DOI:
10.1039/C7RA08010G
(Paper)
RSC Adv., 2017,
7, 43798-43811
Synthesis and characterization of a ZnMn2O4 nanostructure as a chemical nanosensor: a facile and new approach for colorimetric determination of omeprazole and lansoprazole drugs
Received
20th July 2017
, Accepted 5th September 2017
First published on 11th September 2017
Abstract
We have developed a facile method for preparation of ZnMn2O4 nanostructures via an auto-combustion method using various fuels: urea, glycine and L-alanine. The type of fuel and pH of the combustion media have a significant effect on the combustion products. Glycine fuel generated spinel ZnMn2O4 on combustion, and calcination of the sample produced pure spinel ZnMn2O4 nanoparticles with an average crystallite size of 19 nm. Whereas, other fuels generated multiphase compounds on combustion. The products were elucidated by means of XRD, FE-SEM, EDS, TEM, FT-IR, and UV-Vis diffuse reflectance spectra. The as-fabricated product was applied to construct a novel chemical nanosensor for the determination of omeprazole and lansoprazole drugs. Different factors influencing the colorimetric determination of the drugs were examined such as contact time, temperature, initial drug concentration, and ZnMn2O4 dose. The proposed chemical nanosensor revealed high sensitivity, low detection limit, and a relatively wide linear range (0.80–8.0 and 0.80–8.8 μg mL−1 at λmax 454 nm for omeprazole and lansoprazole, respectively).
1. Introduction
A growing interest in spinel metal oxide nanostructures; AB2O4 (A and B = metal), has been observed in the past few years.1–4 This intensive interest is due to the unique physical and chemical properties that these oxides possess, which make them suitable for various applications.5–9 Among these spinel nanostructures, nano-sized zinc manganite (ZnMn2O4) has received a considerable attention due to its various applications such as in sensors, electrodes, lithium rechargeable batteries, specific memory devices, thermistors, and catalysis.10–14 Therefore, many research groups have devoted their efforts to synthesizing spinel ZnMn2O4 nanostructures. In this light, ZnMn2O4 nanostructures with different morphologies and particle sizes have been prepared using various routes such as pyrolysis, solvothermal, electrospinning, hydrothermal, sol–gel, and the solid state method.15–18 However, some of these techniques are time-consuming, relatively complicated and expensive, as well as they include sophisticated instruments. Searching for a facile, inexpensive, and less time-consuming process for the production of ZnMn2O4 nanostructures is still a challenge. Besides, combustion process can fulfill these requirements since it is an inexpensive, simple, short time-consuming, and scalable procedure.19–21 In addition, this method produces porous materials with high surface areas owing to the evolution of gasses during the combustion process.19–21 In this respect, reports on the synthesis of ZnMn2O4 nanostructures using combustion methods are still limited.22,23 However, the combustion processes were not extensively investigated in those reports. And the reported preparations applied higher temperatures, during the calcination steps, and produced nanoparticles with larger crystallite sizes in comparison to our current investigation, as will be explained later in the Results and discussion section.
On the other hand, lansoprazole; C16H14F3N3O2S, 2-[[[3-methyl-4-(2,2,2-trifluoroethoxy)-2-pyridinyl]methyl]sulphinyl]-1H-benzimidazole, is a proton pump inhibitor, and it is effectively used for treatment of gastric ulcers and duodenal.24 This drug is a chemosensitizing cytotoxic, and it can treat various human tumor cells.25 In addition, omeprazole; C17H19N3O3S, 6-methoxy-2-[[(4-methoxy-3,5-dimethyl-2-pyridinyl)methyl]sulphinyl]-1H-benzimidazole, is a proton pump inhibitor which can be used for treatment of symptomatic gastro-esophageal reflux, gastroduodenal ulcers, and Zollinger–Ellison syndrome.26,27 This drug can enhance the oral digoxin bioavailability by decreasing the acid production in the stomach and increasing the gastric permeability to digoxin.26 Some methods have been developed for quantitative determination of lansoprazole and omeprazole drugs in formulations and biological fluids such as HPLC,28,29 capillary electrophoresis,30,31 spectrophotometry,32 and voltammetry.33,34 However, the demand for a simple, reliable, and inexpensive analytical assay of the aforementioned drugs is growing due to their importance. Additionally, it is reported that organic sulfide compounds can be readily converted into sulfoxide (R2SO) and/or sulfone (R2SO2) compounds by oxidation.35,36
Based on the approach presented, we have herein developed a facile synthetic method for the preparation of ZnMn2O4 nanoparticles via an auto-combustion method. Therefore, we have investigated different parameters influencing the product species and their crystallite sizes such as fuel type and pH of the combustion media. Moreover, we have developed, for the first time, a simple, accurate, and sensitive colorimetric determination method for omeprazole and lansoprazole drugs in their pharmaceutical formulations based on the as-prepared ZnMn2O4 nanostructure as a chemical sensor.
2. Experimental
2.1. Materials and reagents
Zinc nitrate (Zn(NO3)2·6H2O), manganese nitrate (Mn(NO3)2·4H2O), potassium chloride (KCl), L-alanine (CH3CH(NH2)COOH), glycine (NH2CH2COOH), urea (NH2CONH2), were provided by Sigma-Aldrich Company. Nitric acid (HNO3) was supplied by Carlo Erba Company, France. Ammonia solution 33% (NH4OH) was purchased from El Nasr Pharmaceutical Chemicals Company (ADWIC) – Company, Egypt. Lansoprazole and omeprazole (Scheme 1) were kindly provided by El Arabeya Company for Pharmaceutical and Chemical Industries, Egypt. All other reagents and chemicals used, as received without any further purification, were of analytical grade.
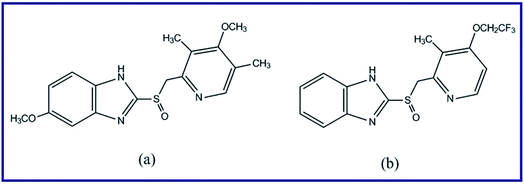 |
| Scheme 1 Chemical structures of omeprazole (a) and lansoprazole (b) drugs. | |
2.2. Fabrication of ZnMn2O4 nanostructures (chemo-sensors) using different fuels
We have proposed an auto-combustion method to prepare ZnMn2O4 nanoparticles employing three different fuels: L-alanine, glycine and urea, and the as-prepared ZnMn2O4 products will be denoted as A, G, and U, respectively. It is noteworthy that in the present combustion method, the stoichiometric compositions of the redox mixtures are calculated based on that the total reducing (F) valency of the fuel and the total oxidizing (O) valency of the mixed metal nitrate salts verify that Φc = (F/O) = 1 (where, Φc is the equivalence ratio).21,37 This was carried out to release the maximum quantity of heat from the combustion process. In a typical procedure: zinc nitrate (3.00 g, 10.08 mmol, 1.00 eq.) and manganese nitrate (5.06 g, 20.02 mmol, 2.00 eq.) were dissolved in 40 mL bi-distilled water. Then, a glycine aqueous solution; 40 mL, (2.52 g, 33.57 mmol, 3.33 eq.) was added to the stirring mixed zinc/manganese nitrate aqueous solution. The reaction blend pH was adjusted using ammonia solution (1
:
1) to pH 6. The reaction blend was then stirred at 80 °C until it turned into a viscous liquid. After that, the temperature of the reaction mixture was increased to 300 °C, while a vigorous self-propagating combustion reaction took place and lasted for ca. 5 min. The as-burnt material (G6) was ground then calcined at 500 °C for 2 h producing ZnMn2O4 products denoted as G6_500. This procedure was repeated using glycine fuel at different pH values such as 3 and 10 using nitric acid or ammonia solution (1
:
1), producing products labelled G3 and G10, respectively. Besides, we synthesized ZnMn2O4 products using similar combustion procedure and calcination temperature, while different fuels such as urea and L-alanine were utilized and the corresponding products were labelled as U and A, respectively. In addition, the aforementioned combustion and calcination steps were repeated while pH of the combustion media was adjusted at 3, 6 or 10 using nitric acid or ammonia solution, respectively. And the produced ZnMn2O4 products were referred to as U3, U6, U10, A3, A6 and A10, according to the corresponding fuel and pH.
