DOI:
10.1039/C7SC03482B
(Edge Article)
Chem. Sci., 2017,
8, 8030-8038
High-efficiency thermoelectric Ba8Cu14Ge6P26: bridging the gap between tetrel-based and tetrel-free clathrates†
Received
9th August 2017
, Accepted 28th September 2017
First published on 29th September 2017
Abstract
A new type-I clathrate, Ba8Cu14Ge6P26, was synthesized by solid-state methods as a polycrystalline powder and grown as a cm-sized single crystal via the vertical Bridgman method. Single-crystal and powder X-ray diffraction show that Ba8Cu14Ge6P26 crystallizes in the cubic space group Pm
n (no. 223). Ba8Cu14Ge6P26 is the first representative of anionic clathrates whose framework is composed of three atom types of very different chemical natures: a transition metal, tetrel element, and pnicogen. Uniform distribution of the Cu, Ge, and P atoms over the framework sites and the absence of any superstructural or local ordering in Ba8Cu14Ge6P26 were confirmed by synchrotron X-ray diffraction, electron diffraction and high-angle annular dark field scanning transmission electron microscopy, and neutron and X-ray pair distribution function analyses. Characterization of the transport properties demonstrate that Ba8Cu14Ge6P26 is a p-type semiconductor with an intrinsically low thermal conductivity of 0.72 W m−1 K−1 at 812 K. The thermoelectric figure of merit, ZT, for a slice of the Bridgman-grown crystal of Ba8Cu14Ge6P26 approaches 0.63 at 812 K due to a high power factor of 5.62 μW cm−1 K−2. The thermoelectric efficiency of Ba8Cu14Ge6P26 is on par with the best optimized p-type Ge-based clathrates and outperforms the majority of clathrates in the 700–850 K temperature region, including all tetrel-free clathrates. Ba8Cu14Ge6P26 expands clathrate chemistry by bridging conventional tetrel-based and tetrel-free clathrates. Advanced transport properties, in combination with earth-abundant framework elements and congruent melting make Ba8Cu14Ge6P26 a strong candidate as a novel and efficient thermoelectric material.
Introduction
Thermoelectric materials play an important role in renewable energy applications, which can convert waste heat into electrical energy and vice versa.1–3 The efficiency of thermoelectric materials is characterized by the dimensionless figure of merit, ZT = S2T/ρκ, where S is the Seebeck thermopower, T is the absolute temperature, ρ is the electrical resistivity, and κ is the thermal conductivity. In crystalline solids, the intrinsic correlations between these three transport properties make enhancement of thermoelectric efficiency challenging due to their dependence on carrier concentration and electronic structure.4–9 The Phonon Glass-Electron Crystal (PGEC) concept was proposed as a way to guide thermoelectric research.10–13 Clathrate compounds, also known as host–guest compounds, are considered to be PGEC compounds due to their covalent three-dimensional frameworks and rattling of guest atoms. The framework acts as a good electrical conductor, while the rattling of guest atoms encapsulated in the framework cages efficiently scatters phonons to reduce thermal conductivity.12,14–23 Besides thermoelectric applications, clathrates are used as photovoltaic materials, Li-batteries anodes, superconductors, and gas storage materials.16,17
Based on the framework-forming elements, all anionic clathrates can be divided into two main groups: tetrel-based (Si, Ge, Sn) and tetrel-free clathrates. The latter group, which is much smaller than the former one, can also be called pnicogen-based or transition metal-based clathrates since the frameworks of all reported tetrel-free clathrates are formed by a combination of the late transition metals of group 10–12 with pnicogen atoms: P, As, or Sb.17 Both groups have distinct chemical and structural properties. One specific feature of tetrel clathrates is the formation of locally disordered frameworks where one crystallographic position is jointly occupied by different atoms, such as Ga/Ge or Zn/Sn.16,17 In turn, tetrel-free clathrates show full segregation of transition metal and phosphorus atoms over different framework positions, which result in the formation of either long-range ordered structures, as in the case of Ba8M16P30, BaM2P4, and Ba8Zn11Cu13P28+δ (M = Cu, Au, Ni), or short-range ordering, as in the case of Ba8(Cu/Zn)16+yP30−y.15,18,24–26
In this work, we report a new type-I clathrate, Ba8Cu14Ge6P26, which combines a transition metal, tetrel element, and pnicogen in its framework. This compound was synthesized and grown as a large crystal through the Bridgman technique. A uniform distribution of the constituent elements and the absence of short- or long-range ordering in the Ba8Cu14Ge6P26 clathrate was confirmed by a combination of synchrotron powder X-ray diffraction, high-angle annular dark field scanning transmission electron microscopy, and X-ray and neutron pair distribution function analyses. Thermoelectric measurements demonstrate that Ba8Cu14Ge6P26 is a p-type semiconductor with a remarkable figure of merit. This newly discovered clathrate builds a bridge between the two clathrate families.
Experimental section
Synthesis
All preparation and handling of samples were performed in an argon-filled glovebox with the O2 level below 1 ppm. All starting materials were commercial grade and were used as received: Ba chunks (Sigma-Aldrich, 99.9%), Cu powder (Sigma-Aldrich, 99.9%), Ge pieces (Alfa Aesar, 99.9999%) and red P powder (Alfa Aesar, 99%).
The polycrystalline sample of Ba8Cu14Ge6P26 was obtained via the solid-state reaction of the elements. The elements with a stoichiometric Ba/Cu/Ge/P ratio of 8/14/6/26 were loaded into a carbonized silica ampoule, evacuated, and flame-sealed. The ampoule was first heated from room temperature to 1123 K over 17 h, and then it was annealed at this temperature for 144 hours. After the furnace was turned off and cooled, the samples were ground and reloaded into a new carbonized silica ampoule in the glovebox, resealed, and reannealed using the same temperature profile as the first annealing. The same procedure was repeated a third time. After three annealings, a uniform melted chunk was found as the final product, and was characterized as single-phase by powder X-ray diffraction (Fig. 1 and S1†).
