DOI:
10.1039/C7SC00724H
(Edge Article)
Chem. Sci., 2017,
8, 3775-3780
Photoswitchable interlocked thiodiglycolamide as a cocatalyst of a chalcogeno-Baylis–Hillman reaction†
Received
15th February 2017
, Accepted 7th March 2017
First published on 7th March 2017
Abstract
En route to a photoswitchable interlocked catalyst we have proved the ability of thiodiglycolamide to act as a template in the formation of hydrogen-bonded [2]rotaxanes. X-ray diffraction studies reveal the shielding of the sulfide atom by the macrocycle. A series of molecular shuttles are described as having an isomerizable fumaramide and thiodiglycolamide binding sites for controlling the relative ring position at will. By employing these systems as photoregulated catalysts, the TiCl4-mediated chalcogeno-Morita–Baylis–Hillman reaction is tested. In the presence of the maleamide shuttle, in which the sulfide function is encapsulated by the macrocycle, a complete loss in control of the geometry of the produced aldol is observed. The E-aldol adduct is predominantly obtained when the photoisomerized fumaramide shuttle, in which the sulfide function is exposed, is used.
Introduction
Taking as model the majority of the enzymatically driven processes that are ubiquitous in nature,1 chemists are devoting much effort to the designing of catalysts with switchable activity.2 These compounds are able to control programmed multistep procedures or selectively afford synthetic targets. Among the external triggering stimuli, both light3 and chemical reactions,4 including coordination events,4c–e redox reactions,4f and changes in pH,4a,b solvent4g or temperature,4h have been employed to control the catalytic activity and the regio- and stereoselectivity of the reactions in which they are involved.2
Although the first examples of the incorporation of catalytic centers in rotaxanes5 were reported in the last decade,6 the use of interlocked molecular architectures7 as switchable catalysts is a growing topic nowadays.2,8 In this area, pH-driven molecular shuttles have been used for controlling the catalytic activity or the result of a particular reaction. Some recent examples of these systems include the control of the rate9 and the stereochemistry10 of Michael addition reactions or participation in selected chemical transformations through different activation modes.11 To the best of our knowledge, light-driven threaded systems12 programmed to modify their catalytic activity at will remain unexplored. In order to tackle this issue, herein we have designed a photo-responsive molecular shuttle that could communicate the reactivity of a nucleophilic center in one of its states, whereas the same feature remained silenced in the other state.
In this regard we have envisioned a photoswitchable interlocked catalyst by the incorporation of a sulfide functionality13 in one of the two binding sites of a hydrogen-bonded molecular shuttle5,7,8a,14 that also contains a photoisomerizable fumaramide station (Fig. 1).15 We expected that in the active mode of this catalyst, in which the sulfide is exposed to the surrounding reaction medium, the system catalyzes an organic transformation in a selective fashion (catalysis ON). This chemical behavior would drastically change by the light-promoted E to Z interconversion of the olefinic station promoting the coverage of the sulfur-based station by the macrocycle, and thus precluding its participation in the considered process (catalysis OFF).
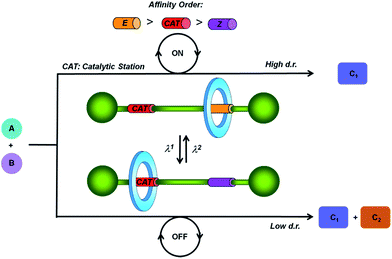 |
| Fig. 1 Representation of a photoswitchable E/Z interlocked catalyst. | |
For the catalytic model reaction we selected one of the most appealing organic transformations that is catalyzed by sulfides,16 the Morita–Baylis–Hillman (MBH) reaction. This atom-economic carbon–carbon bond forming reaction is broadly used for the functionalization of activated alkenes or alkynes by reaction with different electrophiles under the influence of a catalytic system.17
Results and discussion
Hydrogen-bonding directed assembly of thiodiglycolamide [2]rotaxanes
The formation of amide-based rotaxanes requires the use of a suitable template to maximize the efficiency of the assembly.18 In the case of tetralactam-based rotaxanes having four benzylic amide functions in the ring, a variety of structural motifs have been employed, including 1,4-dicarboxamides,19 nitrones,20 squaraines,21 organophosphorus species,22 di(acylamino)pyrididines23 and azodicarboxamides.6d,24
Thiodiglycolamides 2, that are easily obtained from commercially available thiodiglycolic acid 1 (Scheme 1), were chosen as the sulfur-containing threads. These compounds contain two carbonyl groups as hydrogen bond acceptors, two bulky groups as stoppers and an embedded sulfur atom as the future catalytic active center. Firstly, the ability of these compounds to template the assembly of benzylic amide [2]rotaxanes was assayed. The five-component clipping reaction of 2a using p-xylylenediamine and isophthaloyl chloride in the presence of triethylamine afforded the [2]rotaxane 3a. The surrogate 3b was also prepared, which bears two dibenzylamino groups as the stoppers of the interlocked species, enhancing its solubility in chlorinated solvents when compared to 3a. As we expected, both of the rotaxanes were obtained in low yields (3a, 13%; 3b, 11%) indicating a moderate affinity of the thiodiglycolamide binding site for the macrocycle.