2.3. Materials characterization
The composition and phase purity of the as-fabricated products were analyzed utilizing powder X-ray diffraction (XRD) patterns measured by 18 kW diffractometer (Bruker; model D8 Advance) equipped with a monochromated Cu-Kα radiation (λ = 1.54178 Å). Morphologies of the as-fabricated ZnMn2O4 products were examined using a field emission scanning electron microscope (FE-SEM) connected with a microscope (FE-SEM) (JEOL JSM-6500F). And TEM images of the as-fabricated ZnMn2O4 products were taken using a high-resolution transmission electron microscope (HR-TEM) (JEM-2100) at 200 kV accelerating voltage. The functional groups of the as-fabricated ZnMn2O4 products were determined by collecting their FT-IR spectra in the range of 4000–400 cm−1 employing an FT-IR spectrometer (Thermo Scientific; model Nicolet iS10) utilizing KBr pellets. The UV-Visible spectra of the drug solutions, under investigation, were measured on an UV-Vis spectrophotometer (Jasco, model v670).
2.4. Colorimetric determination of lansoprazole and omeprazole drugs
For the determination of lansoprazole and omeprazole drugs with an initial concentration of 10 mg L−1 (dissolved in ethanol), 0.05 g of the as-prepared ZnMn2O4 chemosensor was added to 20 mL of the prepared drug solution, and the pH value of the solution was adjusted using 0.5 M HNO3 or NaOH solutions. After that, the volume of the reaction mixture was adjusted to 25 mL by the addition of bi-distilled water, and the mixture was then allowed to stir for a specific time. Notably, the color of the drug solution after addition of the chemosensor nanomaterial turned into yellow. Different parameters influencing the developing of that yellow color have been investigated such as contact time, pH, ZnMn2O4 dose, initial drug concentration, and temperature. The absorbance spectra of the supernatant were measured employing an UV-Vis spectrophotometer at λmax = 454 nm, after separation of the suspension by centrifugation. Interestingly, the order of addition does not influence the accuracy, sensitivity, and reproducibility of the present developed method.
3. Results and discussion
3.1. Auto-combustion fabrication and characterization of ZnMn2O4 chemosensor
In our earlier study, we have reported on the preparation of MgAl2O4, MgO, and TiO2 nanostructures via a combustion procedure using metal nitrate salts and different fuels.19–21 And we concluded that the fuel type and equivalence ratio (Φc) value influenced the crystallite sizes of the products. However, we have not examined the effect of pH of the combustion media on the combustion process. Therefore, in this light, we have explored the influence of fuel type and combustion media pH on the combustion of zinc/manganese nitrate (oxidant). As we will explain later, the type and amount of the fuel, as well as the pH value of the combustion media have a significant impact on the combustion products, their morphologies, and their crystal sizes. Hence, the combustion reactions between zinc nitrate/manganese nitrate and a fuel such as glycine, L-alanine, or urea, were carried out at different pH values and at fuel-to-oxidant equivalence ratio (Φc) of 1. The produced ZnMn2O4 nanostructure obtained at the optimum conditions was then applied to colorimetric determination of omeprazole and lansoprazole drugs. The proposed combustion reactions are presented in Scheme 2. The combustion and calcined products were analyzed using XRD, FR-IR, SEM, UV-Vis reflectance, and TEM techniques.
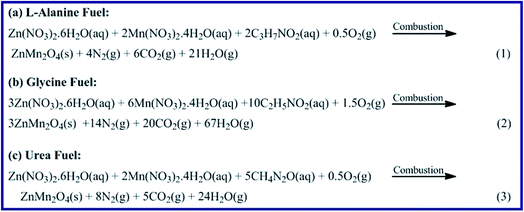 |
| Scheme 2 The proposed combustion reactions of zinc and manganese nitrates with L-alanine, glycine, and urea fuels. | |
3.1.1. XRD study. The crystal structures of the combustion products were investigated using the X-ray diffraction technique. Fig. 1 through 3 present the XRD patterns of the combustion and calcined products using L-alanine, glycine, and urea fuels at different pH values (3, 6, and 10) at Φc = 1. Inspection of the figures revealed that the fuel type and pH of the combustion media have a significant impact on the phase composition and crystallite size of the products. It is reported that pH of the starting solutions of the combustion media can prevent selective precipitation and/or phase segregation during the combustion process which may cause phase inhomogeneity of the reaction blend.38 In the current investigation, we used different fuels such as urea, L-alanine, and glycine, which might act simultaneously as chelating agents as well. The formation of metal coordination or metal chelation complexes is a pH dependent. And the produced soluble chelation complexes are an essential point in preventing the selective precipitation of the cations of interest and this can support the homogeneity of the reaction media during solvents evaporation throughout the combustion process.38,39 Moreover, hydrolysis of the cation compounds and their complexes can be controlled by the pH value of the reaction media.40 Therefore, during the combustion process, there will be a competition between two main reactions: (1) formation of metal chelations and (2) hydrolysis of the formed metal chelations and other metal compounds. And these two main mechanisms can tune the phase, morphology, and/or crystallite size of the products of the combustion process. Based on this discussion, we can explain our following results. Fig. 1 revealed that L-alanine fuel resulted in a mixture (A6) of ZnMnO3, ZnO, and Mn3O4 through the combustion process at pH 6. Calcination of this mixture at 500 °C for 2 h produced pure product (A6_500), corresponding to tetragonal ZnMn2O4 (JCPDS card no. 24-1133, space group: I41/amd). The diffraction peaks of the ZnMn2O4 product appeared at 2θ values of ca. 29.32°, 31.23°, 33.00°, 36.41°, 44.63°, 58.92°, 60.82° and 64.82° corresponding to the reflection planes values of (112), (200), (103), (211), (220), (321), (224), and (400), respectively. Fig. 2 and 3 revealed that combustion at pH value of 3 and 10 brought about a mixture of ZnMn2O4, ZnO, and MnO (A3), and a mixture of Zn(OH)(NO3)(H2O) and (Mn2Zn)7(OH)10(CO3)2Zn5(OH)8 (A10), respectively. And calcination of the combustion products did not give a pure ZnMn2O4 product, but it resulted in different mixed oxides (A3_500 and A10_500), as shown in Fig. 2 and 3. L-Alanine is an amino acid which can exist in three forms: +NH3CH(CH3)COOH, +NH3CH(CH3)COO− (zwitterionic form), and NH2CH(CH3)COO−, as dominant species. This will depend on the pH values of the media: 3, 6, and 10, since the isoelectric point value of this amino acid is 6. The rate of formation of L-alanine–metal complexes is low at acidic pH values, and it enhances with increasing the pH value of the media. Besides, in alkaline media the formed chelate complexes may undergo hydrolysis forming hydroxide species, and this may result in formation of a mixture of different metal oxide species on combustion, as shown in Fig. 3.
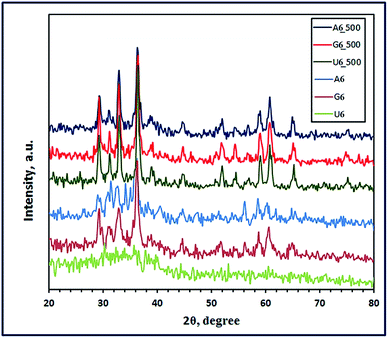 |
| Fig. 1 XRD patterns of the as-fabricated ZnMn2O4 products after combustion (A6, G6, and U6) and calcination (A6_500, G6_500, and U6_500) using L-alanine, glycine and urea fuels, respectively. | |
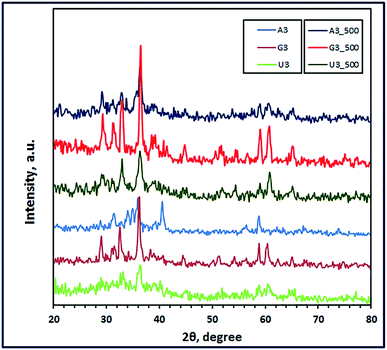 |
| Fig. 2 XRD patterns of the as-fabricated ZnMn2O4 products (at pH 3) after combustion (A3, G3, and U3) and calcination (A3_500, G3_500, and U3_500) using L-alanine, glycine and urea fuels, respectively. | |
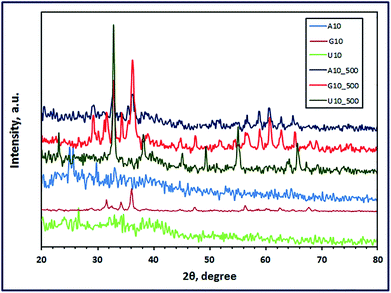 |
| Fig. 3 XRD patterns of the as-fabricated ZnMn2O4 products (at pH 10) after combustion (A10, G10, and U10) and calcination (A10_500, G10_500, and U10_500) using L-alanine, glycine and urea fuels, respectively. | |
Interestingly, the combustion reaction using glycine fuel at pH 6 and 3 gave pure tetragonal ZnMn2O4 (JCPDS card no. 24-1133, space group: I41/amd) with crystallite size values of 11 (G6) and 27 nm (G3), respectively, as shown in Fig. 1 and 2. No other peaks were observed for other crystalline products in the XRD patterns. In addition, calcination of these combustion products gave ZnMn2O4 (G6_500) with crystallite size of 19 nm, and a mixture of ZnMn2O4, Mn3O4, and Mn5O8 (G3_500), as depicted in Fig. 1 and 2, respectively. Glycine is also an amino acid prevailing specific glycine species, based on pH values of the starting solutions of the combustion media. At pH 3, glycine may exist mainly as +NH3CH2COOH and the coordination ability of this species with zinc and manganese cations is weak; moreover, glycine may exist mainly as +NH3CH2COO− (zwitterion, isoelectric point is ∼6) at pH 6 and the complexing ability of this ion is higher than the previous one.41 However, both pH values resulted in pure ZnMn2O4 nanostructures on combustion. The crystallite size of the burnt nanoparticles at pH 6 was smaller than that at pH 3. This may be attributed to that zwitterionic glycine–metal complexes are less soluble at pH 6. And the formed nucleates may prefer to coagulate forming gel quickly during the evaporation of the solvent in the combustion process. Therefore, the primary small particles (crystallites) do not have much time in the combustion process to grow into larger crystallites producing smaller crystallites.40 Increasing the pH of the combustion to 10 enhanced the chelation ability of the glycine species at that pH (NH2CH2COO−); however, the rate of hydrolysis also increased forming different metal hydroxide species as precipitate along with various glycine–metal chelates. And this might explain producing of a mixture of different manganese–zinc oxides on combustion at pH 10.