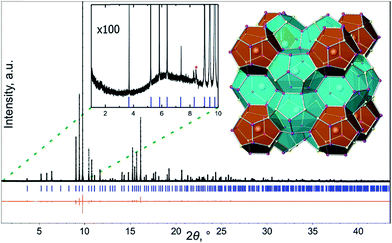 |
| Fig. 1 Results of Rietveld refinement of the synchrotron powder X-ray diffraction data of Ba8Cu14Ge6P26. Experimental pattern: black crosses; calculated pattern: black line; calculated peak positions: blue sticks; difference curve: orange line. Left inset: enhanced view of the low angle part of the experimental pattern with the calculated peak positions shown as blue sticks. One admixture peak is indicated with red star. Right inset: general view of the crystal structure with two types of polyhedra highlighted in (pentagonal dodecahedra) brown and (tetrakaidecahedra) cyan. | |
Differential scanning calorimetry
A Netzsch Differential Scanning Calorimeter (DSC) was used to characterize the thermal behavior of Ba8Cu14Ge6P26. A powder sample (mass: 35.3 mg) was sealed inside an evacuated silica ampoule, heated to 1273 K and cooled down to room temperature with the 10 K min−1 rate.
Bridgman growth
About 5 g of single-phase polycrystalline sample was ground into fine powder in a mortar and loaded into a carbonized silica ampoules with a sharp cone end. The ampoule was evacuated and flame-sealed. This ampoule was further sealed in another thick-walled silica ampoule, which was used to protect the growth tube. The silica ampoule was placed into a home-made two-zone vertical Bridgman furnace for crystal growth. An “oscillating” temperature profile was first applied to melt the sample thoroughly. When the ampoule was placed in the hot zone, the temperature in the hot zone was set to 1208(5) K for 24 hours, then cooled down to 973(5) K for 12 hours and afterwards the temperature was increased to 1123(5) K for 120 hours. After the melt process, the ampoule was dropped at a rate of 2.3 mm h−1 from the hot zone to the cold zone, where the temperature in the hot zone was 1123(5) K, and the temperature of the cold zone was 973(5) K. The crystal growth was completed in five days. Several crystals were grown to check for reproducibility. The Archimedean densities of the crystal slices vary in the range of 99.4–99.8% from theoretical X-ray density.
Elemental analysis
Elemental analysis of selected crystals was carried out on a Hitachi S4100T scanning electron microscope (SEM) with energy-dispersive X-ray (EDX) microanalysis (Oxford INCA Energy) to check for a consistent elemental ratio of the elements in the samples (Fig. S3 and Table S3†).
Single-crystal X-ray diffraction
Single-crystal diffraction experiments were collected at 90 K using a Bruker AXS SMART diffractometer with an APEX-II CCD detector with Mo-Kα radiation. The datasets were recorded as ω-scans with a 0.4° step width and integrated with the Bruker SAINT software package.27 Multi-scan absorption corrections were applied.27 The solution and refinement of the crystal structure were carried out using the SHELX-2014 suite of programs.28 The final refinements were performed using anisotropic atomic displacement parameters for all atoms. A summary of pertinent information relating to unit cell parameters, data collection, and refinements is provided in Table 1 and the atomic parameters and interatomic distances are provided in Tables S1 and S2.† Further details of the crystal structure determination may be obtained from Fachinformationszentrum Karlsruhe, Germany, by quoting the depository number CSD-433052.
Table 1 Selected single crystal data and structure refinement parameters for Ba8Cu14Ge6P26
Temperature |
90(2) K |
Radiation, wavelength |
Mo-Kα, 0.71073 Å |
Space group |
Pm n (no. 223) |
Unit cell dimensions |
a = 10.0626(8) Å |
Unit cell volume, Z |
1018.9(2) Å3, 1 |
Density (calc.) |
5.26 g cm−3 |
Absorption coefficient |
19.61 cm−1 |
Data/parameters |
333/25 |
Goodness-of-fit |
1.14 |
Final R indices [I > 2σ(I)] |
R
1 = 0.015 |
wR2 = 0.025 |
Final R indices [all data] |
R
1 = 0.017 |
wR2 = 0.026 |
Max diff. peak and hole |
0.80 and −0.53 |
Transmission electron microscopy (TEM)
Samples for electron microscopy were ground under ethanol, and the resulting dispersion was transferred to a holey carbon film fixed on a 3 mm copper grid. Electron diffraction (ED) studies were performed using a Tecnai G2 30 UT (LaB6) microscope operated at 300 kV with 0.17 nm point resolution. High-angle annular dark field (HAADF)-scanning TEM (STEM) studies and EDX elemental mapping were performed using a JEM ARM200F cold FEG double aberration corrected electron microscope operated at 200 kV and equipped with a large solid-angle CENTURIO EDX detector and Quantum EELS spectrometer.
X-ray and neutron powder diffraction and PDF
All samples were characterized by X-ray powder diffraction (XRD) using a Rigaku Miniflex 600 diffractometer employing Cu-Kα radiation. High-resolution room temperature synchrotron powder XRD data were collected at beamline 11-BM (λ = 0.459266 Å) at the Advanced Photon Source (APS) at Argonne National Laboratory (ANL) (Fig. 1). The refined unit cell parameter of Ba8Cu14Ge6P26 from synchrotron X-ray diffraction data at room temperature, a = 10.09454(1) Å, agrees with the result obtained from single-crystal refinement, a = 10.0626(8) Å at 90 K. Neutron powder diffraction time-of-flight data for neutron pair distribution function (N-PDF) were collected at the NOMAD beamline at the Spallation Neutron Source (SNS) at Oak Ridge National Laboratory (ORNL). Additional high-energy total X-ray scattering (X-PDF) data (λ = 0.21 Å) were collected at the PDF beamline 11-ID-B at APS ANL. Neutron and X-ray PDF data were analyzed using PDFGUI.29,30
Transport properties
Transport properties were studied on several slices of different Bridgman-grown crystals. Low-temperature transport properties in the temperature range of 2–400 K were studied using the commercial multipurpose Physical Properties Measurement System (PPMS, Quantum Design). The Seebeck thermopower and thermal conductivity were measured using the thermal transport option. The electrical resistivity was measured by a standard four-point alternating-current technique to exclude the resistance of the leads. High-temperature thermal conductivity was carried on a Netzsch LFA 457. The Seebeck coefficient and resistivity measurements were conducted simultaneously on a Linseis LSR-3/1100 instrument system under helium atmosphere. The high-temperature data were additionally verified at the Jet Propulsion Laboratory using both custom and commercial apparatus. The Seebeck coefficient was measured using the light-pipe method with tungsten–niobium thermocouples under high vacuum in a custom set-up.31 Temperature-dependent resistivity was measured using a Van der Pauw 4-point method with tungsten pressure contact probes.32 Thermal diffusivity was measured using a Netzsch LFA 404 system. The temperature was limited to 800 K to prevent sublimation of the samples during measurements. All transport measurements were taken during both heating and cooling (heating rate of 180 K h−1), and showed no hysteresis. The combined measurement uncertainty in the thermoelectric figure of merit is generally assumed to be ∼20%.