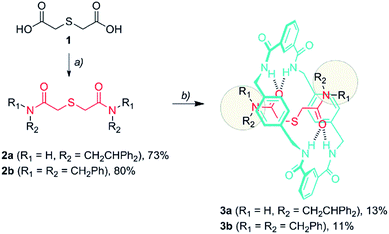 |
| Scheme 1 Synthesis of thiodiglycolamides 2 and the [2]rotaxanes 3. Reagents and conditions: (a) (i) (COCl)2, DMF (cat) and CH2Cl2; (ii) 2,2-diphenylethylamine or dibenzylamine, Et3N, CHCl3, 2a (73%) and 2b (80%), and (b) isophthaloyl dichloride, p-xylylenediamine, Et3N, CHCl3, 3a (13%), and 3b (11%). | |
Molecular structure of the hydrogen-bonded [2]rotaxane 3a in the solid state
In order to get a detailed view of the chemical environment of the active center once encapsulated by the macrocycle, we grew suitable monocrystals for X-ray diffraction analysis by slowly evaporating a solution of 3a in acetonitrile. The resulting interlocked molecular structure of 3a (Fig. 2) shows a bifurcated hydrogen bond (HB) between two of the NH groups of the macrocycle with one of the CO groups of the thiodiglycolamide thread, and a single HB between another NH group of the macrocycle with the second CO group of the thread. The CH2SCH2 skeleton between the HB acceptor of the thread adopts a folded conformation that is established by CH⋯π interactions between one of its methylenic hydrogen atoms and one of the p-xylylene groups of the tetralactam ring (3.4 Å, 86°) (Fig. S3, see ESI†). In contrast with other sulfur-containing functionalities used as templates in the building of amide based rotaxanes,13c the sulfur atom incorporated in the thiodiglycolamide 2a seems to be a mere spectator during the rotaxane formation.
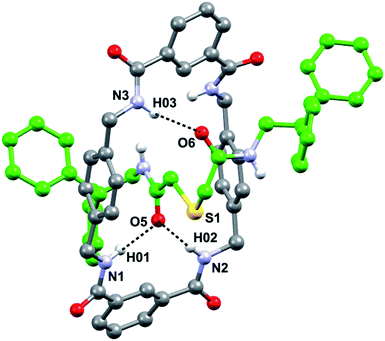 |
| Fig. 2 X-ray structure of the thiodiglycolamide [2]rotaxane 3a crystallized from CH3CN. For clarity, the carbon atoms of the macrocycle are shown in grey, the carbon atoms of the thread are shown in green, the oxygen atoms are depicted in red, the nitrogen atoms are depicted in blue, and selected hydrogen atoms are shown in white. Also for clarity, the solvent molecules have been omitted. Intramolecular hydrogen-bond lengths [Å] (and angles [deg]): O5HN1 2.204 (176.8), O5HN2 2.096 (177.5) and O6HN3 2.096 (152.6). | |
An inspection of the structure of the interlocked thiodiglycolamide 3a in the solid state reveals a reasonable shielding of the sulfur functionality which could reduce or even preclude its participation in a chemical reaction. The next step in our research was directed towards the incorporation of this binding site station into a photoswitchable molecular shuttle.
Synthesis of photoswitchable thiodiglycolamide molecular shuttles
En route to the targeted interlocked catalysts, the first synthetic step required the nucleophilic opening of anhydride 4,25 which was easily obtained from thiodiglycolic acid (1), with 2,2-diphenylethylamine or dibenzylamine to provide the carboxylic acids 5 (Scheme 2). Next, the amide formation reaction of 5 with a monoBoc protected dodecyldiamine (S1) was carried out to produce the carbamates 6. After amine deprotection, a second amide formation reaction with a fumaric acid semiamide12f,26 (S2) allowed for the incorporation of the olefinic station into the dumbbell-shaped compounds E-7 (Scheme 2).