On the other hand, using urea as a fuel produced a mixture of ZnMnO3 and Mn3O4, or a mixture of ZnMn2O4 and Mn3O4 at pH 6 (U6) or at pH 3 (U3), respectively. Calcination of these combustion products at 500 °C for 2 h produced pure tetragonal ZnMn2O4 (U6_500 and U3_500) (JCPDS card no. 24-1133, space group: I41/amd) with a crystallite size of 25 and 20 nm, respectively, as shown in Fig. 1 and 2. We have not observed any other peaks for other crystalline products in the XRD patterns. Moreover, adjusting the pH of the combustion media to 10 gave (Mn2Zn)7(OH)10(CO3)2 precursor (U10) on combustion, as shown in Fig. 3. However, calcination of that precursor produced a mixture of ZnMn2O4 and Mn2O3 (U10_500). The obtained results exhibited that these pH values do not have a remarkable influence on the combustion products. This may be attributed to that during heating the solutions prior to the combustion process, urea decomposes into ammonia and CO2 which can increase the alkalinity of the media enhancing hydrolysis of the cations into different hydroxides.8 These metal hydroxides might be converted into various metal oxides on combustion. However, on sintering the burnt oxides, produced at pH 3 and 6, may react in the solid state forming pure ZnMn2O4 nanostructures.
Based on the obtained results, we have arrived at a conclusion that pure ZnMn2O4 nanoparticles can be synthesized using L-alanine and urea fuels at pH 6 on combustion followed by calcination at 500 °C for 2 h. However, the combustion process resulted in directly pure ZnMn2O4 nanoparticles when glycine fuel was used and the combustion media pH was 6. And calcination of the combustion product in this case did not influence the product composition but it increased the crystallite size only. On the other side, adjusting pH to 3 produced pure ZnMn2O4 product only after calcination of the combustion products at 500 °C for 2 h when glycine and urea were used as fuels. Consequently, the present combustion method, in which Φc = 1, gave pure spinel ZnMn2O4 nanoparticles with much smaller crystallite size compared to the reported one.22 In that reported method, the applied Φc was not equal to 1; therefore, the authors used higher calcination temperature, 600 °C, for a longer time (6 h) so that the produced crystallite size was ca. 50 nm.22 Notably, the average crystallite sizes of the combustion and calcined pure products were estimated employing the Debye–Scherrer equation (eqn (4)) and listed in Table 1.42
|
D = 0.9λ/β cosθB
| (4) |
where,
β,
λ, and
θB are the full width of the diffraction peak at half maximum (FWHM), X-ray radiation wavelength (nm), and Bragg diffraction angle, respectively.
Table 1 Influence of fuel, pH and calcination temperature on the produced phases of the combustion processes
Fuel |
Equivalence ratio, Φc |
pH |
Product phases after combustion |
Product phases after calcination at 500 °C for 1 h |
Crystallite size (after combustion, after calcination) (nm) |
Product label (after combustion, calcination) |
L-Alanine |
1 |
3 |
ZnMn2O4, ZnO, MnO |
ZnMn2O4, Mn3O4 |
—, — |
A3, A3_500 |
1 |
6 |
ZnMnO3, ZnO, Mn3O4 |
ZnMn2O4 |
—, 28 |
A6, A6_500 |
1 |
10 |
Zn(OH)(NO3)(H2O), (Mn2Zn)7(OH)10(CO3)2Zn5(OH)8 |
ZnMn2O4, Mn3O4, Mn2O3 |
—, — |
A10, A10_500 |
Glycine |
1 |
3 |
ZnMn2O4 |
ZnMn2O4, Mn3O4, Mn5O8 |
27, — |
G3, G3_500 |
1 |
6 |
ZnMn2O4 |
ZnMn2O4 |
11, 19 |
G6, G6_500 |
1 |
10 |
ZnMn2O4, ZnO, Mn3O4, Mn5O8 |
ZnMn2O4, ZnO |
— |
G10, G10_500 |
Urea |
1 |
3 |
Mn3O4, ZnMn2O4 |
ZnMn2O4 |
—, 20 |
U3, U3_500 |
1 |
6 |
Mn3O4, ZnMnO3 |
ZnMn2O4 |
—, 25 |
U6, U6_500 |
1 |
10 |
(Mn2Zn)7(OH)10(CO3)2 |
ZnMn2O4, Mn2O3 |
—, — |
U10, U10_500 |
3.1.2. FT-IR study. The chemical compositions of the combustion and calcination products were elucidated by using the FT-IR spectra. In this light, Fig. 4 revealed the FT-IR spectra of the combustion products generated using L-alanine, glycine, and urea fuels at fuel-to-oxidant equivalence ratio (Φc) of 1 and at different pH values of the combustion media. The FT-IR results exhibited that glycine fuel was the optimum fuel for the current combustion procedure because this fuel generated ZnMn2O4 phase on combustion, at pH 3 (G3) and pH 6 (G6) of the combustion media (Fig. 4). This conclusion is based on the two characteristic vibrational frequencies appeared at ca. 496 and 615 cm−1 which can be attributed to ZnMn2O4.43 However, this fuel at the aforementioned pH conditions produced some organic residue, and this was attributed to appearance of some vibrational frequencies at ca. 1050 (C–O stretching) and 1426 cm−1 (CH2 stretching). This is consistent with the published results.43,44 Notably, increasing the pH of the combustion media to pH 10, resulted in different phases (G10) on combustion as shown in Table 2. Nevertheless, the other used fuels (urea and alanine) did not gave pure ZnMn2O4 phase on combustion, as shown in Fig. 4 and Table 2. However, alanine fuel produced phases (A3): ZnMn2O4, ZnO, and MnO (at pH 3, A3), phases (A6): ZnMnO3, ZnO, and Mn3O4 (at pH 6), and phases (A10): Zn(OH)(NO3)(H2O) and (Mn2Zn)7(OH)10(CO3)2Zn5(OH)8 (at pH 10), along with some organic residues,2,43–49 as shown in Fig. 4 and Table 2. Urea fuel gave also different phases (U3, U6, U10) on combustion at different pH values. Based on glycine fuel results, we focused on the combustion products (A6, G6, U6) generated using the three fuels at pH 6, for comparison purpose. These combustion products were calcinated at 500 °C for 2 h to eliminate the organic residues and converting some multi-phases products into single-phase products. The FT-IR spectra of the calcined products (A6_500, G6_500, U6_500) are depicted in Fig. 4. Interestingly, the calcined products; G6_500 and U6_500, of the combustion samples generated from glycine and urea fuels, respectively, are composed of pure ZnMn2O4 phase. This was clear by the presence of only the characteristic vibrational frequencies of ZnMn2O4 product (ca. 508 and 620 cm−1). However, calcination of the combustion products generated using alanine fuel did not produce pure product, and it generated organic residue along with ZnMn2O4 product (A6_500), as listed in Table 2. Fig. 4 also shows two characteristic vibrational absorptions corresponding to bending and stretching vibrations of OH groups of adsorbed water on all products at ca. 1600 and 3430 cm−1, respectively. These results are consistent with the reported data.6,20,50–54 The vibrational frequencies of the produced products are presented in Table 2.