Results and discussion
Composition and crystal structure
Our motivation to search for new clathrates was to produce electron-balanced compounds with the semiconductor-like properties that are highly desirable for thermoelectric applications. The clathrate Ba8Cu16P30 was reported to exhibit metallic properties due to an insufficient number of valence electrons to form four bonds per clathrate framework atom.25,33,34 In this work we realized an electron-balanced compound by the replacement of some Cu (1 valence electron) and P (5 valence electrons) atoms with Ge (4 valence electrons) atoms. Several synthesized samples with different nominal Ba/Ge/Cu/P ratios contained the same phase with a similar composition as was evidenced by EDX and unit cell parameter determinations. The precise elemental ratio in the new clathrate was determined by a combination of three different methods. First, the refinement of single-crystal X-ray diffraction data resulted in the chemical formula of Ba8M20.1(2)P25.9(2), where M indicates the merging of Cu and Ge elements due to their similar X-ray atomic scattering factors. Tests of different crystals selected from different batches all resulted in an identical composition within the standard deviations of the refinements. Afterwards, the chemical composition of different crystals analyzed by EDX spectroscopy resulted in the formula Ba8Cu14.4(2)Ge5.5(1)P22(1) when normalized to 8 Ba atoms (Table S3†). Note that light P atoms cannot be accurately determined by EDX, and some overlap of Cu and Ge lines hinder precise determination of the elemental contents. However, the averaged amount of Cu + Ge from EDX is 19.9(3), which agrees very well with the results from single-crystal diffraction. Finally, Zintl counting exactly predicts the Ba8Cu14Ge6P26 composition for 20 metal Cu + Ge atoms. Indeed, in the clathrate-I system Ba8X46, where X is the framework atom, each Ba atoms donates 2 electrons to the framework becoming Ba2+, and 4 electrons are required for per framework atom, X, to achieve an electron balance by forming 4 covalent bonds in the framework.17 Since there are 46 atoms in the framework, a total of 46 × 4 = 184 electrons are required. Besides the 16 electrons from Ba atoms, the framework atoms need additional 168 electrons coming from X elements. Assuming the total Cu + Ge content is equal to 20 and 26 P atoms in the framework, the only electron-balanced solution is 14 Cu atoms (14 × 1 = 14 electrons), 6 Ge atoms (6 × 4 = 24 electrons), and 26 P atoms (26 × 5 = 130 electrons), resulting in exactly 168 electrons. The electron-balanced nature of Ba8Cu14Ge6P26 was further confirmed by properties measurements (vide infra). All our attempts to synthesize samples with Ba8Cu14+xGe6−xP26 nominal compositions, where x varies from 0.1 to 1, resulted in no detectable unit cell parameter shifts or changes in transport properties, indicating that Ba8Cu14Ge6P26 is a line compound.
Ba8Cu14Ge6P26 crystallizes in the clathrate-I structure type with the space group Pm
n (no. 223). Clathrate-I is the most abundant structure type among inorganic clathrates. Its crystal structure can be described as composed of two types of polyhedra: pentagonal dodecahedra and tetrakaidecahedra. The Ba atoms are encapsulated inside both types of polyhedra. Tetrakaidecahedra share their hexagonal faces, forming columns running along all three main crystallographic directions and the residual space filled by dodecahedra (Fig. 1 inset). There is no evidence for vacancies on the Ba sites. Three framework sites, 6c, 16i, and 24k are jointly occupied by Cu, Ge and P. Cu and Ge, atomic numbers 29 and 32, are difficult to distinguish by X-ray diffraction due to similar atomic scattering factors. During the refinement, all framework sites were originally refined as jointly occupied by Cu and P under the constraints of equivalent atomic displacement parameters and the total occupancy of each site was fixed to 100%. Similar refinements with all framework positions set as Ge/P resulted in a slightly lower but similar M/P ratio. At the final stages of the refinement, each framework site was refined as jointly occupied by Cu + Ge + P. An additional compositional restraint was applied to fix the total elemental content in the structure to 14Cu + 6Ge + 26P (Tables 1, S1, and S2†). Mixed occupancy of the framework positions by several different elements, including Cu/Ge mixing, is common for the tetrel clathrates, such as Ba8Ga16Ge30,35 Ba8Ga17.1Ge25.6Sb2.7,36 and Ba8Cu6−xGe40+x.37
Superstructures and partial orderings are well-known in the crystal chemistry of clathrates. For the type-I clathrate, the aristotype structure crystallizes in space group Pm
n (no. 223) with a ≈ 10 Å and volume V ≈ 1000 Å3. Different kinds of superstructures have been reported for type-I clathrates ranging from lowered symmetry within the same volume unit cell38 to an increase of the unit cell volume by a factor of 4–8 due to either the ordering of vacancies in the tetrel-based clathrates, as in the cases of Cs8Sn44 or Ba8Ge43, or the separation of atoms of different chemical natures over distinct framework sites, as in the case of Ba8Cu16P30 and Ba8Au16P30.15,24,39–41 The vacancies in the framework or guest positions do not necessarily lead to the formation of a superstructure.42,43 Alternatively, the formation of short-range ordering without a long-range superstructure is possible, but is more difficult to detect with diffraction techniques and requires the application of local probes. For example, the recently reported type-I clathrate Ba8M16+yP30−y (M = Cu, Zn) exhibits partial superstructural ordering confirmed with ED, STEM, and PDF, and can also be detected due to presence of weak extra diffraction peaks in its synchrotron powder diffraction pattern.25 No such extra peaks were detected in the diffraction pattern of Ba8Cu14Ge6P26 sample (Fig. 1 inset), which demonstrates the absence of long-range superstructural ordering in Ba8Cu14Ge6P26. To further investigate possible short-range ordering, we applied electron microscopy.