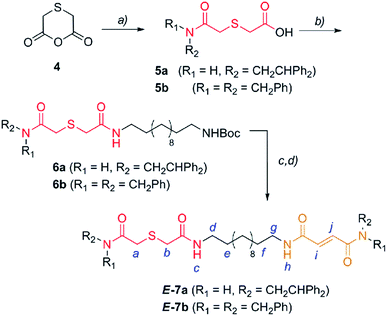 |
| Scheme 2 Synthesis of two binding site threads E-7a,b. Reagents and conditions: (a) 2,2-diphenylethylamine or dibenzylamine, pyridine, reflux, Et2O, 5a (70%) and 5b (48%); (b) 12-(tert-butoxycarbonylamino)dodecylamine (S1), EDCI, DMAP, CH2Cl2, 6a (43%) and 6b (94%); (c) CF3CO2H and CH2Cl2; and (d) (E)-4-(2,2-diphenylethylamino)-4-oxobut-2-enoic acid (S2a) or (E)-4-(dibenzylamino)-4-oxobut-2-enoic acid (S2b), EDCI, DMAP, CH2Cl2, 7a (59%) and 7b (66%). The full experimental procedure can be found in the ESI.† | |
With the sulfur containing threads E-7 in hand, we assembled the benzylic amide [2]rotaxanes E-8via a five component clipping reaction using p-xylylenediamine, isophthaloyl dichloride and triethylamine in chloroform (Scheme 3). The photoisomerization of the trans double bond of the interlocked compounds E-8 led to the corresponding maleamide counterparts Z-8. The recovery of the starting shuttle was efficiently reached via cis-to-trans isomerization promoted by irradiating at 312 nm (conditions c, Scheme 3).
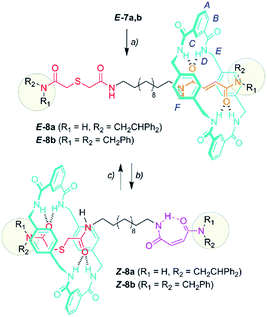 |
| Scheme 3 Synthesis of molecular shuttles 8 and the interconversion of their translational co-conformers. Reagents and conditions: (a) isophthaloyl dichloride, p-xylylenediamine, Et3N, CHCl3, E-8a (25%) and E-8b (46%); (b) 254 nm, CH2Cl2, Z-8a (49%) and Z-8b (53%); and (c) 312 nm, CH2Cl2, E-8a (46%) and E-8b (58%). The full experimental procedure can be found in the ESI.† | |
Fig. 3 displays the stacked 1H NMR spectra of threads 7b27 and rotaxanes 8b. The comparison of these spectra allows us to establish the relative location of the ring in the interlocked systems. The thiodiglycolamide signals (Ha and Hb, red) are at similar chemical shifts in both the thread and rotaxane (see the traces in Fig. 3a and b), thus proving that this station is empty in E-8b, whereas the hydrogens of the methyne fumaramide (Hi and Hj, orange) are shifted ∼1.33 ppm upfield in this rotaxane with respect to its naked thread E-7b due to the shielding effect of the xylylene aromatic rings. So, in this co-conformer the macrocycle is mainly located at the fumaramide station of E-8b.
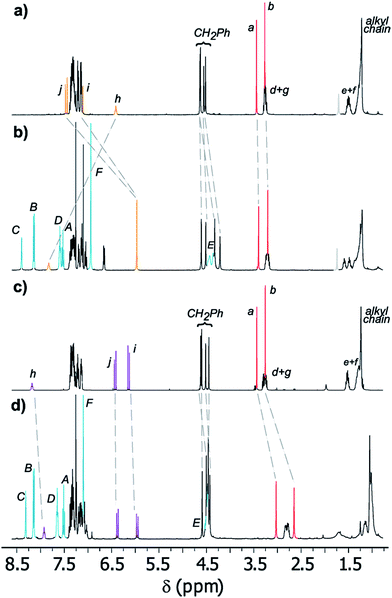 |
| Fig. 3 Partial 1H NMR spectra (400 MHz, CDCl3, 298 K) of (a) thread E-7b, (b) [2]rotaxane E-8b, (c) thread Z-7b, and (d) [2]rotaxane Z-8b. The assignments correspond to the lettering shown in Schemes 2 and 3. | |
Additionally, while the maleamide signals of Z-8b appear at similar chemical shifts to those of thread Z-7b, the thiodiglycolamide signals of Z-8b are shifted by 0.40 ppm with respect to those of Z-7b (see the traces in Fig. 3c and d), suggesting that the ring moves over to this binding site and the light-driven ring translocation from the fumaramide unit to the sulfide moiety occurs with excellent positional integrity.