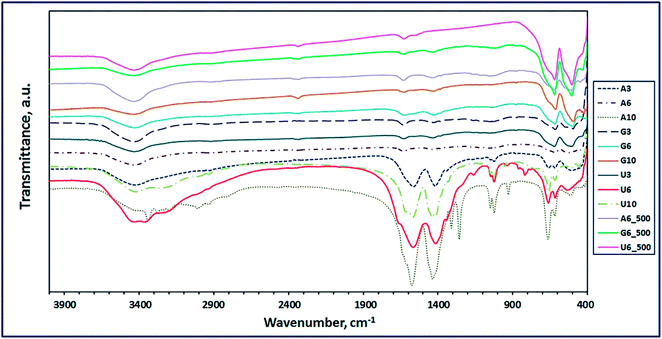 |
| Fig. 4 FT-IR spectra of the as-fabricated ZnMn2O4 products after combustion and calcination (A6_500, G6_500, and U6_500) using L-alanine, glycine and urea fuels, respectively. | |
Table 2 FT-IR vibrational frequencies of the as-synthesized products
Product |
Label |
νMn–O, cm−1 |
νZn–O, cm−1 |
νMnCO3, cm−1 |
νZnCO3, cm−1 |
νC–O, cm−1 |
νCH2, cm−1 |
νOH, cm−1 |
δOH, cm−1 |
sh = shoulder. |
ZnMn2O4 |
A3 |
600, 617 |
510 |
— |
— |
1024 |
1419 |
3430 |
1563 |
ZnO |
— |
510 |
— |
— |
MnO |
600 |
— |
— |
— |
ZnMnO3 |
A6 |
617 |
500sha |
— |
— |
1054 |
1432 |
3433 |
1613 |
ZnO |
— |
500sha |
— |
— |
Mn3O4 |
520, 617 |
— |
— |
— |
Zn(OH)(NO3)(H2O) |
A10 |
— |
463 |
— |
— |
1024 |
1436 |
3339 |
1575 |
(Mn2Zn)7(OH)10(CO3)2Zn5(OH)8 |
522, 665 |
463 |
1253, 105, 930, 665, 463 |
1253, 1050, 930, 665, 463 |
ZnMn2O4 |
G3 |
615 |
496 |
— |
— |
1052 |
1431 |
3432 |
1631 |
ZnMn2O4 |
G6 |
618 |
501 |
— |
— |
1050sha |
1426 |
3432 |
1623 |
ZnMn2O4 |
G10 |
613 |
494 |
— |
— |
— |
1436 |
3434 |
1626 |
ZnO |
— |
458 |
— |
— |
Mn3O4 |
613, 494 |
— |
— |
— |
Mn5O8 |
877, 613 |
— |
— |
— |
Mn3O4 |
U3 |
508, 621 |
508 |
— |
— |
1053 |
1433 |
3433 |
1626 |
ZnMn2O4 |
508, 621 |
— |
— |
Mn3O4 |
U6 |
534, 616 |
534 |
— |
— |
1024 |
1417 |
3432 |
1566 |
ZnMnO3 |
534, 616 |
— |
— |
(Mn2Zn)7(OH)10(CO3)2 |
U10 |
658 |
460 |
1416, 952, 658 |
1416, 952, 460 |
1049 |
1440 |
3427 |
1567 |
ZnMn2O4 |
A6_500 |
620 |
508 |
— |
— |
1052 |
1431 |
3434 |
1631 |
ZnMn2O4 |
G6_500 |
621 |
505 |
— |
— |
— |
— |
3434 |
1632 |
ZnMn2O4 |
U6_500 |
620 |
504 |
— |
— |
— |
— |
3434 |
1626 |
3.1.3. Morphology investigation. The morphology of the as-fabricated ZnMn2O4 products: A6_500, G6_500, and U6_500, calcined at 500 °C was investigated by using a field-emission scanning electron microscope (FE-SEM). As shown in Fig. 5(ai), L-alanine fuel generated ZnMn2O4 nanostructure (A6_500) with aggregates in the form of irregular and curled layers. However, a high-magnification FE-SEM image (Fig. 5(aii)) exhibits that those layers are composed of sphere-like particles with an average diameter of ca. 80 nm. Fig. 5(aii) also shows the existence of in-plane pores within the ZnMn2O4 layers. However, there are some dark spots appeared in those FE-SEM images (Fig. 5(ai and ii)) indicating the presence of some carbon residues along with the ZnMn2O4 product. Fig. 5(bi) revealed that glycine fuel produced ZnMn2O4 product (G6_500) with porous irregular agglomerates composed of porous peanut-like particles with an average size of ca. 300 nm, as shown in a high-magnification FE-SEM image (Fig. 5(bii)). Whereas, the calcined ZnMn2O4 product particles (U6_500), produced from combustion with urea fuel, were aggregated in thick curled layers and these layers were agglomerated in flower-like structures as shown in a low-magnification FE-SEM image (Fig. 5(ci)). Close inspection of the high-magnification FE-SEM image (Fig. 5(cii)) of these layers, revealed that the layers consisted of uniform sphere-like particles with an average diameter of ca. 100 nm. Notably, the porous structures of the ZnMn2O4 products are produced during the combustion process by action of the released gases (e.g. CO2 and N2) at elevated temperatures.20,21,53 The detailed structures of the ZnMn2O4 products calcined at 500 °C are displayed in TEM images (Fig. 6). Fig. 6(a–c) showed that the products generated by the three different fuels have similar morphologies. And the produced ZnMn2O4 particles have a hexagon, sphere, and cube-like structures with an average particle size of ca. 30, 20, 25, for A6_500, G6_500, and U6_500 ZnMn2O4 products, respectively. The obtained results are compatible with the XRD ones. Eventually, the EDS analysis results (not shown here) support that the as-synthesized spinel ZnMn2O4 is composed solely of zinc, manganese, and oxygen elements.
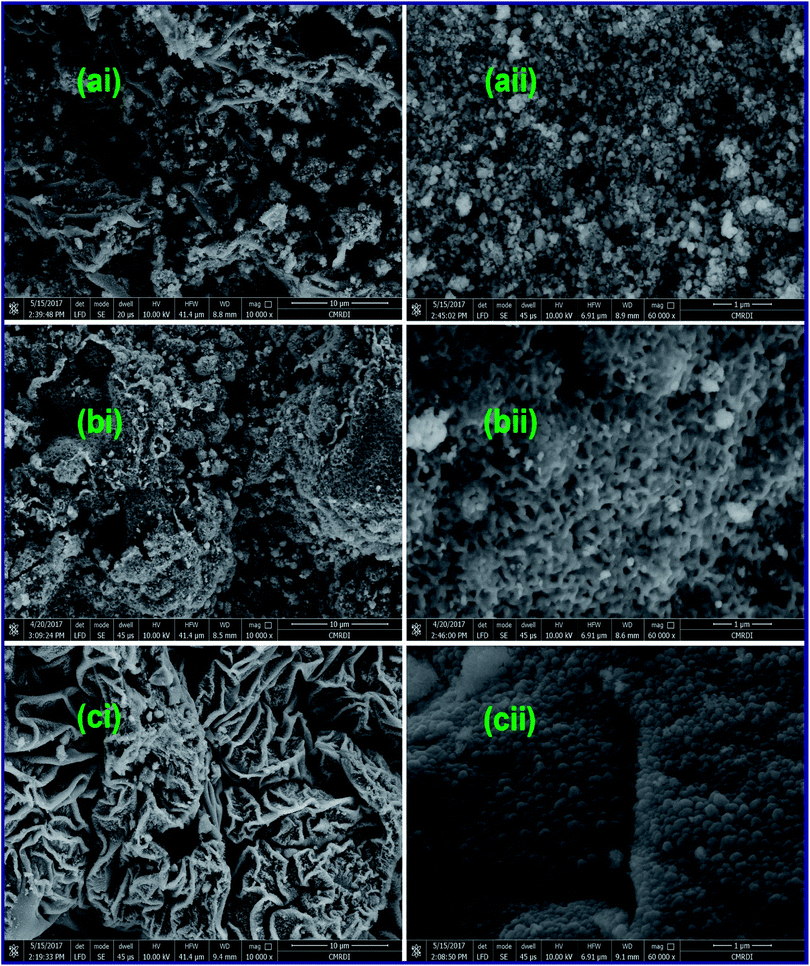 |
| Fig. 5 FE-SEM images of the as-fabricated ZnMn2O4 products after calcination (A6_500 (a), G6_500 (b), and U6_500 (c)) by L-alanine, glycine and urea fuels, respectively. | |
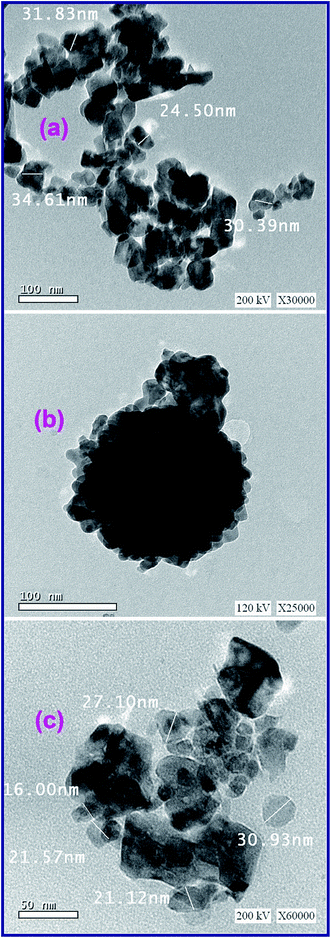 |
| Fig. 6 TEM images of the as-fabricated ZnMn2O4 products after calcination (A6_500 (a), G6_500 (b), and U6_500 (c)) generated by L-alanine, glycine and urea fuels, respectively. | |
3.1.4. Optical properties investigation. We focused in our subsequent investigation on the spinel ZnMn2O4 product (G6_500) fabricated using glycine fuel owing to its small crystallite size and high purity compared to the other products (A6_500 and U6_500). The optical band gap energy (Eg) of the spinel ZnMn2O4 product (G6_500) can be determined employing its UV-Vis diffuse reflectance spectrum (Fig. 7(a)) by utilizing the Kubelka–Munk function, F(R), (eqn (5)).55,56 |
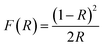 | (5) |