Elemental mapping shows uniform distributions of the Ba, Cu, Ge, and P elements over the entire studied crystals (Fig. 2b). All observed diffraction spots in the ED patterns can be indexed in the cubic clathrate-I unit cell. No superstructural reflections were found (Fig. 2a). This is in a stark contrast to the Ba8M16+yP30−y (M = Cu, Zn) clathrate where preferential Cu–Zn bonding resulted in the formation of a trigonal superstructure, detectable by ED.25 High-resolution HAADF-STEM investigations confirm the well-ordered cubic nature of the Ba8Cu14Ge6P26 crystallites with good agreement to the overlayed structural models (Fig. 2c). No structural defects, like antiphase boundaries or stacking faults, were observed in the studied Ba8Cu14Ge6P26 crystallites.
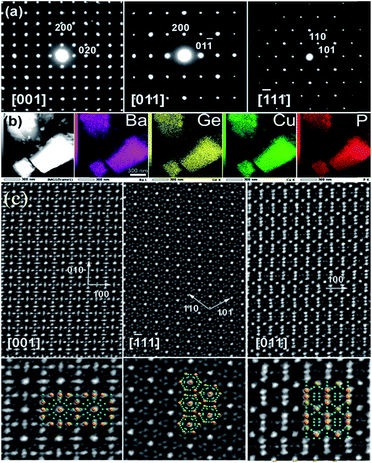 |
| Fig. 2 (a) ED patterns along the main cubic zones and (b) elemental mapping of selected Ba8Cu14Ge6P26 crystals. Ba: purple, Ge: yellow, Cu: green, P: red. (c) HAADF-STEM images along main zone axes [001], [ 11] and [011] of Ba8Cu14Ge6P26 are shown. The insets in the bottom show structural fragment overlaps (Ba: yellow, Cu/Ge/P: blue). | |
Synchrotron X-ray and neutron PDF analyses also confirmed the absence of local ordering in Ba8Cu14Ge6P26 (Fig. 3). Independent fittings of the short-range (1.9 Å ≤ r ≤ 9.9 Å) and long range (9.9 Å ≤ r ≤ 19.9 Å) PDF regions were performed using the cubic Pm
n model. Due to the similarity of the neutron scattering lengths for Cu (7.7 fm) and Ge (8.2 fm) the occupancies of the atomic positions in the framework were fixed to the values obtained from the single crystal X-ray diffraction experiment and were not refined further. The structural model determined from the single crystal X-ray experiment describes the PDF patterns well, indicating the absence of any short-range ordering. The presence of local ordering in the clathrate framework results in significant perturbations of the short-range PDF data.25
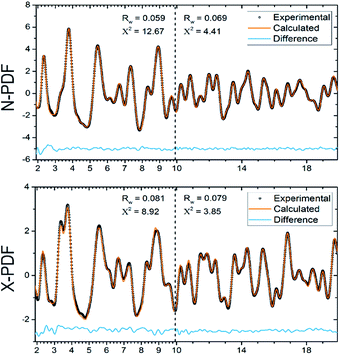 |
| Fig. 3 Experimental neutron (top) and X-ray (bottom) pair distribution functions and their fits are shown for a cubic model of Ba8Cu14Ge6P26. Experimental data are open black circles, calculated fits are red lines, and difference curves are shown as blue lines. | |
Based on TEM, synchrotron XRD, and PDF results, the crystal structure of Ba8Cu14Ge6P26 is established to have primitive cubic Pm
n symmetry with a uniform distribution of the framework elements and without local ordering, superstructure, or defects. The main difference between the Cu–Zn–P and Cu–Ge–P frameworks is the preference for Cu and Zn to form chemical bonds to each other. We have elegantly shown that an increase of the Zn content resulted in the formation of a clathrate where all metal atoms are joined in Cu–Zn dumbbells.26 Apparently, such bonding preference is absent in the case of the Ba–Cu–Ge–P clathrate, which resulted in the formation of the cubic structure with a uniform distribution of Cu, Ge, and P over the clathrate framework sites.
Although clathrate-I is a well-known structure type, Ba8Cu14Ge6P26 is the first representative of inorganic clathrates with electropositive guest atoms where the framework is composed of a transition metal, tetrel element, and pnicogen. The tetrel-based clathrates are a prevailing group with over 100 compounds with diverse properties.17 Until now, only a dozen tetrel-free clathrates have been reported, which include phosphides, Ba8M16P30 (ref. 15 and 24) and AeM2P4 (Ae = Sr, Ba; M = Ni, Cu),18 arsenides and antimonides, A8E18Pn28, A = Rb, Cs; E = Zn, Cd; Pn = As, Sb.44,45 Prior to our work, there was an insurmountable gap between these two clathrate groups, despite the reported attempts to dope Sb into tetrel clathrates.36 Ba8Cu14Ge6P26 fills the gap between tetrel-based and pnicogen-based clathrates and provides new insight and opportunities for clathrate research. High thermal stability and the electron-balanced nature of this clathrate result in outstanding transport properties.
Thermal stability
DSC characterization results for Ba8Cu14Ge6P26 are shown in Fig. 4 and S4.† Ba8Cu14Ge6P26 melts congruently at 1108(3) K and recrystallizes at 1006(3) K. No decomposition or phase transition were detected upon melting and crystallization, which was verified by powder XRD (Fig. S2†). The thermal stability of Ba8Cu14Ge6P26 was further confirmed by powder XRD of the phase after annealing a single-phase sample in a sealed tube at 1223 K for 12 hours. The congruent melting of Ba8Cu14Ge6P26 makes the single-crystal growth process feasible. We applied the vertical Bridgman method to grow large crystals of Ba8Cu14Ge6P26 (Fig. 4 bottom inset). A slice of the grown crystal with a mirror-like surface is also shown in Fig. 4 top inset.