Carbon–carbon bond forming reactions using thiodiglycolamide [2]rotaxanes as catalysts
Having obtained our photoswitchable sulfide-based [2]rotaxanes, we focused on the chalcogeno-Baylis–Hillman reaction developed by Kataoka et al.28 The presence of a catalytic amount of a chalcogenide (a sulfur or selenide derivative) enabled the control of the stereochemistry (cis–trans configuration) of the chloromethylene aldols obtained via TiCl4 promoted reactions between aldehydes and activated alkynes. Thus the presence of the catalytic amounts of dimethyl sulfide predominantly led to the formation of the E-isomer at short reaction times and low temperatures. In order to explore the viability of the thiodiglycolamide-based [2]rotaxanes for acting as catalysts of this transformation we tested the TiCl4-promoted Baylis–Hillman reaction between p-nitrobenzaldehyde and 3-butyn-2-one in the presence of catalytic amounts of our interlocked and non-interlocked thiodiglycolamides. First, we carried out this reaction in the presence of sulfide 2a (0.1 equiv.; Table 1, entry 1) and TiCl4 (1 equiv.) at 5 °C for 3 h affording a mixture of the geometrical isomers of α-chloromethylene aldol 9 with the olefinic E product as the major isomer (85
:
15 dr). The better solubility of 2b enhances this ratio up to 91
:
9 (Table 1, entry 2). If the single-binding site rotaxanes 3 are used as the sulfide sources, the stereochemical control is null and a practically equimolar mixture of E and Z aldols is obtained (Table 1, entries 3 and 4) as a consequence of the shielding of the sulfide fragment by the macrocycle, which precludes its participation in the reaction. The use of the interlocked catalyst E-8a affords aldol 9 in a 56
:
44 ratio in favor of the E isomer (Table 1, entry 5). When the most soluble derivative E-8b is employed, better control is obtained (80
:
20) as a result of an increase in the interlocked sulfide concentration in the reaction (Table 1, entry 6) (see Fig. S1†). Finally, a 1
:
1 mixture of both isomers of 9 are obtained in the presence of the interlocked catalyst Z-8b in which the ring is positioned over the thiodiglycolamide station (Table 1, entry 7). As expected, the diastereomeric ratios achieved in the presence of 3a, 3b and Z-8b are similar to the one obtained in the absence of the chalcogenide derivative (Table 1, entry 8). Notably, the respective sulfide-based catalyst remains unaltered at the end of these experiments, and it could be recovered for further use.
Table 1 Thiodiglycolamide-catalyzed Baylis–Hillman reaction mediated by TiCl4a
Although the reaction conversion using the two station [2]rotaxane decreased with respect to those obtained using non-interlocked catalysts, the employment of the optimized trans co-conformer E-8b allowed a maximum 80
:
20 ratio to be achieved in favor of the chloromethylene aldol E-9. The photoisomerized cis co-conformer Z-8 keeps the sulfide function in a latent state and is kinetically protected by the surrounding ring against other external reagents.8d
This example shows for the first time that the employment of photoswitchable interlocked catalysts can be useful to control the stereochemical course of a reaction. In contrast to pH-driven catalysts, this unprecedented light-responsive interlocked catalyst is enabled to work in neutral media, expanding its potential applicability to a broad variety of transformations in which these molecular shuttles can play an important role in regulating the outcome of the reactions at will.
Conclusions
In summary, we have shown that thiodiglycolamides are able to act as a template in the formation of benzylic amide rotaxanes. The molecular structure of one of these interlocked compounds in the solid state reveals that its sulfide functional group is well covered by the macrocyclic tetralactam and so is isolated from its surroundings. These findings were used to design a switchable interlocked sulfide which includes a fumaramide binding site as a photoisomerizable unit for controlling the relative ring position. Two versions of this prototype varying the number of secondary amides in the thread (and then differing in solubility in halogenated solvents) were straightforwardly synthesized from thiodiglycolic anhydride. The behavior of the sulfide function of both translational co-conformers of these molecular shuttles was assayed in a MBH type reaction promoted by TiCl4 using p-nitrobenzaldehyde and 3-butyn-2-one. With the fumaramide shuttle, in which the sulfide function is exposed, the E-aldol adduct is predominantly obtained. Using the photoisomerized shuttle, in which the sulfide function is encapsulated by the macrocycle, a complete loss of diastereoselectivity is observed.
Acknowledgements
This work was supported by the MINECO (CTQ2014-56887 P) with joint financing by FEDER Funds from the European Union, and Fundacion Seneca-CARM (Project 19240/PI/14). A. M.-C. also thanks the MINECO (Contract No. FPDI-2013-16623) for his postdoctoral contract.