where, R corresponds to the percentage reflectance. Fig. 7(a) reveals an absorption band in the visible region at ca. 656 nm which is consistent with the published results.10 The spinel ZnMn2O4 product (G6_500) direct band gap energy was calculated by plotting [F(R)xhν]2 versus hν (Fig. 7(b)) where hν is the incident photon energy (eV). Then, the band gap energy value (Eg) of the as-fabricated spinel ZnMn2O4 product (G6_500) was determined to be 1.51 eV, utilizing the extrapolation of the linear section of the plot at [F(R)xhν]2 = 0. And the calculated band gap energy value is compatible with the reported ones.10,14 The estimated band gap energy value indicates the semiconducting properties of the as-fabricated spinel ZnMn2O4 product (G6_500).2,8
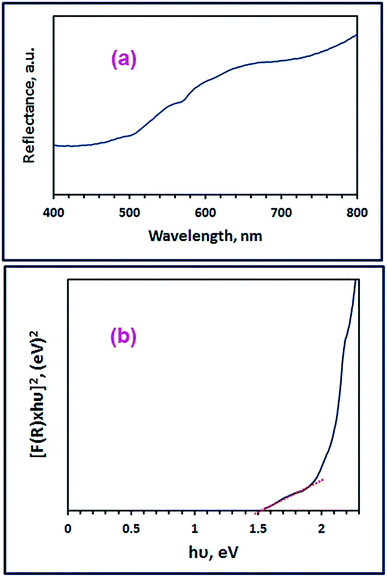 |
| Fig. 7 Diffuse reflectance spectrum (a), and plot [F(R)xhν]2 against hν (b) of the as-fabricated ZnMn2O4 product (G6_500). | |
3.2. Colorimetric determination of omeprazole and lansoprazole drugs
It was reported that organic sulfide compounds can be oxidized to sulfoxide (R2SO) and/or sulfone compounds (R2SO2), or it can even be brominated utilizing N-bromosuccinimide.36,57,58 The oxidation of drugs containing sulfide groups can be also performed using iodate in an acidic medium and by following the liberated iodine spectrophotometrically, the concentration of the drugs can be determined.59,60 However, these methods are multistep and tedious procedures. Consequently, we have developed a simple and accurate method for determination of such type of drugs based on their oxidation using semiconducting nanomaterials. This determination method was based on that when the drug containing sulfide functional group was stirred with the as-prepared ZnMn2O4 nanoparticles, the color of the solution was turned into yellow. In order to be able to interpret this result, we repeated these experiments in the presence of an oxidizing agent such as H2O2 and we did not add any amount of ZnMn2O4 to the reaction. The same yellow color with similar absorbance was obtained. Based on this, we concluded that the produced yellow color upon addition of ZnMn2O4 to the drugs of interest might be owing to the oxidation of the drugs with that semiconducting material (ZnMn2O4). Moreover, this oxidation may be due to one or two of the following reasons: the first reason is: under visible light, the electrons absorb light and transfer through excitation from valence band to the conduction band of ZnMn2O4 leaving holes in the valence band. These holes of the valence band may abstract electrons from the sulfide groups of the drugs leading to their oxidation. The second reason is: Mn3+ of the spinel product (ZnMn2O4) may oxidize the drugs, in the presence of H2O molecules, from R2SO forms to sulfone derivatives (R2SO2), leaving Mn2+. Hence, during the oxidation process of the drugs, some of the spinel ZnMn2O4 product may be converted into ZnMn2O3 due to its own reduction, as presented in Scheme 3. Hence, we have investigated various factors influencing the determination procedure such as selecting absorption wavelength of the oxidized drugs, reaction time, reaction temperature, pH of the media, volume of the drug solution, concentration of the drugs, and dose of the nanomaterial.
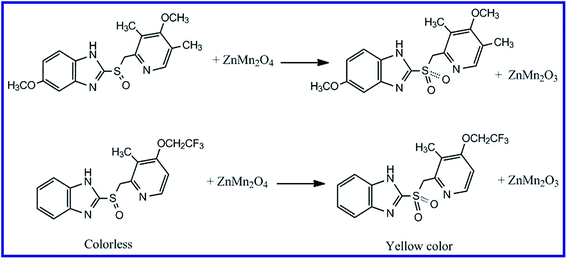 |
| Scheme 3 Proposed mechanism for the oxidation of omeprazole and lansoprazole drugs with ZnMn2O4 nanoparticles. | |
3.2.1. Selection of the appropriate absorption wavelength. A solution of the drug of interest (omeprazole or lansoprazole) was stirred with 50 mg of ZnMn2O4 nanoparticles, as explained in the Experimental section, for 1 h. The produced yellow color was scanned using an UV-Vis spectrophotometer in the wavelength range of 200–800 nm to detect the λmax of the oxidized drugs. Fig. 8 and 9 exhibited spectra of the omeprazole and lansoprazole drugs, respectively, before and after oxidation with ZnMn2O4 nanomaterial. The images of the drug solutions before and after adding ZnMn2O4 nanoparticles are presented in the inset of the corresponding figures (Fig. 8 and 9). The images clearly show turning the colorless color of the drug solutions into yellow color upon addition of the ZnMn2O4 nanoparticles. The spectra of the oxidized drugs revealed an absorption maximum at ca. 454 nm.
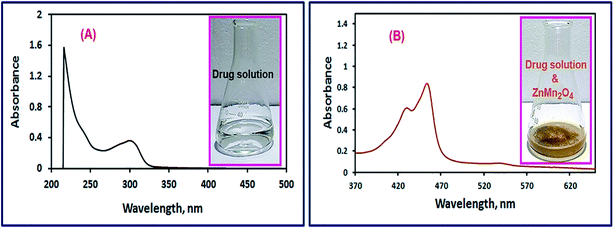 |
| Fig. 8 UV-Vis spectra of omeprazole drug solutions in the absence (A), and presence of ZnMn2O4 nanoparticles (B). The images of the drug solutions before and after adding ZnMn2O4 nanoparticles are presented in the inset of the corresponding figures. | |
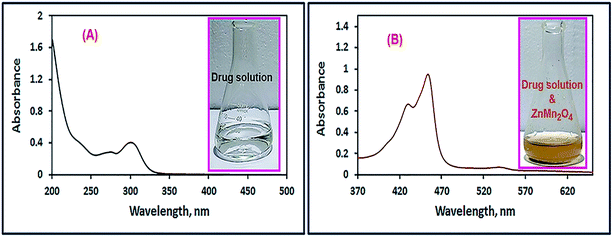 |
| Fig. 9 UV-Vis spectra of lansoprazole drug solutions in the absence (A), and presence of ZnMn2O4 nanoparticles (B). The images of the drug solutions before and after adding ZnMn2O4 nanoparticles are presented in the inset of the corresponding figures. | |
3.2.2. Effect of pH. The influence of reaction media pH on the color development was examined in the pH range of 2–9 at ambient temperature (25 ± 1 °C). The results exhibited that the intensity of the yellow enhanced gradually with increasing the pH value until it reached its maximum at pH 7 and it remained constant as displayed in Fig. 10. Hence, the optimum pH value for this color development was opted to be 7 in the subsequent study.
