DOI:
10.1039/C7RA02346D
(Paper)
RSC Adv., 2017,
7, 17346-17352
Integration of heterologous 4-hydroxybenzoic acid transport proteins in Rhodobacter sphaeroides for enhancement of coenzyme Q10 production
Received
25th February 2017
, Accepted 13th March 2017
First published on 20th March 2017
Abstract
The highly redox-active compound, Coenzyme Q10 (CoQ10), has attracted increasing interest due to its clinical benefits and important applications in the pharmaceutical, food and health industries. Although the metabolic pathways involved in CoQ10 biosynthesis are known, very few reports are available concerning the development of engineering strategies to enhance CoQ10 production in the natural producer Rhodobacter sphaeroides. In this work, three membrane transport proteins, AcPcaK, KpPcaK, and CgPcaK from different organisms (Acinetobacter calcoaceticus, Klebsiella pneumoniae and Corynebacterium glutamicum, respectively) were individually heterologously expressed in R. sphaeroides GY-2 to enhance the uptake of extracellular 4-hydroxybenzoic acid (4HBA), an important intermediate of CoQ10 synthesis. Each of the PcaK open reading frames (ORFs) was inserted behind an RFP gene to weaken their expression in order to avoid inhibition of cell growth. The transport efficiency and consumption of 4HBA, as well as CoQ10 productivity of the resulting recombinant R. sphaeroides GY-2 strains has been studied. The CoQ10 productivity of RS-CgPcaK and RS-KpPcaK was effectively improved by the addition of external 4HBA, reaching maximum values of 17.45 and 18.06 mg g−1 DCW (18.05% and 20.82% higher than R. sphaeroides GY-2), respectively. The strategy used in this study proved effective for enhancing the biotechnological production of CoQ10 and thus holds promise for further improvements, including industrial applications.
Introduction
The ubiquinone Coenzyme Q10 (CoQ10) is composed of a benzoquinone “head” group and a side chain composed of ten isoprenoid units, which distinguishes it from other CoQn cognates. CoQ10 is embedded within the hydrophobic domain of the phospholipid bilayer of cytoplasmic and organellar membranes, where it functions as an electron carrier in the respiratory chain.1,2 CoQ10 plays an important role as a redox-active molecule in the formation of disulfide bonds, as an antioxidant for removing harmful reactive oxygen species, and as a controller of the cellular redox status.3–5 The clinical benefits of CoQ10 supplementation include the prevention of heart failure and acute myocardial infarction in cardiovascular diseases, as well as positive effects in mitochondrial and certain neurodegenerative diseases and diabetes.6,7 Furthermore, CoQ10 acts as a special regulator of the genes involved in cholesterol metabolism and inflammatory responses.8 Over the past two decades, there has been growing interest in the research and production of CoQ10 due to these health-promoting effects which it exerts either as a food additive or medicine. Some prokaryotic microorganisms, such as Rhodobacter sphaeroides and Agrobacterium tumefaciens, have been studied by researchers as natural producers of CoQ10.9–11 In prokaryotic cells, the biosynthesis of CoQ10 utilizes three combined pathways. The benzoquinone “head” is synthesized from 4-hydroxybenzoic acid (4HBA) in the shikimate pathway, whereas the isoprenoid side chain is synthesized by an IPP synthesis enzyme, using ten isopentenyl pyrophosphate (IPP) units derived from pyruvate and glyceraldehyde-3-phosphate via the methylerythritol phosphate (MEP) pathway. Finally, CoQ10 is assembled by linking the isoprenoid side chain to the benzoquinone head in the ubiquinone pathway.
This detailed knowledge of the key genes and metabolic pathways involved in CoQ10 biosynthesis has made it possible to develop rational genetic engineering strategies to enhance the CoQ10 productivity of prokaryotic microorganisms. For instance, improved CoQ10 production was obtained by overexpressing the native rate-limiting enzymes of the MEP and ubiquinone pathways in R. sphaeroides.11,12 However, most relevant studies were carried out using E. coli as the CoQ10 producer, owing to its ease of genetic manipulation.2,5,13–15 The specific productivity of CoQ10 achieved by these metabolically engineered E. coli strains was very low and not comparable to the natural CoQ10 producers such as R. sphaeroides, A. tumefaciens and R. radiobacter.16,17 Thus, it seems particularly attractive and practical to directly genetically modify R. sphaeroides and improve upon its intrinsically high CoQ10 titer.