Notes and references
-
(a) E. Kuah, S. Toh, J. Yee, Q. Ma and Z. Gao, Chem.–Eur. J., 2016, 22, 8404–8430 CrossRef CAS PubMed;
(b) R. Wolfenden and M. J. Snider, Acc. Chem. Res., 2001, 34, 938–945 CrossRef CAS PubMed;
(c) M. Garcia-Viloca, J. Gao, M. Karplus and D. G. Truhlar, Science, 2004, 303, 186–195 CrossRef CAS PubMed.
- V. Blanco, D. A. Leigh and V. Marcos, Chem. Soc. Rev., 2015, 44, 5341–5370 RSC.
-
(a) R. S. Stoll and S. Hecht, Angew. Chem., Int. Ed., 2010, 49, 5054–5075 CrossRef CAS PubMed;
(b) J. Wang and B. L. Feringa, Science, 2011, 331, 1429–1432 CrossRef CAS PubMed;
(c) D. Wilson and N. R. Branda, Angew. Chem., Int. Ed., 2012, 51, 5431–5434 CrossRef CAS PubMed;
(d) A. Nojiri, N. Kumagai and M. Shibasaki, Chem. Commun., 2013, 49, 4628–4630 RSC;
(e) L. Osorio-Planes, C. Rodriguez-Escrich and M. A. Pericas, Org. Lett., 2014, 16, 1704–1707 CrossRef CAS PubMed;
(f) D. Zhao, T. M. Neubauer and B. L. Feringa, Nat. Commun., 2015, 6, 6652 CrossRef CAS PubMed;
(g) Q. Zhang, W.-Z. Wang, J.-J. Yu, D.-H. Qu and H. Tian, Adv. Mater., 2017, 29, 1604948 CrossRef PubMed.
- pH:
(a) X. Tian, C. Cassani, Y. Liu, A. Moran, A. Urakawa, P. Galzerano, E. Arceo and P. Melchiorre, J. Am. Chem. Soc., 2011, 133, 17934–17941 CrossRef CAS PubMed;
(b) S. A. Moteki, J. Han, S. Arimitsu, M. Akakura, K. Nakayama and K. Maruoka, Angew. Chem., Int. Ed., 2012, 51, 1187–1190 CrossRef CAS PubMed, Coordination;
(c) S. De, S. Pramanik and M. Schmittel, Angew. Chem., Int. Ed., 2014, 53, 14255–14259 CrossRef CAS PubMed;
(d) C. M. McGuirk, J. Mendez-Arroyo, A. M. Lifschitz and C. A. Mirkin, J. Am. Chem. Soc., 2014, 136, 16594–16601 Search PubMed;
(e) J. T. Foy, D. Ray and I. Aprahamian, Chem. Sci., 2015, 6, 209–213 RSC, Redox;
(f) P. Neumann, H. Dib, A.-M. Caminade and E. Hey-Hawkins, Angew. Chem., Int. Ed., 2015, 54, 311–314 CrossRef CAS PubMed, Solvent;
(g) Y. Sohtome, S. Tanaka, K. Takada, T. Yamaguchi and K. Nagasawa, Angew. Chem., Int. Ed., 2010, 49, 9254–9257 Search PubMed, Temperature;
(h) G. Storch and O. Trapp, Angew. Chem., Int. Ed., 2015, 54, 3580–3586 CrossRef CAS PubMed.
- M. Xue, Y. Yang, X. Chi, X. Yan and F. Huang, Chem. Rev., 2015, 115, 7398–7501 CrossRef CAS PubMed.
- For some examples see:
(a) P. Thordarson, E. J. A. Bijsterveld, A. E. Rowan and R. J. M. Nolte, Nature, 2003, 424, 915–918 CrossRef CAS PubMed;
(b) Y. Tachibana, N. Kihara and T. Takata, J. Am. Chem. Soc., 2004, 126, 3438–3439 CrossRef CAS PubMed;
(c) G. Hattori, T. Hori, Y. Miyake and Y. Nishibayashi, J. Am. Chem. Soc., 2007, 129, 12930–12931 CrossRef CAS PubMed;
(d) J. Berna, M. Alajarin and R.-A. Orenes, J. Am. Chem. Soc., 2010, 132, 10741–10747 CrossRef CAS PubMed;
(e) B. Lewandowski, G. De Bo, J. W. Ward, M. Papmeyer, S. Kuschel, M. J. Aldegunde, P. M. E. Gramlich, D. Heckmann, S. M. Goldup, D. M. D’Souza, A. E. Fernandes and D. A. Leigh, Science, 2013, 339, 189–193 CrossRef CAS PubMed;
(f) M. Galli, J. E. M. Lewis and S. M. Goldup, Angew. Chem., Int. Ed., 2015, 54, 13545–13549 CrossRef CAS PubMed;
(g) S. Hoekman, M. O. Kitching, D. A. Leigh, M. Papmeyer and D. Roke, J. Am. Chem. Soc., 2015, 137, 7656–7659 CrossRef CAS PubMed;
(h) Y. Cakmak, S. Erbas-Cakmak and D. A. Leigh, J. Am. Chem. Soc., 2016, 138, 1749–1751 CrossRef CAS PubMed.