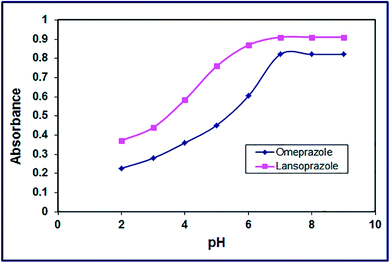 |
| Fig. 10 Effect of pH of the drug solution on color development of the products. | |
3.2.3. Effect of time. Solutions of the drugs (omeprazole or lansoprazole) were blended with 50 mg of ZnMn2O4 nanoparticles, as explained in the Experimental section. The optimum reaction time was estimated by following the color development with time elapsed at pH 7 and ambient temperature (25 ± 1 °C). It is clear from Fig. 11 that complete color intensity of the oxidized drugs was obtained after 30 and 40 min for omeprazole and lansoprazole drugs, respectively, and the color remained stable for 24 h.
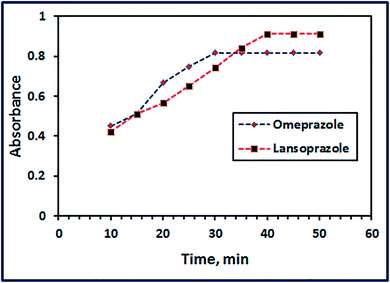 |
| Fig. 11 Effect of reaction time on color development of the products. | |
3.2.4. Effect of temperature. Studying the effect of temperature (in the range of 25–55 °C) on the color intensity of the oxidized drugs revealed that temperature has not a remarkable effect, as shown in Fig. 12.
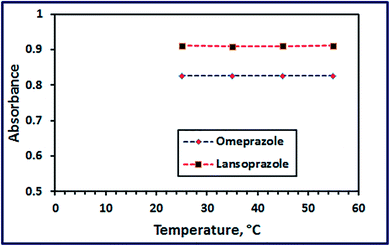 |
| Fig. 12 Effect of reaction temperature on color development of the products. | |
3.2.5. Effect of ZnMn2O4 dose. Effect of ZnMn2O4 nanostructure dose on the color intensity of the oxidized drugs (at λmax 454 nm) has been examined at constant drug concentration and at pH 7. The results depicted in Fig. 13 exhibited that the color intensity of the reaction mixture enhanced with increasing the nano-material quantity until it reached maximum at 50 mg. Afterward, increasing the ZnMn2O4 nanosensor dose did not have any significant influence on the color intensity.
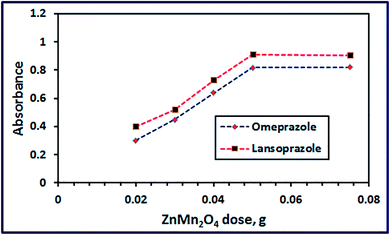 |
| Fig. 13 Effect of ZnMn2O4 dose on color development of the products. | |
3.2.6. Calibration and precision of the ZnMn2O4 chemical nanosensor. Typical calibration curves for omeprazole and lansoprazole with the as-prepared ZnMn2O4 nanosensor obtained from linear regression analysis of absorbance against concentration of the drugs (μg mL−1) were made. Plotting the concentration of each drug versus its absorbance revealed that Beer's law limits were 0.80–8.0 and 0.80–8.8 μg mL−1 at λmax 454 nm for omeprazole and lansoprazole, respectively, as shown in Fig. 14. Moreover, the corresponding correlation coefficients are 0.999 and 0.998 for omeprazole and lansoprazole drugs, respectively. The Sandell sensitivity was estimated to be 0.012 and 0.0125 μg cm−2, for omeprazole and lansoprazole drugs, respectively. Moreover, the limit of detection was found to be 0.264 and 0.093 μg mL−1 for omeprazole and lansoprazole, respectively. And the limit of quantification was determined to be 0.88 and 0.98 μg mL−1 for omeprazole and lansoprazole, respectively. Therefore, it can be concluded from the above discussion that ZnMn2O4 nanostructure can be applied as a chemical nanosensor to detect omeprazole and lansoprazole drugs precisely in ethanolic solutions. On the other hand, some of the published spectrophotometric methods have one or more disadvantages, such as using extraction or heating during determination, low sensitivity, or determination narrow range, as tabulated in Table 3.
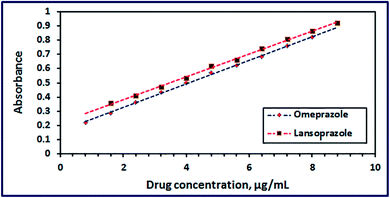 |
| Fig. 14 Calibration plots of omeprazole and lansoprazole drugs using ZnMn2O4 nanoparticles. | |
Table 3 Comparison of the performance of the proposed method with those of the published methods for the determination of lansoprazole and omeprazole drugsa
Item no. |
Used reagents |
λmax (nm) |
Linear range (μg mL−1) |
Main remarks |
Ref. |
Where, L = lansoprazole, Om = omeprazole, MBTH = 3-methyl-2-benzothiazolinone hydrazine, and NBS = N-bromosuccinimide. |
1L |
Bromate–bromide mixture and iron(II) with thiocyanate |
470 |
0.5–4 |
It includes multistep procedure, many reagents, and narrower linear range |
61 |
2L |
NBS–chloranilic acid utilizing FIA system |
530 |
5–150 |
It is less sensitive and it includes many reagents |
62 |
3L |
Ceric ammonium sulphate with indigo carmine dye |
610 |
0.25–3 |
Sensitive but it includes multistep procedure, many reagents, and narrower linear range |
63 |
4L−Om |
Fe(III) with ferricyanide |
730L, 720Om |
0.2–3.6, 0.08–2.8 |
Sensitive but it includes many used reagents and slow reactions |
64 |
5Om |
Celestine blue dye and NBS in presence of HCl solution |
540 |
0.4–2.4 |
Sensitive but it includes many used reagents, multistep reactions, and narrower linear range |
65 |
6Om |
MBTH reagent and Fe(III) |
660 |
0.1–10 |
Less sensitive and it includes multistep reactions |
65 |
7L−Om |
ZnMn2O4 |
454, 454 |
0.8–8.8L, 0.8–8Om |
It is a single step reaction, and sensitive. It does not require heating, extraction, or pH adjustment |
Present procedure |
4. Conclusions
In this investigation, a novel approach has been adopted to use ZnMn2O4 nanostructure as a chemical nanosensor. Different ZnMn2O4 nanostructures have been synthesized using different fuels such as L-alanine, glycine, and urea via an auto-combustion process. Various factors influencing the combustion process have been explored. Glycine fuel produced the purest spinel ZnMn2O4 product on combustion while the other fuels generated multi-phases. The as-prepared spinel ZnMn2O4 nanostructure proved its efficiency as a chemical nanosensor for the sensitive determination of omeprazole and lansoprazole drugs. The developed chemical nanosensor showed high sensitivity, low detection limit, and a relatively wide linear range (0.80–8.0 and 0.80–8.8 μg mL−1 at λmax 454 nm for omeprazole and lansoprazole, respectively). This study may open a new approach for developing new chemical sensors for colorimetric determination of various drugs.
Conflicts of interest
There are no conflicts to declare.
References
- M. Y. Nassar and M. Khatab, Cobalt ferrite nanoparticles via a template-free hydrothermal route as an efficient nano-adsorbent for potential textile dye removal, RSC Adv., 2016, 6, 79688–79705 RSC.
- M. Y. Nassar and S. Abdallah, Facile controllable hydrothermal route for a porous CoMn2O4 nanostructure: synthesis, characterization, and textile dye removal from aqueous media, RSC Adv., 2016, 6, 84050–84067 RSC.
- N. Guo, X. Q. Wei, X. L. Deng and X. J. Xu, Synthesis and property of spinel porous ZnMn2O4 microspheres, Appl. Surf. Sci., 2015, 356, 1127–1134 CrossRef CAS.
- W. Dang, F. Wang, Y. Ding, C. Feng and Z. Guo, Synthesis and electrochemical properties of ZnMn2O4 microspheres for lithium-ion battery application, J. Alloys Compd., 2017, 690, 72–79 CrossRef CAS.
