tissue which was shown in blue stain by Masson's trichrome in Fig. 6A and the inflammatory cells detected by staining with H&E and PAS were also observed in the group treated with free Sal B (red arrows in Fig. 6A). In contrast, the histopathological kidney specimens from the group treated with the HChi-Ca-Sal B nanocomplex exhibited diminished collagen levels, and decreased levels of inflammatory cells.
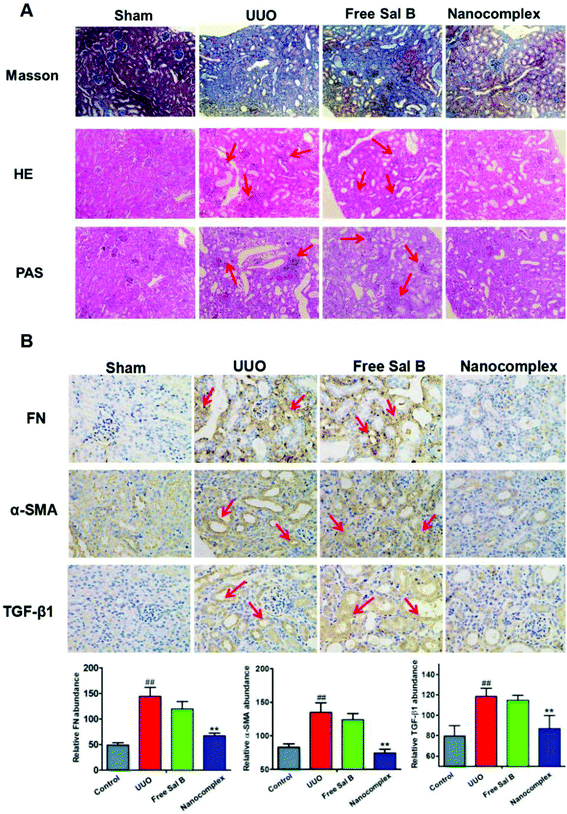 |
| Fig. 6 (A) Histological sections of kidneys from sham-operated mice, UUO mice, UUO mice treated with free Sal B, and UUO mice treated with the Sal B nanocomplex. Sections were stained with Masson's trichrome, H&E and PAS (×200). Red arrow: inflammation area. (B) Immunohistochemical analysis of kidneys from sham-operated mice, UUO mice, UUO mice treated with free Sal B, and UUO mice treated with the Sal B nanocomplex. Sections were stained with antibodies against FN (fibronectin), α-SMA and TGF-β1 (×200). Red arrows: accumulation of proteins. #p < 0.01 vs. sham mice, **p < 0.01 vs. UUO mice. | |
Immunohistochemical staining was also performed to confirm the pathological changes in the obstructed kidney in the UUO group and the anti-fibrosis activity of the HChi-Ca-Sal B nanocomplex. Immunohistochemical analysis revealed more α-SMA staining in obstructed kidneys than in normal kidneys after UUO injury (Fig. 6B). As expected, HChi-Ca-Sal B resulted in a much greater reduction of α-SMA expression than free Sal B. We also assessed the renal levels of fibronectin and TGF-β1 (brown color in Fig. 6B), two major components of the extracellular matrix, by immunohistochemistry. The lowest expression levels were observed in the HChi-Ca-Sal B-treated group.
Hydroxyproline (Hyp) content is a specific marker of collagen synthesis. We measured the Hyp content in the kidneys of the 4 groups in the UUO experiment. As shown in Fig. 7B, the kidney Hyp content increased significantly in the UUO group when compared with that of the sham group. In contrast, the kidney Hyp content of the HChi-Ca-Sal B-treated UUO mice was significantly lower than that of the UUO mice. The Hyp content in the group treated with free Sal B was slightly lower than that in the UUO group, but the difference was not significant. We also determined the plasma level of malondialdehyde (MDA), a marker of reduced renal function (Fig. 7C). MDA production was significantly increased in UUO mice compared with the sham group. However, MDA levels were significantly lower in the HChi-Ca-Sal B group than in the UUO group. MDA levels were moderately reduced in the group treated with free Sal B.