-
(a)
A. Credi, S. Silvi and M. Venturi, Molecular Machines and Motors: Recent Advances and Perspectives, Springer, Switzerland, 2014 Search PubMed;
(b)
C. J. Bruns and J. F. Stoddart, The Nature of the Mechanical Bond: From Molecules to Machines, Wiley, New York, 2016 Search PubMed.
-
(a) D. A. Leigh, V. Marcos and M. R. Wilson, ACS Catal., 2014, 4, 4490–4497 CrossRef CAS;
(b) S. Erbas-Cakmak, D. A. Leigh, C. T. McTernan and A. L. Nussbaumer, Chem. Rev., 2015, 115, 10081–10206 CrossRef CAS PubMed;
(c) T. Pan and J. Liu, ChemPhysChem, 2016, 17, 1752–1758 CrossRef CAS PubMed;
(d) J. E. M. Lewis, M. Galli and S. M. Goldup, Chem. Commun., 2017, 53, 298–312 RSC.
- V. Blanco, A. Carlone, K. D. Hänni, D. A. Leigh and B. Lewandowski, Angew. Chem., Int. Ed., 2012, 51, 5166–5169 CrossRef CAS PubMed.
- V. Blanco, D. A. Leigh, V. Marcos, J. A. Morales-Serna and A. L. Nussbaumer, J. Am. Chem. Soc., 2014, 136, 4905–4908 CrossRef CAS PubMed.
-
(a) V. Blanco, D. A. Leigh, U. Lewandowska, B. Lewandowski and V. Marcos, J. Am. Chem. Soc., 2014, 136, 15775–15780 CrossRef CAS PubMed;
(b) J. Beswick, V. Blanco, G. De Bo, D. A. Leigh, U. Lewandowska, B. Lewandowski and K. Mishiro, Chem. Sci., 2015, 6, 140–143 RSC.
-
(a) D.-H. Qu, Q.-C. Wang and H. Tian, Angew. Chem., Int. Ed., 2005, 44, 5296–5299 CrossRef CAS PubMed;
(b) V. Balzani, A. Credi and M. Venturi, Chem. Soc. Rev., 2009, 38, 1542–1550 RSC;
(c) D.-H. Qu, Q.-C. Wang, Q.-W. Zhang, X. Ma and H. Tian, Chem. Rev., 2015, 115, 7543–7588 CrossRef CAS PubMed;
(d) G. Ragazzon, M. Baroncini, S. Silvi, M. Venturi and A. Credi, Nat. Nanotechnol., 2015, 10, 70–75 CrossRef CAS PubMed;
(e) C. Cheng, P. R. McGonigal, J. F. Stoddart and R. D. Astumian, ACS Nano, 2015, 9, 8672–8688 CrossRef CAS PubMed;
(f) A. Martinez-Cuezva, S. Valero-Moya, M. Alajarin and J. Berna, Chem. Commun., 2015, 51, 14501–14504 RSC;
(g) K. Iwaso, Y. Takashima and A. Harada, Nat. Chem., 2016, 8, 625–632 CrossRef CAS PubMed;
(h) F. B. Schwarz, T. Heinrich, A. Lippitz, W. E. S. Unger and C. A. Schalley, Chem. Commun., 2016, 52, 14458–14461 RSC.
-
(a) S. Saito, E. Takahashi and K. Nakazono, Org. Lett., 2006, 8, 5133–5136 CrossRef CAS PubMed;
(b) H. Zheng, Y. Li, C. Zhou, Y. Li, W. Yang, W. Zhou, Z. Zuo and H. Liu, Chem.–Eur. J., 2011, 17, 2160–2167 CrossRef CAS PubMed;
(c) A. Altieri, V. Aucagne, R. Carrillo, G. J. Clarkson, D. M. D’Souza, J. A. Dunnett, D. A. Leigh and K. M. Mullen, Chem. Sci., 2011, 2, 1922–1928 RSC;
(d) E. M. Peck, C. G. Collins and B. D. Smith, Org. Lett., 2013, 15, 2762–2765 CrossRef CAS PubMed.