- M. Y. Nassar, T. Y. Mohamed and I. S. Ahmed, One-pot solvothermal synthesis of novel cobalt salicylaldimine–urea complexes: a new approach to Co3O4 nanoparticles, J. Mol. Struct., 2013, 1050, 81–87 CrossRef CAS.
- M. Y. Nassar, Size-controlled synthesis of CoCO3 and Co3O4 nanoparticles by free-surfactant hydrothermal method, Mater. Lett., 2013, 94, 112–115 CrossRef CAS.
- M. Y. Nassar and I. S. Ahmed, Template-free hydrothermal derived cobalt oxide nanopowders: synthesis, characterization, and removal of organic dyes, Mater. Res. Bull., 2012, 47, 2638–2645 CrossRef CAS.
- M. Y. Nassar and I. S. Ahmed, Hydrothermal synthesis of cobalt carbonates using different counter ions: an efficient precursor to nano-sized cobalt oxide (Co3O4), Polyhedron, 2011, 30, 2431–2437 CrossRef CAS.
- J. Yuan, C. Chen, Y. Hao, X. Zhang, R. Agrawal, W. Zhao, C. Wang, H. Yu, X. Zhu, Y. Yu, Z. Xiong and Y. Xie, Fabrication of three-dimensional porous ZnMn2O4 thin films on Ni foams through electrostatic spray deposition for high-performance lithium-ion battery anodes, J. Alloys Compd., 2017, 696, 1174–1179 CrossRef CAS.
- R. Gherbi, Y. Bessekhouad and M. Trari, Structure, optical and transport properties of Mg-doped ZnMn2O4, J. Alloys Compd., 2016, 655, 188–197 CrossRef CAS.
- G. Fierro, M. Lo Jacono, M. Inversi, R. Dragone and G. Ferraris, Preparation, characterization and catalytic activity of Co–Zn-based manganites obtained from carbonate precursors, Appl. Catal., B, 2001, 30, 173–185 CrossRef CAS.
- L. Zhao, X. Li and J. Zhao, Fabrication, characterization and photocatalytic activity of cubic-like ZnMn2O4, Appl. Surf. Sci., 2013, 268, 274–277 CrossRef CAS.
- C. Chanel, S. Fritsch, C. Drouet, A. Rousset, M. L. Martínez Sarrión, L. Mestres and M. Morales, Synthesis, thermogravimetric and high temperature X-ray diffraction analyses of zinc-substituted nickel manganites, Mater. Res. Bull., 2000, 35, 431–439 CrossRef CAS.
- R. Gherbi, Y. Bessekhouad and M. Trari, Optical and transport properties of Sn-doped ZnMn2O4 prepared by sol–gel method, J. Phys. Chem. Solids, 2016, 89, 69–77 CrossRef CAS.
- K. Chhor, J. F. Bocquet, C. Pommier and B. Chardon, Heat capacity and thermodynamic behaviour of Mn3O4 and ZnMn2O4 at low temperatures, J. Chem. Thermodyn., 1986, 18, 89–99 CrossRef CAS.
- K. J. Kim and P. Young Ran, Sol–gel growth and structural and optical investigation of manganese-oxide thin films: structural transformation by Zn doping, J. Cryst. Growth, 2004, 270, 162–167 CrossRef CAS.
- X.-D. Zhang, Z.-S. Wu, J. Zang, D. Li and Z.-D. Zhang, Hydrothermal synthesis and characterization of nanocrystalline Zn–Mn spinel, J. Phys. Chem. Solids, 2007, 68, 1583–1590 CrossRef CAS.
- S. Guillemet-Fritsch, C. Chanel, J. Sarrias, S. Bayonne, A. Rousset, X. Alcobe and M. L. Martinez Sarriòn, Structure, thermal stability and electrical properties of zinc manganites, Solid State Ionics, 2000, 128, 233–242 CrossRef CAS.
- M. Y. Nassar, T. Y. Mohamed, I. S. Ahmed and I. Samir, MgO nanostructure via a sol-gel combustion synthesis method using different fuels: an efficient nano-adsorbent for the removal of some anionic textile dyes, J. Mol. Liq., 2017, 225, 730–740 CrossRef CAS.
- M. Y. Nassar, E. I. Ali and E. S. Zakaria, Tunable auto-combustion preparation of TiO2 nanostructures as efficient adsorbents for the removal of an anionic textile dye, RSC Adv., 2017, 7, 8034–8050 RSC.
- M. Y. Nassar, I. S. Ahmed and I. Samir, A novel synthetic route for magnesium aluminate (MgAl2O4) nanoparticles using sol–gel auto combustion method and their photocatalytic properties, Spectrochim. Acta, Part A, 2014, 131, 329–334 CrossRef CAS PubMed.
- A. Sahoo and Y. Sharma, Synthesis and characterization of nanostructured ternary zinc manganese oxide as novel supercapacitor material, Mater. Chem. Phys., 2015, 149–150, 721–727 CrossRef CAS.
- T. Zhang, Y. Gao, H. Yue, H. Qiu, Z. Guo, Y. Wei, C. Wang, G. Chen and D. Zhang, Convenient and high-yielding strategy for preparing nano-ZnMn2O4 as anode material in lithium-ion batteries, Electrochim. Acta, 2016, 198, 84–90 CrossRef CAS.
- N. El-Enany, F. Belal and M. Rizk, The alternating current polarographic behavior and determination of lansoprazole and omeprazole in dosage forms and biological fluids, J. Biochem. Biophys. Methods, 2008, 70, 889–896 CrossRef CAS PubMed.
- A. De Milito, R. Canese, M. L. Marino, M. Borghi, M. Iero, A. Villa, G. Venturi, F. Lozupone, E. Iessi, M. Logozzi, P. D. Mina, M. Santinami, M. Rodolfo, F. Podo, L. Rivoltini and S. Fais, pH-dependent antitumor activity of proton pump inhibitors against human melanoma is mediated by inhibition of tumor acidity, Int. J. Cancer, 2010, 127, 207–219 CrossRef CAS PubMed.
- F. C. Souza, T. M. Baptista, E. B. Marques, R. B. M. Barros and C. B. V. Scaramello, Omeprazole does not modulate pharmacokinetic of digoxin in patients with heart failure, Int. J. Cardiol., 2015, 179, 343–344 CrossRef PubMed.
- M. Gabello, M. C. Valenzano, M. Barr, P. Zurbach and J. M. Mullin, Omeprazole Induces Gastric Permeability to Digoxin, Dig. Dis. Sci., 2010, 55, 1255–1263 CrossRef CAS PubMed.
- M. L. C. Montanari, Q. B. Cass, A. Leitão, A. D. Andricopulo and C. A. Montanari, The role of molecular interaction fields on enantioselective and nonselective separation of chiral sulfoxides, J. Chromatogr. A, 2006, 1121, 64–75 CrossRef CAS PubMed.
- A. Ekpe and T. Jacobsen, Effect of Various Salts on the Stability of Lansoprazole, Omeprazole, and Pantoprazole as Determined by High-Performance Liquid Chromatography, Drug Dev. Ind. Pharm., 1999, 25, 1057–1065 CrossRef CAS PubMed.
- K. D. Altria, S. M. Bryant and T. A. Hadgett, Validated capillary electrophoresis method for the analysis of a range of acidic drugs and excipients, J. Pharm. Biomed. Anal., 1997, 15, 1091–1101 CrossRef CAS PubMed.
- A. Tivesten, S. Folestad, V. Schönbacher and K. Svensson, Nonaqueous capillary electrophoresis for the analysis of labile pharmaceutical compounds, Chromatographia, 1999, 49, S7–S11 CAS.
- A.-A. M. Wahbi, O. Abdel-Razak, A. A. Gazy, H. Mahgoub and M. S. Moneeb, Spectrophotometric determination of omeprazole, lansoprazole and pantoprazole in pharmaceutical formulations, J. Pharm. Biomed. Anal., 2002, 30, 1133–1142 CrossRef CAS PubMed.
- A. Radi, Anodic voltammetric assay of lansoprazole and omeprazole on a carbon paste electrode, J. Pharm. Biomed. Anal., 2003, 31, 1007–1012 CrossRef CAS PubMed.
- A. M. Qaisi, M. F. Tutunji and L. F. Tutunji, Acid decomposition of omeprazole in the absence of thiol: a differential pulse polarographic study at the static mercury drop electrode (SMDE), J. Pharm. Sci., 2006, 95, 384–391 CrossRef CAS PubMed.