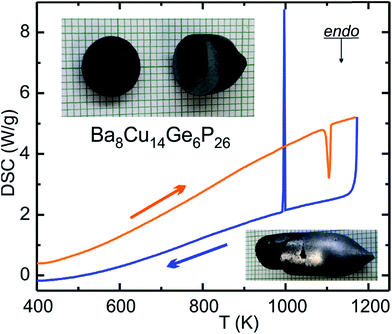 |
| Fig. 4 DSC results for Ba8Cu14Ge6P26. Heating: orange curve; cooling: blue curve. The inset are photographs of (bottom) Bridgman growth crystal and (top) slices of such crystals with mirror-like surface on a background of mm-grid paper. | |
Thermoelectric properties
The presence of grain boundaries may mask the intrinsic properties of solids. In thermoelectric characterization of polycrystalline materials, the effect of grain boundaries is minimized by studying dense pellets. However, large crystals are more suitable for studying the intrinsic properties of compounds. For Ba8Cu14Ge6P26, characterization of slices from several crystals grown by the vertical Bridgman method were performed (Fig. 4 inset). The data obtained at UC Davis were verified by measurements at JPL. Note that JPL measurements were run on a slice of a different crystal and using different equipment (Fig. S6†). Both the low-temperature and high-temperature thermoelectric properties show good agreement with each other with small discontinuities at 300–400 K due to the different methods and instruments used (Fig. 5).
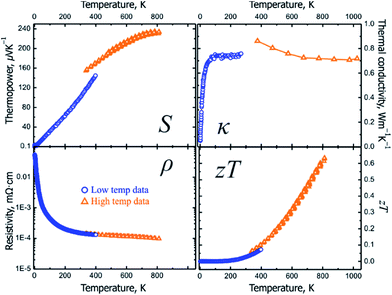 |
| Fig. 5 Low- (blue) and high-temperature (orange) transport properties on a slice of the Bridgman growth crystal of Ba8Cu14Ge6P26: (top left) Seebeck thermopower; (top right) thermal conductivity; (bottom left) electrical resistivity; (bottom right) thermoelectric figure of merit, ZT. The estimated standard deviations for ZT are ∼20%, see Fig. S6† and discussion therein. | |
The thermopower for Ba8Cu14Ge6P26 samples is positive in the whole studied temperature range (10–812 K) indicating that holes are the main charge carrier type. The Seebeck thermopower was measured several times during heating and cooling in the high-temperature range with good consistency. At room temperature, a promising value for thermopower, 102 μV K−1, was achieved. At the maximum measured temperature, 812 K, the thermopower value increases up to 234 μV K−1 (Table 2 and Fig. 5).
Table 2 A summary of the thermoelectric properties of Ba8Cu14Ge6P26 and related clathrates
|
At 300 K |
At 812 K |
Ref. |
S μV K−1 |
ρ μΩ m |
κ W m−1 K−1 |
ZT
|
S μV K−1 |
ρ μΩ m |
κ W m−1 K−1 |
ZT
|
Ba8.01Ga15.79Al2.95Ge26.91 is a p-type Ge-based clathrate with high thermoelectric performance.
Data at peak ZT temperature of 750 K.
|
Ba8Cu14Ge6P26 |
101.5 |
149.7 |
0.77 |
0.03 |
234 |
98 |
0.7 |
0.63 |
This work |
Ba8Cu5.3Ge39.8 |
154 |
1610 |
1.4 |
0.003 |
— |
— |
— |
— |
46
|
Ba8Ga15.8Al3Ge27a |
187 |
83 |
1.05 |
0.12 |
254b |
78b |
1.0b |
0.6b |
47
|
Ba8Cu16P30 |
12.8 |
11.7 |
1.2 |
0.004 |
46 |
19 |
1.4 |
0.07 |
This work |
Ba8Cu14Ge6P26 is the first quaternary anionic clathrate connecting tetrel-based and tetrel-free clathrate families. It is reasonable to compare the properties of Ba8Cu14Ge6P26 to the properties of its ternary counterparts, Ba8Cu5.3Ge39.8 (ref. 46) and Ba8Cu16P30, which are also p-type conductors. Additionally, the performance of Ba8Cu14Ge6P26 is compared to Ba8Ga15.8Al3Ge27, the one of the best performing Ge-based, p-type thermoelectric clathrate (Table 2).47 For Ba8Cu16P30, no high-temperature thermoelectric properties have been reported,33 so we re-synthesized this sample and measured high-temperature thermoelectric properties shown in the last line of Table 2. The Seebeck thermopower value at 300 K of Ba8Cu14Ge6P26, 102 μV K−1, is much higher than that for Ba8Cu16P30 (13 μV K−1) and lower than the thermopowers for Ba8Cu5.3Ge39.8 (154 μV K−1) and Ba8Ga15.8Al3Ge27 (187 μV K−1). With increasing temperature, the Seebeck value of Ba8Cu14Ge6P26, 234 μV K−1 at 812 K, is comparable to the thermopower of the Ba8Ga15.8Al3Ge27 clathrate, 254 μV K−1 at 750 K.
The resistivities of multiple single-crystalline and pressed polycrystalline Ba8Cu14Ge6P26 samples decrease with increasing temperature over the whole temperature range, which indicates a thermally activated behavior typical for semiconductors (Fig. 5, bottom left). The resistivity of Ba8Cu14Ge6P26 at 300 K is 150 μΩ m, which decreases to 98 μΩ m at 812 K. Ba8Cu16P30 exhibits lower resistivity, 12 μΩ m, at 300 K. In turn, the room temperature resistivity for Ba8Cu5.3Ge39.8 is much higher, 1610 μΩ m. At 812 K, the resistivity of Ba8Cu14Ge6P26 remains the highest compared to the resistivities of Ba8Ga15.8Al3Ge27 and Ba8Cu16P30 (Table 2). The resulting power factor for Ba8Cu14Ge6P26 at 812 K is 5.62 μW cm−1 K−2. The relatively high resistivity of Ba8Cu14Ge6P26 indicates that further optimization of its thermoelectric efficiency can be performed via tuning the electrical conductivity by charge carrier concentration modifications. For example, for Ba8Ga16+xGe30−x p-type clathrates, the typical values of ZT are less than 0.5.48 However, a significantly higher performance was recently reported for a Bridgman growth sample with the composition Ba8Ga16.6Ge28.7.49 While the Seebeck coefficient and thermal conductivity for this clathrate are comparable to those of Ba8Cu14Ge6P26, adjustment of the Ga/Ge ratio resulted in the significant reduction of resistivity down to 35 μΩ m at 823 K.49 Investigations of aliovalent doping into the cation sublattice of Ba8Cu14Ge6P26 are currently underway.