In the present study, we focused on increasing the supplementation and availability of 4HBA, the initial precursor of the ubiquinone pathway, which we predicted would result in enhanced CoQ10 production in R. sphaeroides. PcaK is a transporter and chemoreceptor membrane protein which is known to facilitate the transmembrane transport of 4HBA in Pseudomonas putida and Klebsiella pneumoniae.18,19 In this study, three PcaK homologs, those derived from Acinetobacter calcoaceticus ATCC 14987 (AcPcaK, Genbank accession no. KUM11311.1), Klebsiella pneumoniae ATCC 33495 (KpPcaK, Genbank accession no. KUP78028.1) and Corynebacterium glutamicum ATCC 13032 (CgPcaK, Genbank accession no. BAV23994.1), have been expressed in R. sphaeroides GY-2, a high-yield CoQ10 producing mutant strain obtained from R. sphaeroides 2.4.1 (ATCC 17023) in our previous work. We sought to improve the CoQ10 productivity in R. sphaeroides GY-2 by extracellularly supplementing 4HBA, which would then be transported into the cells by the overexpressed PcaK. However, the growth and metabolism of many microorganisms can be greatly inhibited by species-specific concentrations of 4HBA.20 To circumvent this obstacle, each of the PcaK reading frames has been inserted behind a red fluorescent protein (RFP) gene under the control of the tac promoter (Fig. 1), in order to control for the effects of 4HBA on biomass accumulation and the utilization of supplemented 4HBA for the synthesis of CoQ10 in the resulting recombinant R. sphaeroides strains. This work thus provides a novel genetic engineering strategy for the improvement of CoQ10 production in R. sphaeroides and other industrially relevant microorganisms.
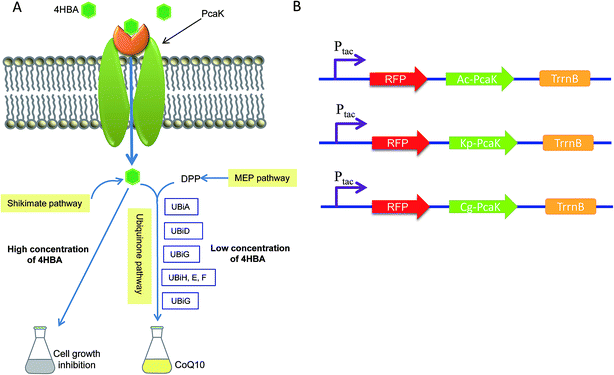 |
| Fig. 1 Schematic presentation of the PcaK functions in CoQ10 biosynthesis. (A) PcaK-mediated membrane transport of 4HBA. (B) Construction of the expressing fragments of PcaK in this study. DPP: decaprenyl diphosphate; 4HBA: 4-hydroxybenzoic acid; UbiA: 4HBA-polyprenyltransferase; UbiD, B, E, F, G, H: quinoid ring modification enzymes. | |
Experimental
Strains, media and culture conditions
The strains and plasmids used in this study are listed in Table 1. E. coli strains were cultivated at 37 °C in Luria-Bertani (LB) medium supplemented with 50 mg ml−1 of kanamycin sulfate when necessary. E. coli DH5α was used for cloning and plasmid construction and E. coli S17-1 was used for bi-parental conjugation. R. sphaeroides GY-2 is a mutant strain derived from R. sphaeroides 2.4.1 (ATCC 17023) via screening, which has a high yield of CoQ10. The specific culture medium used for R. sphaeroides GY-2 comprised 4 g l−1 glucose, 8 g l−1 yeast extract, 5 g l−1 NaCl, 2 g l−1 MgCl2, 3 g l−1 KH2PO4, 1 g l−1 CaCl2, 1 g l−1 glutamic acid, 0.4 g l−1 aspartic acid, 2 g l−1 nitrilotriacetic acid, 1 g l−1 nicotinic acid (Amresco Ltd., Solon, US), and 1 g l−1 biotin (Sigma-Aldrich, St Louis, US), with an initial pH of 4.5–5.5.