-
(a) L. Liu, Y. Liu, P. Liu, J. Wu, Y. Guan, X. Hu, C. Lin, Y. Yang, X. Sun, J. Ma and L. Wang, Chem. Sci., 2013, 4, 1701–1706 Search PubMed;
(b) C. G. Collins, E. M. Peck, P. J. Kramer and B. D. Smith, Chem. Sci., 2013, 4, 2557–2563 RSC;
(c) M. J. Langton and P. D. Beer, Acc. Chem. Res., 2014, 47, 1935–1949 CrossRef CAS PubMed;
(d) K. Zhu, C. A. O’Keefe, V. N. Vukotic, R. W. Schurko and S. J. Loeb, Nat. Chem., 2015, 7, 514–519 CrossRef CAS PubMed;
(e) P. Wei, X. Yan, T. R. Cook, X. Ji, P. J. Stang and F. Huang, ACS Macro Lett., 2016, 5, 671–675 CrossRef CAS;
(f) D. C. Jagesar, P. G. Wiering, E. R. Kay, D. A. Leigh and A. M. Brouwer, ChemPhysChem, 2016, 17, 1902–1912 CrossRef CAS PubMed;
(g) T. Legigan, B. Riss-Yaw, C. Clavel and F. Coutrot, Chem.–Eur. J., 2016, 22, 8835–8847 CrossRef CAS PubMed;
(h) X. Ma, J. Zhang, J. Cao, X. Yao, T. Cao, Y. Gong, C. Zhao and H. Tian, Chem. Sci., 2016, 7, 4582–4588 RSC;
(i) G. Ragazzon, A. Credi and B. Colasson, Chem.–Eur. J., 2017, 23, 2149–2156 CrossRef CAS PubMed;
(j) V. N. Vukotic, K. Zhu, G. Baggi and S. J. Loeb, Angew. Chem., Int. Ed., 2017 DOI:10.1002/anie.201612549.
-
(a) A. Altieri, G. Bottari, F. Dehez, D. A. Leigh, J. K. Y. Wong and F. Zerbetto, Angew. Chem., Int. Ed., 2003, 42, 2296–2300 CrossRef CAS PubMed;
(b) G. Bottari, D. A. Leigh and E. M. Perez, J. Am. Chem. Soc., 2003, 125, 13360–13361 CrossRef CAS PubMed;
(c) E. M. Perez, D. T. F. Dryden, D. A. Leigh, G. Teobaldi and F. Zerbetto, J. Am. Chem. Soc., 2004, 126, 12210–12211 CrossRef CAS PubMed.
- E. M. McGarrigle, E. L. Myers, O. Illa, M. A. Shaw, S. L. Riches and V. K. Aggarwal, Chem. Rev., 2007, 107, 5841–5883 CrossRef CAS PubMed.
-
(a)
E. Ciganek, in Organic Reactions, ed. L. A. Paquette, Wiley, New York, 1997, vol. 51, pp. 201–350 Search PubMed;
(b) V. Declerck, J. Martinez and F. Lamaty, Chem. Rev., 2009, 109, 1–48 CrossRef CAS PubMed;
(c) D. Basavaiah, B. S. Reddy and S. S. Badsara, Chem. Rev., 2010, 110, 5447–5674 CrossRef CAS PubMed;
(d) D. Basavaiah and G. Veeraraghavaiah, Chem. Soc. Rev., 2012, 41, 68–78 RSC;
(e) T. Y. Liu, M. Xie and Y. C. Chen, Chem. Soc. Rev., 2012, 41, 4101–4112 RSC.
-
(a) C. A. Schalley, T. Weilandt, J. Brüggemann and F. Vögtle, Top. Curr. Chem., 2004, 248, 141–200 CrossRef;
(b) C. A. Schalley, W. Reckien, S. Peyerimhoff, B. Baytekin and F. Vögtle, Chem.–Eur. J., 2004, 10, 4777–4789 CrossRef CAS PubMed;
(c) E. R. Kay, D. A. Leigh and F. Zerbetto, Angew. Chem., Int. Ed., 2007, 46, 72–191 Search PubMed;
(d) G. T. Spence and P. D. Beer, Acc. Chem. Res., 2013, 46, 571–586 CrossRef CAS PubMed;
(e) A. Tron, P. J. Thornton, B. Kauffmann, J. H. R. Tucker and N. D. McClenaghan, Supramol. Chem., 2016, 28, 733–741 CrossRef CAS;
(f) A. Vidonne, T. Kosikova and D. Philp, Chem. Sci., 2016, 7, 2592–2603 RSC.