Ba8Cu14Ge6P26 inherits the low thermal conductivity of the clathrate family of structures due to its intrinsically complex host–guest structure and rattling of guest atoms.10,16,17 The thermal conductivity of Ba8Cu14Ge6P26 increases with temperature and reaches a plateau at 100 K of 0.75 W m−1 K−1 without a pronounced maximum in the 50–150 K range, which is not typical for crystalline solids and more common for amorphous materials. Such behavior was observed for some clathrates with complex disorder in the framework.16,50,51 The statistical disorder of Cu, Ge, and P seems to be responsible for such behavior and the extremely low values of the thermal conductivity for Ba8Cu14Ge6P26. As temperature increases, the thermal conductivity decreases down to a value of 0.72 W m−1 K−1 at 812 K. The thermal conductivity value of Ba8Cu14Ge6P26 is lower than the thermal conductivities of a majority of clathrate compounds (1–3 W m−1 K−1).16,17 The thermal conductivity of Ba8Cu14Ge6P26 is also comparable to that of some of the state-of-art thermoelectric materials, such as Yb14MnSb11 (0.7 W m−1 K−1 at 300 K),52 Bi0.5Sb1.5Te3 (0.7 W m−1 K−1 at 300 K),53 [010] direction of the SnSe single crystal (0.7 W m−1 K−1 at 300 K),54 and Ca3AlSb3 (1.3 W m−1 K−1 at 300 K).55,56 The thermal conductivity consists of a charge carrier contribution and phonon contribution, κtotal = κe + κL = LT/ρ + κL (κe: electronic thermal conductivity; κL: lattice thermal conductivity; L: Lorenz number; ρ: electrical resistivity; T: absolute temperature), where the L can be estimated based on the Seebeck coefficient values.57 The lattice and electronic contributions to total thermal conductivity for Ba8Cu14Ge6P26 are shown in Fig. S5.† The lattice thermal conductivity exhibits a similar trend to the total thermal conductivity and reaches 0.58 W m−1 K−1 at 812 K, which is comparable to the lattice thermal conductivity at 800 K for many state-of-the-art thermoelectric materials, such as Yb14MnSb11 (0.51 W m−1 K−1),58 Sr3GaSb3 (0.52 W m−1 K−1),59 and Ba8Ga16Ge30 (0.65 W m−1 K−1).60
Finally, due to a combination of the high thermopower, ultralow thermal conductivity, and intermediate electrical conductivity, the Bridgman growth sample of Ba8Cu14Ge6P26 exhibits a promising thermoelectric figure of merit, ZT, of 0.63 at 812 K (Fig. 5, bottom right). The average ZT for the 400–800 K range is 0.35. The maximum ZT of Ba8Cu14Ge6P26 at 812 K is 9 times higher than the value for the ternary tetrel-free counterpart, Ba8Cu16P30 (0.07). Ba8Cu14Ge6P26 is among the most-efficient p-type Ge-containing clathrate thermoelectrics. Compared to the highly tuned and more expensive Ba8Ga15.8Al3Ge27 compound, the initial achievement of ZT = 0.63 makes Ba8Cu14Ge6P26 an attractive platform for the development of mid- and high-temperature thermoelectrics. Ba8Cu14Ge6P26 exhibits comparable thermoelectric efficiency to the unoptimized Yb14MnSb11, which is the base for the development of the most efficient high-temperature p-type thermoelectric materials.52,61–64 For example, at 812 K the thermoelectric efficiency for Ba8Cu14Ge6P26 and pristine Yb14MnSb11 are 0.63 and 0.53, respectively. Many strategies to tune carrier concentration and enhance electrical conductivity, such as doping,5 band engineering,65 and aliovalent substitutions in the framework and guest sublattices16,17 may be applied to improve the thermoelectric efficiency of Ba8Cu14Ge6P26. From a chemistry point-of-view, a novel clathrate system connecting tetrel-based clathrates and tetrel-free clathrates may reinvigorate interest in searching for new clathrates with high thermoelectric performance.
Conclusions
A new clathrate compound, Ba8Cu14Ge6P26, was synthesized by a traditional solid-state reaction and grown as single crystal via the vertical Bridgman growth method. Ba8Cu14Ge6P26 crystallizes in a type-I clathrate structure with cubic system Pm
n space group. The three-dimensional framework is built from uniformly distributed Cu, Ge and P atoms, which is confirmed by ED, STEM, single crystal and powder synchrotron diffraction, and X-ray and neutron PDF analyses. The congruent melting of Ba8Cu14Ge6P26, as revealed by DSC, allows for crystal growth via vertical Bridgman growth, which is beneficial for exploring intrinsic physical properties. Thermoelectric characterizations show that Ba8Cu14Ge6P26 is a p-type semiconductor with a promising figure of merit, ZT = 0.63 at 812 K. Ba8Cu14Ge6P26 exhibits auspicious thermoelectric properties outperforming majority of p-type Ge-based and tetrel-free clathrates. The efforts to reduce the resistivity of Ba8Cu14Ge6P26 through aliovalent doping are currently underway.
Conflicts of interest
There are no conflicts to declare.
Author contributions
All authors have given approval to the final version of the manuscript.
Funding sources
This research was supported by the U.S. Department of Energy, Office of Basic Energy Sciences, Division of Materials Science and Engineering under Award DE-SC0008931. Part of this work was performed at the California Institute of Technology/Jet Propulsion Laboratory under contract with the National Aeronautics and Space Administration. This part of the work was supported by the NASA Science Missions Directorate's Radioisotope Power Systems Thermoelectric Technology Development Project. Use of the Advanced Photon Source at Argonne National Laboratory was supported by the U.S. Department of Energy, Office of Science, Office of Basic Energy Sciences, under Contract No. DE-AC02-06CH11357. Research conducted at ORNL's Spallation Neutron Source was sponsored by the Scientific User Facilities Division, Office of Basic Energy Sciences, U.S. Department of Energy.
Acknowledgements
The authors wish to thank Prof. Dr S. M. Kauzlarich for use of SPS, DSC, LFA, and LSR-3 equipment; S. Lapidus at the APS ANL for collecting synchrotron XRD pattern; and K. Page, and J. Nueufeind at the SNS ORNL for help with the neutron PDF measurements.