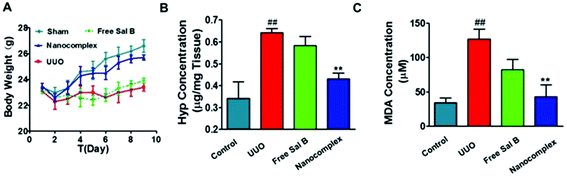 |
| Fig. 7 (A) Body weight and (B) Hyp and (C) MDA content in renal lysates from mice in the following groups: Sham, UUO, UUO + free Sal B, and UUO + nanocomplex (at a Sal B dose of 10 mg kg−1). ##p < 0.01 vs. sham mice, **p < 0.01 vs. UUO mice (n = 5). | |
To evaluate the safety of the nanocomplex, we monitored body weight variations in the treated mice during the experimental period. As shown in Fig. 7A, the surgical procedure apparently induced a temporary decrease in body weight in the animals, but they subsequently recovered. In the nanocomplex treatment group and the sham group, the body weight increased steadily compared to that of the UUO mice.
Discussion
Mussels produce an adhesive protein that is rich in catechol and amine groups, which facilitates the attachment to a variety of metal ions. Inspired by this, we synthesized catechol-modified chitosan (HCA-Chi) via EDC chemistry by forming amide bonds between the carboxylic acid group in hydrocaffeic acid and the primary amine groups in chitosan. The 1H NMR spectrum confirms the success of the coupling reaction (Fig. S1†). Unlike the unmodified chitosan, which is soluble only under acidic conditions, the HCA-Chi was soluble in acidic, neutral, and basic solutions, probably because of the presence of multiple catechol groups that can break the intramolecular hydrogen bonds and alter the secondary structure of the chitosan chain.29 The enhanced solubility of HCA-Chi is highly beneficial for the coordination reaction with metal ions.
We employed UV-Vis absorption spectroscopy to gain insight into the binding of metal ions (from copper nitrate, calcium chloride, or ferric chloride) with Sal B and HCA-Chi at different pH values.30 The Fe3+ coordination complex exhibited a lower pH-responsive point (pH 3.4) suggesting that it could resist the competitive bonding of protons under lysosomal pH conditions, which might be not beneficial for the cellular quick release behavior.31 Ca2+ and Cu2+ based systems showed a similar pH-responsive range (pH 4–7) implying the sensitive cleavage of coordination bonds in a lysosomal environment and satisfactory stability under physiological conditions. Thus we selected HCA-Chi-Ca as the metal–chitosan host for the assembly with Sal B in the following experiments, mainly because of the physiological toxicity of copper ions.
The ternary nanocomplex HCA-Chi-Ca-Sal B (HChi-Ca-Sal B) was prepared by using a two-step solvothermal method under a pH gradient. During the nanocomplex formation, the Ca2+ ions formed coordination bonds with the dihydroxyl groups of the catechol units of Sal B and HCA-Chi. TEM and DLS detection confirmed the spherical morphology of the prepared nanocomplex. WAXD analysis revealed that the HChi-Ca-Sal B nanocomplex possessed a disordered structure and Sal B existed in an amorphous or molecular state rather than in a crystalline state within the nanocomplex. In view of the uniform distribution of calcium in a solid sphere, we presumed that a single nanocomplex would probably exist as a metal–ligand hybrid structure rather than a core–shell structure, and coordination bonds exist between the ligands and metal ions. These coordination bonds, combined with several weak bonds, including hydrogen bonds, van der Waals forces, etc., are all involved in the assembly of the nanocomplexes and hence in the binding to the active compound.
As mentioned above, the formation and cleavage of metal–ligand coordination bonds are sensitive to external pH variations. The in vitro release profile of Sal B from the HChi-Ca-Sal B nanocomplex was further investigated by HPLC at different pH values, i.e. pH 7.4, 6.8 and 5.0, that simulate the normal physiological environment, inflammatory microenvironment and endo/lysosomal compartments, respectively. A pH-sensitive release behavior was observed in this experiment. As the pH of the release medium was gradually decreased from 7.4 to 5.0, the amount of Sal B released into the solution gradually increased. This can be explained by the higher concentration of protons (H+) at pH 5.0 compared to that at pH 7.4. The protons could compete with metal ions, leading to the cleavage of the coordination bonds and eventual disruption of the nanocomplexes. In addition, the nanocomplex displayed satisfactory stability, while in an acidic environment, the complex underwent disassociation and released Sal B. This pH-responsive property means that HChi-Ca-Sal B could be a promising drug delivery system for the treatment of renal fibrosis.