-
(a) J. Berna, G. Bottari, D. A. Leigh and E. M. Perez, Pure Appl. Chem., 2007, 79, 39–54 CrossRef CAS;
(b) J. Berna, M. Alajarin, J. S. Martinez-Espin, L. Buriol, M. A. P. Martins and R.-A. Orenes, Chem. Commun., 2012, 48, 5677–5679 RSC;
(c) M. R. Panman, B. H. Bakker, D. den Uyl, E. R. Kay, D. A. Leigh, W. J. Buma, A. M. Brouwer, J. A. J. Geenevasen and S. Woutersen, Nat. Chem., 2013, 5, 929–934 CrossRef CAS PubMed;
(d) A. Martinez-Cuezva, C. Lopez-Leonardo, D. Bautista, M. Alajarin and J. Berna, J. Am. Chem. Soc., 2016, 138, 8726–8729 CrossRef CAS PubMed.
-
(a) V. Bermudez, N. Capron, T. Gase, F. G. Gatti, F. Kajzar, D. A. Leigh, F. Zerbetto and S. Zhang, Nature, 2000, 406, 608–611 CrossRef CAS PubMed;
(b) D. M. D’Souza, D. A. Leigh, L. Mottier, K. M. Mullen, F. Paolucci, S. J. Teat and S. Zhang, J. Am. Chem. Soc., 2010, 132, 9465–9470 CrossRef PubMed.
-
(a) E. Arunkumar, C. C. Forbes, B. C. Noll and B. D. Smith, J. Am. Chem. Soc., 2005, 127, 3288–3289 CrossRef CAS PubMed;
(b) J. J. Gassensmith, J. M. Baumes and B. D. Smith, Chem. Commun., 2009, 6329–6338 RSC;
(c) C. G. Collins, J. M. Lee, A. G. Oliver, O. Wiest and B. D. Smith, J. Org. Chem., 2014, 79, 1120–1130 CrossRef CAS PubMed.
- R. Ahmed, A. Altieri, D. M. D́Souza, D. A. Leigh, K. M. Mullen, M. Papmeyer, A. M. Z. Slawin, J. K. Y. Wong and J. D. Woollins, J. Am. Chem. Soc., 2011, 133, 12304–12310 CrossRef CAS PubMed.
-
(a) A. Martinez-Cuezva, J. Berna, R.-A. Orenes, A. Pastor and M. Alajarin, Angew. Chem., Int. Ed., 2014, 53, 6762–6767 CrossRef CAS PubMed;
(b) A. Martinez-Cuezva, A. Pastor, G. Cioncoloni, R.-A. Orenes, M. Alajarin, M. D. Symes and J. Berna, Chem. Sci., 2015, 6, 3087–3094 RSC;
(c) A. Martinez-Cuezva, F. Carro-Guillen, A. Pastor, M. Marin-Luna, R.-A. Orenes, M. Alajarin and J. Berna, ChemPhysChem, 2016, 17, 1920–1926 CrossRef CAS PubMed;
(d) A. Tron, I. Pianet, A. Martinez-Cuezva, J. H. R. Tucker, L. Pisciottani, M. Alajarin, J. Berna and N. D. McClenaghan, Org. Lett., 2017, 19, 154–157 CrossRef CAS PubMed.
- J. Berna, M. Alajarin, C. Marin-Rodriguez and C. Franco-Pujante, Chem. Sci., 2012, 3, 2314–2320 RSC.
- Y. Nagao, S. Miyamoto, K. Hayashi, A. Mihira and S. Sano, Tetrahedron Lett., 2002, 43, 1519–1522 CrossRef CAS.
- A. Carlone, S. M. Goldup, N. Lebrasseur, D. A. Leigh and A. Wilson, J. Am. Chem. Soc., 2012, 134, 8321–8323 CrossRef CAS PubMed.
- The corresponding Z isomers of threads 7 were obtained for an analytical purpose and their syntheses are described in the ESI.†.
-
(a) T. Kataoka, H. Kinoshita, S. Kinoshita, T. Iwamura and S.-I. Watanabe, Angew. Chem., Int. Ed., 2000, 39, 2358–2360 CrossRef CAS;
(b) S. Kinoshita, H. Kinoshita, T. Iwamura, S.-I. Watanabe and T. Kataoka, Chem.–Eur. J., 2003, 9, 1496–1502 CrossRef CAS PubMed;
(c) H. Kinoshita, S. Kinoshita, Y. Munechika, T. Iwamura, S.-I. Watanabe and T. Kataoka, Eur. J. Org. Chem., 2003, 4852–4861 CrossRef CAS.
Footnote |
† Electronic supplementary information (ESI) available: Experimental procedures, spectroscopic and mass spectrometry data for all of the new compounds, and the full crystallographic details of 3a. CCDC 1532346. For ESI and crystallographic data in CIF or other electronic format see DOI: 10.1039/c7sc00724h |
|
This journal is © The Royal Society of Chemistry 2017 |
Click here to see how this site uses Cookies. View our privacy policy here.