References
- T. M. Tritt, Science, 1996, 272, 1276 CrossRef CAS.
- L. Bell, Science, 2008, 321, 1457–1461 CrossRef CAS PubMed.
- F. J. DiSalvo, Science, 1999, 295, 703–706 CrossRef.
- G. J. Snyder and E. S. Toberer, Nat. Mater., 2008, 7, 105–114 CrossRef CAS PubMed.
-
D. M. Rowe, Thermoelectrics Handbook: Macro to Nano, CRC/Taylor & Francis, Boca Raton, FL, 2006 Search PubMed.
- J. R. Sootsman, D. Y. Chung and M. G. Kanatzidis, Angew. Chem., Int. Ed., 2009, 48, 8616–8639 CrossRef CAS PubMed.
- G. Tan, L. D. Zhao and M. G. Kanatzidis, Chem. Rev., 2016, 116, 12123–12149 CrossRef CAS PubMed.
- J. Wang and K. Kovnir, J. Am. Chem. Soc., 2015, 137, 12474–12477 CrossRef CAS PubMed.
- J. Wang, X. C. Liu, S. Q. Xia and X. T. Tao, J. Am. Chem. Soc., 2013, 135, 11840–11848 CrossRef CAS PubMed.
-
G. A. Slack, CRC Handbook of Thermoelectrics, ed. D. M. Rowe, CRC Press, Boca Raton, FL, 1995, p. 407 Search PubMed.
- M. Beekman, D. T. Morelli and G. S. Nolas, Nat. Mater., 2015, 7, 1182–1185 CrossRef PubMed.
- T. Takabatake, K. Suekuni, T. Nakayama and E. Kaneshita, Rev. Mod. Phys., 2014, 86, 669 CrossRef CAS.
- C. Xiao, J. Xu, B. Cao, K. Li, M. Kong and Y. Xie, J. Am. Chem. Soc., 2012, 134, 7971–7977 CrossRef CAS PubMed.
- M. Christensen, S. Johnsena and B. B. Iversen, Dalton Trans., 2010, 39, 978–992 RSC.
- J. Fulmer, O. I. Lebedev, V. V. Roddatis, D. Kaseman, S. Sen, J. Dolyniuk, K. Lee, A. V. Olenev and K. Kovnir, J. Am. Chem. Soc., 2013, 135, 12313–12323 CrossRef CAS PubMed.
-
G. S. Nolas, The Physics and Chemistry of Inorganic Clathrates, Springer, Philadelphia, New York, 2014 Search PubMed.
- J. Dolyniuk, B. Owens-Baird, J. Wang, J. V. Zaikina and K. Kovnir, Mater. Sci. Eng., R, 2016, 108, 1–46 CrossRef.
- J. Dolyniuk, J. Wang, K. Lee and K. Kovnir, Chem. Mater., 2015, 27, 4476–4484 CrossRef CAS.
- S. Bobev and S. C. Sevov, J. Solid State Chem., 2000, 153, 92–105 CrossRef CAS.
- K. A. Kovnir and A. V. Shevelkov, Russ. Chem. Rev., 2004, 73, 923–938 CrossRef CAS.
- A. V. Shevelkov and K. Kovnir, Struct. Bonding, 2011, 139, 97–142 CrossRef CAS.
- B. B. Iversen, A. E. C. Palmqvist, D. E. Cox, G. S. Nolas, G. D. Stucky, N. P. Blake and H. Metiu, J. Solid State Chem., 2000, 149, 455–458 CrossRef CAS.
- H. Kleinke, Chem. Mater., 2010, 22, 604–611 CrossRef CAS.
- J. Dunner and A. Mewis, Z. Anorg. Allg. Chem., 1995, 621, 191–196 CrossRef.
- J. Dolyniuk, P. S. Whitfield, K. Lee, O. I. Lebedev and K. Kovnir, Chem. Sci., 2017, 8, 3650–3659 RSC.
- J. Dolyniuk, J. V. Zaikina, D. C. Kaseman, S. Sen and K. Kovnir, Angew. Chem., Int. Ed., 2017, 56, 2418–2422 CrossRef CAS PubMed.
-
Bruker APEX2, Bruker AXS Inc., Madison, WI, 2005 Search PubMed.
- G. M. Sheldrick, Acta Crystallogr., Sect. A: Found. Crystallogr., 2008, 64, 112–122 CrossRef CAS PubMed.
- T. Proffen and S. J. L. Billinge, J. Appl. Crystallogr., 1999, 32, 572–575 CrossRef CAS.
- C. L. Farrow, P. Juhas, J. W. Liu, D. Bryndin, E. S. Božin, J. Bloch, T. Proffen and S. J. L. Billinge, J. Phys.: Condens. Matter, 2007, 19, 335219 CrossRef CAS PubMed.
- C. Wood, D. Zoltan and G. Stapfer, Rev. Sci. Instrum., 1985, 56, 719–722 CrossRef CAS.
- K. A. Borup, E. S. Toberer, L. D. Zoltan, G. Nakatsukasa, M. Errico, J. P. Fleurial, B. B. Iversen and G. J. Snyder, Rev. Sci. Instrum., 2012, 83, 123902 CrossRef PubMed.
- D. Huo, S. Yuji Muro and T. Takabatake, Appl. Phys. Lett., 2003, 82, 2640 CrossRef CAS.
- K. Kovnir, U. Stockert, S. Budnyk, Yu. Prots, M. Baitinger, S. Paschen, A. V. Shevelkov and Y. Grin, Inorg. Chem., 2011, 50, 10387–10396 CrossRef CAS PubMed.
- M. Christensen, N. Lock, J. Overgaard and B. B. Iversen, J. Am. Chem. Soc., 2006, 128, 15657–15665 CrossRef CAS PubMed.
- S. E. Latturner, J. D. Bryan, N. Blake, H. Metiu and G. D. Stucky, Inorg. Chem., 2002, 41, 3956–3961 CrossRef CAS PubMed.
- S. Johnson, A. Bentien, G. K. H. Madsen, B. B. Iversen and M. Nygren, Chem. Mater., 2006, 18, 4633–4642 CrossRef.