The safety of the nanocomplex is critically important if it is to be used for renal fibrosis therapy. The result showed that neither HCA-Chi nor the nanocomplex exhibited clear cytotoxicity at the concentrations tested. According to a previous study, chitosan-derived carriers are endocytosed by a megalin-mediated pathway in HK-2 cells.26 Our result indicated that the complexes were easily internalized into the acidic endo/lysosomes of HK-2 cells, which is critically important for the cellular release of Sal B, since the coordination bonds in the nanocomplex are lysosomal pH sensitive as described above. Furthermore, the protonation of the amino groups of HCA-Chi in the acidic environment of the endosome led to the formation of cationic charged HCA-Chi, which could also disrupt the endo/lysosome membrane. We could predict that once HChi-Ca-Sal B is endocytosed into cells, the nanocomplex will be disrupted due to the positive charge of HCA-Chi and cleavage of the coordination bonds, and Sal B will be released within the cell which will maximize its therapeutic efficiency.
Accumulating evidence suggests that tubular EMT plays a pathogenic role in renal tubulointerstitial fibrosis in response to TGF-β1.13 HK-2 cells are among the best-characterized renal epithelial cell types and have been widely used to investigate various aspects of EMT.32,33 HK-2 cells exposed to TGF-β1 for 72 h exhibited a complete conversion from epithelial cells to myofibroblasts, and maintained those characteristics for 144 h, as evidenced by the acquisition of a spindle-like morphology, activation of α-SMA and fibronectin expression. The result showed that HK-2 cells treated with the nanocomplex at a Sal B concentration of 100 μmol l−1 had extensive cobblestone morphology, similar to that of the untreated control cell lines. It is worth noting that there is no difference between the HChi-Ca-Sal B nanocomplex-treated group and the free Sal B-treated group in cell morphology or length/width ratio. It demonstrates that our nanocomplex and free Sal B are equally potent at reversing EMT in the HK-2 cell line.
In vivo imaging experiment was performed to confirm the specific renal distribution of the HChi-Ca-Sal B nanocomplex; the results showed the perfect accumulation of the nanocomplex in the kidney, whereas free Cy7 had no renal targeting ability. The specific renal targeting ability of the HChi-Ca-Sal B nanocomplex was attributed to low molecular weight chitosan (LMWC), which was specifically taken up by renal tubular cells, where the megalin receptor would likely mediate its binding and uptake.34 The reactive amino groups of the glucosamine residue in LMWC are essential for its renal-targeting ability.35 Furthermore, the degree of catechol substitution in the chitosan backbone was only approximately 6% in our study, which would not weaken the renal targeted ability of LMWC. In addition, the coordination bonds of the HChi-Ca-Sal B nanocomplex displayed satisfactory stability in the normal physiological environment which could ensure the effective delivery of Sal B in the circulation. The in vivo imaging results indicated that the HChi-Ca-Sal B coordination nanocomplex might be a promising delivery carrier of Sal B for renal fibrosis therapy, which will contribute to the enhanced therapeutic efficacy of Sal B.
The rodent model of unilateral ureteral obstruction (UUO) has been widely used to study the mechanisms of renal fibrosis.36–38 In this model, the kidney becomes fibrotic in less than a week. It has been reported that a typical feature of the fibrotic kidney is the activation and proliferation of myofibroblasts in the renal interstitium, since these cells are a major source of ECM proteins.39 The abundance of myofibroblasts in the kidney can be assessed by the accumulation of α-SMA. The UUO model was successfully established as shown by histopathological examination and immunohistochemical staining. As expected, HChi-Ca-Sal B resulted in a much better anti-fibrosis activity than free Sal B. The lowest expression levels of ECM proteins including α-SMA, fibronectin and TGF-β1 were observed in the HChi-Ca-Sal B treated group. Furthermore, the lowest Hyp content and MDA level were determined in the nanocomplex treated group. Together, these results indicated that HChi-Ca-Sal B had better anti-fibrosis efficacy than free Sal B. This can be attributed to the specific kidney-targeting ability of HChi-Ca-Sal B in the UUO model, which leads to the maximum therapeutic efficiency of Sal B.