- A. Ghaemi, S. Rayati, S. Zakavi and N. Safari, Highly efficient oxidation of sulfides to sulfones with tetra-n-butylammonium hydrogen monopersulfate catalyzed by β-tri- and tetra-brominated meso-tetraphenylporphyrinatomanganese(III) acetate, Appl. Catal., A, 2009, 353, 154–159 CrossRef CAS.
- K. Kaczorowska, Z. Kolarska, K. Mitka and P. Kowalski, Oxidation of sulfides to sulfoxides. Part 2: oxidation by hydrogen peroxide, Tetrahedron, 2005, 61, 8315–8327 CrossRef CAS.
- S. R. Jain, K. C. Adiga and V. R. Pai Verneker, A new approach to thermochemical calculations of condensed fuel-oxidizer mixtures, Combust. Flame, 1981, 40, 71–79 CrossRef CAS.
- M. Radpour, S. Alamolhoda and S. M. Masoudpanah, Effects of pH value on the microstructure and magnetic properties of solution combusted Fe3O4 powders, Ceram. Int., 2017, 43, 13729–13734 CrossRef CAS.
- B. Pourgolmohammad, S. M. Masoudpanah and M. R. Aboutalebi, Effect of starting solution acidity on the characteristics of CoFe2O4 powders prepared by solution combustion synthesis, J. Magn. Magn. Mater., 2017, 424, 352–358 CrossRef CAS.
- A. Shanmugavani, R. K. Selvan, S. Layek, L. Vasylechko and C. Sanjeeviraja, Influence of pH and fuels on the combustion synthesis, structural, morphological, electrical and magnetic properties of CoFe2O4 nanoparticles, Mater. Res. Bull., 2015, 71, 122–132 CrossRef CAS.
- T. Peng, X. Liu, K. Dai, J. Xiao and H. Song, Effect of acidity on the glycine–nitrate combustion synthesis of nanocrystalline alumina powder, Mater. Res. Bull., 2006, 41, 1638–1645 CrossRef CAS.
- R. Jenkins and R. L. Snyder, Introduction to X-ray powder diffractometry, John Wiley & Sons, Inc., New York, 1996 Search PubMed.
- P. F. Teh, Y. Sharma, Y. W. Ko, S. S. Pramana and M. Srinivasan, Tuning the morphology of ZnMn2O4 lithium ion battery anodes by electrospinning and its effect on electrochemical performance, RSC Adv., 2013, 3, 2812–2821 RSC.
- C. Lan, J. Zhao, L. Zhang, C. Wen, Y. Huang and S. Zhao, Self-assembled nanoporous graphene quantum dot-Mn3O4 nanocomposites for surface-enhanced Raman scattering based identification of cancer cells, RSC Adv., 2017, 7, 18658–18667 RSC.
- B. Yang, Z. Tian, B. Wang, Z. Sun, L. Zhang, Y. Guo, H. Li and S. Yan, Facile synthesis of Fe3O4/hierarchical-Mn3O4/graphene oxide as a synergistic catalyst for activation of peroxymonosulfate for degradation of organic pollutants, RSC Adv., 2015, 5, 20674–20683 RSC.
- M. Y. Nassar, A. S. Amin, I. S. Ahmed and S. Abdallah, Sphere-like Mn2O3 nanoparticles: facile hydrothermal synthesis and adsorption properties, J. Taiwan Inst. Chem. Eng., 2016, 64, 79–88 CrossRef CAS.
- M. Y. Nassar, M. M. Moustafa and M. M. Taha, Hydrothermal tuning of the morphology and particle size of hydrozincite nanoparticles using different counterions to produce nanosized ZnO as an efficient adsorbent for textile dye removal, RSC Adv., 2016, 6, 42180–42195 RSC.
- Z. N. Kayani, F. Nazir, S. Riaz and S. Naseem, Structural, optical and magnetic properties of manganese zinc oxide thin films prepared by sol–gel dip coating method, Superlattices Microstruct., 2015, 82, 472–482 CrossRef CAS.
- J. Zhang, J. Du, H. Wang, J. Wang, Z. Qu and L. Jia, A novel mild route to hausmannite Mn3O4 nanocubes at room temperature and its catalytic performance, Mater. Lett., 2011, 65, 2565–2567 CrossRef CAS.
- H. M. Aly, M. E. Moustafa, M. Y. Nassar and E. A. Abdelrahman, Synthesis and characterization of novel Cu(II) complexes with 3-substituted-4-amino-5-mercapto-1,2,4-triazole Schiff bases: a new route to CuO nanoparticles, J. Mol. Struct., 2015, 1086, 223–231 CrossRef CAS.
- M. Mostafa, H. M. Saber, A. A. El-Sadek and M. Y. Nassar, Preparation and performance of 99Mo/99mTc chromatographic column generator based on zirconium molybdosilicate, Radiochim.
Acta, 2016, 257–265 CAS.
- M. Y. Nassar, A. S. Attia, K. A. Alfallous and M. F. El-Shahat, Synthesis of two novel dinuclear molybdenum(0) complexes of quinoxaline-2,3-dione: new precursors for preparation of α-MoO3 nanoplates, Inorg. Chim. Acta, 2013, 405, 362–367 CrossRef CAS.
- M. Y. Nassar, A. A. Ali and A. S. Amin, A facile Pechini sol-gel synthesis of TiO2/Zn2TiO2/ZnO/C nanocomposite: an efficient catalyst for the photocatalytic degradation of orange G textile dye, RSC Adv., 2017, 7, 30411–30421 RSC.
- M. Y. Nassar and E. A. Abdelrahman, Hydrothermal tuning of the morphology and crystallite size of zeolite nanostructures for simultaneous adsorption and photocatalytic degradation of methylene blue dye, J. Mol. Liq., 2017, 242, 364–374 CrossRef CAS.
- P. Pascariu, A. Airinei, M. Grigoras, N. Fifere, L. Sacarescu, N. Lupu and L. Stoleriu, Structural, optical and magnetic properties of Ni doped SnO2 nanoparticles, J. Alloys Compd., 2016, 668, 65–72 CrossRef CAS.
- C. Sánchez, E. Flores, M. Barawi, J. M. Clamagirand, J. R. Ares and I. J. Ferrer, Marcasite revisited: optical absorption gap at room temperature, Solid State Commun., 2016, 230, 20–24 CrossRef.
- P. Kowalski, K. Mitka, K. Ossowska and Z. Kolarska, Oxidation of sulfides to sulfoxides. Part 1: oxidation using halogen derivatives, Tetrahedron, 2005, 61, 1933–1953 CrossRef CAS.
- A. El-Maaboud and I. Mohamed, Utility of diphenylamine and N-bromosuccinimide for colorimetric determination of certain phenothiazine drugs, Talanta, 1997, 44, 1173–1182 CrossRef CAS PubMed.
- E. Chikwana, B. Davis, M. K. Morakinyo and R. H. Simoyi, Oxyhalogen–sulfur chemistry — kinetics and mechanism of oxidation of methionine by aqueous iodine and acidified iodate, Can. J. Chem., 2009, 87, 689–697 CrossRef CAS.
- F. A. El-Yazbi and S. M. Blaih, Spectrophotometric and titrimetric determination of clindamycin hydrochloride in pharmaceutical preparations, Analyst, 1993, 118, 577–579 RSC.
- K. Basavaiah, V. Ramakrishna, U. R. Anil kumar and B. C. Somashekar, Spectrophotometric determination of lansoprazole in pharmaceuticals using bromate-bromide mixture based on redox and complexation reactions, Ecletica Quim., 2007, 32, 57–64 CrossRef CAS.
- I. F. Al-Momani and M. H. Rababah, Validation of HPLC and FIA Spectrophotometric Methods for the Determination of Lansoprazole in Pharmaceutical Dosage Forms and Human Plasma, Am. J. Anal. Chem., 2010, 01, 6 Search PubMed.
- K. Basavaiah, V. Ramakrishna and U. Kumar, Use of ceric ammonium sulphate and two dyes, methyl orange and indigo carmine, in the determination of lansoprazole in pharmaceuticals, Acta Pharmaceutica, 2007, 211 CAS.
- A. A. Syed and A. Syeda, Spectrophotometric determination of certain benzimidazole proton pump inhibitors, Indian J. Pharm. Sci., 2008, 70, 507–510 CrossRef CAS PubMed.
- C. S. P. Sastry, P. Y. Naidu and S. S. N. Murty, Spectrophotometric methods for the determination of omeprazole in bulk form and pharmaceutical formulations, Talanta, 1997, 44, 1211–1217 CrossRef CAS PubMed.
|
This journal is © The Royal Society of Chemistry 2017 |