- J. V. Zaikina, K. A. Kovnir, U. Burkhardt, W. Schnelle, F. Haarmann, U. Schwarz, Y. Grin and A. V. Shevelkov, Inorg. Chem., 2009, 48, 3720–3730 CrossRef CAS PubMed.
- A. Kaltzoglou, T. F. Fässler, M. Christensen, S. Johnsen, B. Iversen, I. Presniakov, A. Sobolev and A. Shevelkov, J. Mater. Chem., 2008, 18, 5630–5637 RSC.
- W. Carrillo-Cabrera, S. Budnyk, Y. Prots and Y. Grin, Z. Anorg. Allg. Chem., 2004, 630, 2267–2276 CrossRef CAS.
- F. Dubois and T. F. Fssler, J. Am. Chem. Soc., 2005, 127, 3264–3265 CrossRef CAS PubMed.
- J. V. Zaikina, K. A. Kovnir, F. Haarmann, W. Schnelle, U. Burkhardt, H. Borrmann, U. Schwarz, Y. Grin and A. V. Shevelkov, Chem.–Eur. J., 2008, 14, 5414–5422 CrossRef CAS PubMed.
- K. A. Kovnir, J. V. Zaikina, L. N. Reshetova, A. V. Olenev, E. V. Dikarev and A. V. Shevelkov, Inorg. Chem., 2004, 43, 3230–3236 CrossRef CAS PubMed.
- Y. Liu, L.-M. Wu, L.-H. Li, S.-W. Du, J. D. Corbett and L. Chen, Angew. Chem., Int. Ed., 2009, 48, 5305–5308 CrossRef CAS PubMed.
- H. He, A. Zevalkink, Z. M. Gibbs, G. J. Snyder and S. Bobev, Chem. Mater., 2012, 24, 3596–3603 CrossRef CAS.
- X. Shi, J. Yang, S. Bai, J. Yang, H. Wang, M. Chi, J. R. Salvador, W. Zhang, L. Chen and W. Wong-Ng, Adv. Funct. Mater., 2010, 20, 755–763 CrossRef CAS.
- S. Deng, X. Tang, P. Li and Q. Zhang, J. Appl. Phys., 2008, 103, 073503 CrossRef.
- D. Cederkrantz, A. Saramat, G. J. Snyder and A. E. C. Palmqvist, J. Appl. Phys., 2009, 106, 074509 CrossRef.
- L.-H. Wang and L.-S. Chang, J. Alloys Compd., 2017, 722, 644–650 CrossRef CAS.
- G. S. Nolas, J. L. Cohn, J. S. Dyck, C. Uher and J. Yang, Phys. Rev. B: Condens. Matter Mater. Phys., 2002, 65, 165201 CrossRef.
- G. S. Nolas, B. C. Chakoumakos, B. Mahieu, G. J. Long and T. J. R. Weakley, Chem. Mater., 2000, 12, 1947–1953 CrossRef CAS.
- S. R. Brown, S. M. Kauzlarich, F. Gascoin and G. J. Snyder, Chem. Mater., 2006, 18, 1873–1877 CrossRef CAS.
- B. Poudel, Q. Hao, Y. Ma, Y. Lan, A. Minnich, B. Yu, X. Yan, D. Wang, A. Muto, D. Vashaee, X. Chen, J. Liu, M. S. Dresselhaus, G. Chen and Z. F. Ren, Science, 2008, 320, 634–638 CrossRef CAS PubMed.
- L.-D. Zhao, S.-H. Lo, Y. Zhang, H. Sun, G. J. Tan, C. Uher, C. Wolverton, V. P. Dravid and M. G. Kanatzidis, Nature, 2014, 508, 373–377 CrossRef CAS PubMed.
- A. Zevalkink, E. S. Toberer, W. G. Zeier, E. Flage-Larsen and G. J. Snyder, Energy Environ. Sci., 2011, 4, 510–518 CAS.
- W. G. Zeier, A. Zevalkink, E. Schechtel, W. Tremel and G. J. Snyder, J. Mater. Chem., 2012, 22, 9826–9830 RSC.
- H.-S. Kim, Z. M. Gibbs, Y. Tang, H. Wang and G. J. Snyder, APL Mater., 2015, 3, 041506 CrossRef.
-
J.-A. Paik, E. Brandon, T. Calliat, R. Ewell and J. P. Fleurial, Proceedings of Nuclear and Emerging Technologies for Space, 2011 Search PubMed.
- A. Zevalkink, W. G. Zeier, G. Pomrehn, E. Schechtel, W. Tremel and G. J. Snyder, Energy Environ. Sci., 2012, 5, 9121–9128 CAS.
- X. Hou, Y. Zhou, L. Wang, W. Zhang, W. Zhang and L. Chen, J. Alloys Compd., 2009, 482, 544–547 CrossRef CAS.
- E. S. Toberer, C. A. Cox, S. R. Brown, T. Ikeda, A. F. May, S. M. Kauzlarich and G. J. Snyder, Adv. Funct. Mater., 2008, 18, 2795–2800 CrossRef CAS.
- C. A. Cox, E. S. Toberer, A. A. Levchenko, S. R. Brown, G. J. Snyder, A. Navrotsky and S. M. Kauzlarich, Chem. Mater., 2009, 21, 1354–1360 CrossRef CAS.
- E. S. Toberer, S. R. Brown, T. Ikeda, S. M. Kauzlarich and G. J. Snyder, Appl. Phys. Lett., 2008, 93, 062110 CrossRef.
- Y. Hu, S. K. Bux, J. H. Grebenkemper and S. M. Kauzlarich, J. Mater. Chem. C, 2015, 3, 10566 RSC.
- Y. Pei, H. Wang and G. J. Snyder, Adv. Mater., 2012, 24, 6125–6135 CrossRef CAS PubMed.
Footnote |
† Electronic supplementary information (ESI) available: Calculated and experimental powder XRD patterns; tables with crystallographic information; SEM and EDS results; additional DSC, thermal conductivities, and ZT figures. CCDC 1568106. For ESI and crystallographic data in CIF or other electronic format see DOI: 10.1039/c7sc03482b |
|
This journal is © The Royal Society of Chemistry 2017 |
Click here to see how this site uses Cookies. View our privacy policy here.