Taken together, we have successfully synthesized catechol-conjugated chitosan which possesses the ability to coordinate with metal ions. By adjusting the external pH conditions, it was possible to form a coordination nanocomplex with calcium chloride while simultaneously loading the water-soluble drug Sal B. The prepared HChi-Ca-Sal B nanocomplex showed satisfactory stability under physiological conditions and pH-dependent drug release in an acidic environment. The HChi-Ca-Sal B nanocomplex was able to reverse the TGF-β1-induced EMT in HK-2 cells in a dose-dependent manner, as judged by the morphological conversion from myofibroblasts to cobblestone-like epithelial cells. Western blot results demonstrated that the HChi-Ca-Sal B nanocomplex decreased the expression levels of α-SMA and fibronectin. Furthermore, compared with free Sal B, HChi-Ca-Sal B significantly attenuated the morphological kidney lesions in the UUO model and down-regulated the expression of ECM proteins in the tubulointerstitium in the in vivo pharmacodynamics study. These effects can be attributed to the specific kidney-targeting ability of HChi-Ca-Sal B, which maximizes the efficacy of Sal B. In conclusion, these results demonstrated that our HCA-Chi-based coordination nanocomplex is a promising kidney-targeted delivery system for the improved treatment of renal fibrosis with Sal B.
Conflicts of interest
There are no conflicts to declare.
Acknowledgements
This work was financially supported by the National Science and Technology Major Project (2017YFA0205400), the National Natural Science Foundation of China (no. 81373983, 81573377, 81373604 and 81503259), the Natural Science Foundation (no. 20141352 and BK20151002) and the Six Talent Peaks Project (no. SWYY-011) of Jiangsu Province, the Project Program of State Key Laboratory of Natural Medicines, China Pharmaceutical University (no. SKLNMZZJQ201603, SKLNMKF201608) and the Fundamental Research Funds for the Central Universities (no. 2016PT067).
References
- L. O. Lerman and A. R. Chade, Angiogenesis in the kidney: a new therapeutic target?, Curr. Opin. Nephrol. Hypertens., 2009, 18, 160–165 CrossRef CAS PubMed.
- M. X. Li and B. C. Liu, Epithelial to mesenchymal transition in the progression of tubulointerstitial fibrosis, Chin. Med. J., 2007, 120, 1925–1930 CAS.
- S. Lovisa, V. S. LeBleu, B. Tampe, H. Sugimoto, K. Vadnagara and J. L. Carstens,
et al. Epithelial-to-mesenchymal transition induces cell cycle arrest and parenchymal damage in renal fibrosis, Nat. Med., 2015, 21, 998–1009 CrossRef CAS PubMed.
- M. Iwano, D. Plieth, T. M. Danoff, C. Xue, H. Okada and E. G. Neilson, Evidence that fibroblasts derive from epithelium during tissue fibrosis, J. Clin. Invest., 2002, 110, 341–350 CrossRef CAS PubMed.
- J. Yang and Y. Liu, Dissection of key events in tubular epithelial to myofibroblast transition and its implications in renal interstitial fibrosis, Am. J. Pathol., 2001, 159, 1465–1475 CrossRef CAS PubMed.
- Y. Liu, Renal fibrosis: new insights into the pathogenesis and therapeutics, Kidney Int., 2006, 69, 213–217 CrossRef CAS PubMed.
- M. E. Grams, E. K. Chow, D. L. Segev and J. Coresh, Lifetime incidence of CKD stages 3-5 in the United States, Am. J. Kidney Dis., 2013, 62, 245–252 CrossRef PubMed.
- Y. Zhong, Y. Deng, Y. Chen, P. Y. Chuang and J. He, Therapeutic use of traditional Chinese herbal medications for chronic kidney diseases, Kidney Int., 2013, 84, 1108–1118 CrossRef PubMed.
- Y. B. Hao, T. P. Xie, A. Korotcov, Y. F. Zhou, X. W. Pang and L. Shan,
et al. Salvianolic acid B inhibits growth of head and neck squamous cell carcinoma in vitro and in vivo via cyclooxygenase-2 and apoptotic pathways, Int. J. Cancer, 2009, 124, 2200–2209 CrossRef CAS PubMed.
- F. Wang, Y. Y. Liu, L. Y. Liu, Q. J. Zeng, C. S. Wang and K. Sun,
et al. The attenuation effect of 3,4-dihydroxy-phenyl lactic acid and salvianolic acid B on venular thrombosis induced in rat mesentery by photochemical reaction, Clin. Hemorheol. Microcirc., 2009, 42, 7–18 CAS.
- Q. Liu, H. Chu, Y. Ma, T. Wu, F. Qian and X. Ren,
et al. Salvianolic Acid B Attenuates Experimental Pulmonary Fibrosis through Inhibition of the TGF-beta Signaling Pathway, Sci. Rep., 2016, 6, 27610 CrossRef CAS PubMed.
- R. H. Pan, F. Y. Xie, H. M. Chen, L. Z. Xu, X. C. Wu and L. L. Xu,
et al. Salvianolic acid B reverses the epithelial-to-mesenchymal transition of HK-2 cells that is induced by transforming growth factor-beta, Arch. Pharmacal Res., 2011, 34, 477–483 CrossRef CAS PubMed.
- J. M. Fan, Y. Y. Ng, P. A. Hill, D. J. Nikolic-Paterson, W. Mu and R. C. Atkins,
et al. Transforming growth factor-beta regulates tubular epithelial-myofibroblast transdifferentiation in vitro, Kidney Int., 1999, 56, 1455–1467 CrossRef CAS PubMed.
- S. Akilesh, T. B. Huber, H. Wu, G. Wang, B. Hartleben and J. B. Kopp,
et al. Podocytes use FcRn to clear IgG from the glomerular basement membrane, Proc. Natl. Acad. Sci. U. S. A., 2008, 105, 967–972 CrossRef CAS PubMed.
- Z. Zhang, Q. Zheng, J. Han, G. Gao, J. Liu and T. Gong,
et al. The targeting of 14-succinate triptolide-lysozyme conjugate to proximal renal tubular epithelial cells, Biomaterials, 2009, 30, 1372–1381 CrossRef CAS PubMed.
- B. Schechter, R. Arnon, C. Colas, T. Burakova and M. Wilchek, Renal accumulation of streptavidin: potential use for targeted therapy to the kidney, Kidney Int., 1995, 47, 1327–1335 CrossRef CAS PubMed.
- Y. Yamamoto, Y. Tsutsumi, Y. Yoshioka, H. Kamada, K. Sato-Kamada and T. Okamoto,
et al. Poly(vinylpyrrolidone-co-dimethyl maleic acid) as a novel renal targeting carrier, J. Controlled
Release, 2004, 95, 229–237 CrossRef CAS PubMed.
- H. Kodaira, Y. Tsutsumi, Y. Yoshioka, H. Kamada, Y. Kaneda and Y. Yamamoto,
et al. The targeting of anionized polyvinylpyrrolidone to the renal system, Biomaterials, 2004, 25, 4309–4315 CrossRef CAS PubMed.
- C. J. Mathias, D. Hubers, P. S. Low and M. A. Green, Synthesis of [(99 m)Tc]DTPA-folate and its evaluation as a folate-receptor-targeted radiopharmaceutical, Bioconjugate Chem., 2000, 11, 253–257 CrossRef CAS PubMed.
- M. E. Dolman, S. Harmsen, G. Storm, W. E. Hennink and R. J. Kok, Drug targeting to the kidney: Advances in the active targeting of therapeutics to proximal tubular cells, Adv. Drug Delivery Rev., 2010, 62, 1344–1357 CrossRef CAS PubMed.
- H. Cabral, N. Nishiyama and K. Kataoka, Supramolecular nanodevices: from design validation to theranostic nanomedicine, Acc. Chem. Res., 2011, 44, 999–1008 CrossRef CAS PubMed.
- L. Amorin-Ferre, F. Busque, J. L. Bourdelande, D. Ruiz-Molina, J. Hernando and F. Novio, Encapsulation and release mechanisms in coordination polymer nanoparticles, Chemistry, 2013, 19, 17508–17516 CrossRef CAS PubMed.
- L. Xing, H. Zheng, Y. Cao and S. Che, Coordination polymer coated mesoporous silica nanoparticles for pH-responsive drug release, Adv. Mater., 2012, 24, 6433–6437 CrossRef CAS PubMed.
- D. Liu, C. Poon, K. Lu, C. He and W. Lin, Self-assembled nanoscale coordination polymers with trigger release properties for effective anticancer therapy, Nat. Commun., 2014, 5, 4182 CAS.
- L. Xing, Y. Cao and S. Che, Synthesis of core-shell coordination polymer nanoparticles (CPNs) for pH-responsive controlled drug release, Chem. Commun., 2012, 48, 5995–5997 RSC.
- Z. X. Yuan, Z. R. Zhang, D. Zhu, X. Sun, T. Gong and J. Liu,
et al. Specific renal uptake of randomly 50% N-acetylated low molecular weight chitosan, Mol. Pharm., 2009, 6, 305–314 CrossRef CAS PubMed.
- M. S. Lee, J. E. Lee, E. Byun, N. W. Kim, K. Lee and H. Lee,
et al. Target-specific delivery of siRNA by stabilized calcium phosphate nanoparticles using dopa-hyaluronic acid conjugate, J. Controlled Release, 2014, 192, 122–130 CrossRef CAS PubMed.
- G. Shen, R. Xing, N. Zhang, C. Chen, G. Ma and X. Yan, Interfacial Cohesion and Assembly of Bioadhesive Molecules for Design of Long-Term Stable Hydrophobic Nanodrugs toward Effective Anticancer Therapy, ACS Nano, 2016, 10, 5720–5729 CrossRef CAS PubMed.
- J. H. Ryu, Y. Lee, W. H. Kong, T. G. Kim, T. G. Park and H. Lee, Catechol-functionalized chitosan/pluronic hydrogels for tissue adhesives and hemostatic materials, Biomacromolecules, 2011, 12, 2653–2659 CrossRef CAS PubMed.
- L. Xing, H. Zheng and S. Che, A pH-responsive cleavage route based on a metal-organic coordination bond, Chemistry, 2011, 17, 7271–7275 CrossRef CAS PubMed.
- H. Qiao, M. Sun, Z. Su, Y. Xie, M. Chen and L. Zong,
et al. Kidney-specific drug delivery system for renal fibrosis based on coordination-driven assembly of catechol-derived chitosan, Biomaterials, 2014, 35, 7157–7171 CrossRef CAS PubMed.
- J. Zhou, F. Wang, H. Lu and Y. Zhang, [Impact of salvianolic acid-B on TGF-beta1-induced HK-2 epithelial-mesenchymal transition], Zhongguo Zhongyao Zazhi, 2010, 35, 89–93 CAS.
- Q. L. Wang, Y. Y. Tao, J. L. Yuan, L. Shen and C. H. Liu, Salvianolic acid B prevents epithelial-to-mesenchymal transition through the TGF-beta1 signal transduction pathway in vivo and in vitro, BMC Cell Biol., 2010, 11, 31 CrossRef PubMed.
- X. K. He, Z. X. Yuan, X. J. Wu, C. Q. Xu and W. Y. Li, Low
molecular weight hydroxyethyl chitosan-prednisolone conjugate for renal targeting therapy: synthesis, characterization and in vivo studies, Theranostics, 2012, 2, 1054–1063 CrossRef CAS PubMed.
- Z. X. Yuan, J. J. Li, D. Zhu, X. Sun, T. Gong and Z. R. Zhang, Enhanced accumulation of low-molecular-weight chitosan in kidneys: a study on the influence of N-acetylation of chitosan on the renal targeting, J. Drug Targeting, 2011, 19, 540–551 CrossRef CAS PubMed.
- M. T. Grande, B. Sanchez-Laorden, C. Lopez-Blau, C. A. De Frutos, A. Boutet and M. Arevalo,
et al. Snail1-induced partial epithelial-to-mesenchymal transition drives renal fibrosis in mice and can be targeted to reverse established disease, Nat. Med., 2015, 21, 989–997 CrossRef CAS PubMed.
- M. S. Forbes, B. A. Thornhill and R. L. Chevalier, Proximal tubular injury and rapid formation of atubular glomeruli in mice with unilateral ureteral obstruction: a new look at an old model, Am. J. Physiol. Renal. Physiol., 2011, 301, F110–F117 CrossRef CAS PubMed.
- R. L. Chevalier, M. S. Forbes and B. A. Thornhill, Ureteral obstruction as a model of renal interstitial fibrosis and obstructive nephropathy, Kidney Int., 2009, 75, 1145–1152 CrossRef PubMed.
- M. T. Grande and J. M. Lopez-Novoa, Fibroblast activation and myofibroblast generation in obstructive nephropathy, Nat. Rev. Nephrol., 2009, 5, 319–328 CrossRef CAS PubMed.
Footnote |
† Electronic supplementary information (ESI) available. See DOI: 10.1039/c7bm00811b |
|
This journal is © The Royal Society of Chemistry 2018 |