Table 1 Strains, plasmids and primers used in this study
Strain |
Genotype/description |
Reference |
E. coli DH5a |
F-λ-endA1 glnV44 thi-1 recA1 relA1 gyrA96 deoR nupG Φ80dlacZΔM15 Δ(lacZYA-argF)U169, hsdR17(rK− mK+) |
Invitrogen |
E. coli S17-1 |
RP4-2. Tc::Mu-Km::Tn7 |
21 |
R. sphaeroides-GY2 |
CoQ10 high-yield mutant strain obtained from R. sphaeroides 2.4.1 |
This study |
Acinetobacter calcoaceticus |
Genomic source of Ac-Pcak |
ATCC 14987 |
Klebsiella pneumoniae |
Genomic source of Kp-Pcak |
ATCC 33495 |
Corynebacterium glutamicum |
Genomic source of Cg-Pcak |
ATCC 13032 |
Plasmid |
Description |
Reference |
pYC6a-RFP |
pYC6a derivative containing RFP, AmpR |
This study |
pBBR1MCS2 |
Low-copy cloning vector, KanR |
22 |
pBBR1MCS2-tac-RFP |
pBBR1MCS2 derivative expression plasmid with the tac promoter and RFP |
This study |
pBBR1MCS2-tac-RFP derivative containing Pcak from C. glutamicum |
pBBR1MCS2-RFP-CgPcak |
This study |
pBBR1MCS2-RFP-AcPcak |
pBBR1MCS2-tac-RFP derivative containing Pcak from A. calcoaceticus |
This study |
pBBR1MCS2-RFP-KpPcak |
pBBR1MCS2-tac-RFP derivative containing Pcak from K. pneumoniae |
This study |
Primers used in this study |
Designation |
Sequence (5′-3′) |
acPcaK-F |
CCGGAATTCATGGCGAAAACGATAGATGCAA |
acPcaK-R |
CGCGGATCCTTATAGACTTTCTTCGAGCTTTAA |
kpPcaK-F |
CCGGAATTCATGACACAGACTCAACGCCTGGATGTCA |
kpPcaK-R |
CGCGGATCCCTATGACGCTCCGCGCACAGACGCGG |
cgPcaK-F |
CCGGAATTCATGGCGTCAACGACCACCCCAACCCGCG |
cgPcaK-R |
CGCGGATCC TTAAGCGTTCTCGCGATGCCTCAGAGTCTT |
tRFP-F |
ATTCCCCGCGGCACAGCTAACACCACGTCGTC |
tRFP-R |
GAGCCCAAGCTTGAAAGGCCCAGTCTTTCGAC |
The fermentation medium comprised 32 g l−1 glucose, 4 g l−1 corn steep liquor (Beijing Chemical Works, China), 6.5 g l−1 glutamic acid, 4 g l−1 (NH4)2SO4, 3.25 g l−1 NaCl, 10 g l−1 MgSO4, 1.4 g l−1 FeSO4, 1.2 g l−1 KH2PO4, 0.06 g l−1 MnSO4, 0.06 g l−1 CoCl2, 8 g l−1 CaCO3, 1 g l−1 nicotinic acid, and 0.3 g l−1 biotin.
Construction of vectors
pBBR1MCS2 is a low-copy-number broad-host-range vector for DNA cloning in Gram-negative bacteria.23 The expression plasmid pBBR1MCS2-tac-RFP is derived from pBBR1MCS2 and pYC6a-RFP. The fragment containing the tac promoter, RFP and the TrrnB terminator was amplified from pYC6a-RFP using primers tRFP-F and tRFP-R, the resulting fragment digested using the restriction endonucleases SacII and HindIII, and ligated into pBBR1MCS2, yielding the plasmid pBBR1MCS2-tac-RFP. The genes encoding AcPcak, CgPcak and KpPcak were amplified using the primers listed in Table 1, the resulting fragments digested using the restriction endonucleases EcoRI and BamHI, and ligated into pBBR1MCS2-tac-RFP, yielding pBBR1MCS2-RFP-CgPcak, pBBR1MCS2-RFP-AcPcak, and pBBR1MCS2-RFP-KpPcak, respectively. To avoid the formation of a fusion protein comprising Pcak and RFP, a stop codon was added to the end of the open reading frame of RFP. The Q5 polymerase, endonucleases, and T4 ligase used in this study were all purchased from New England Biolabs (Beijing, China).
Bi-parental conjugation
The plasmids pBBR1MCS2-tac-RFP, pBBR1MCS2-RFP-CgPcak, pBBR1MCS2-RFP-AcPcak and pBBR1MCS2-RFP-KpPcak were used to transform E. coli S17-1 via electroporation, and the resulting strains were used as plasmid donors. The process of conjugation mating was carried out as described in a previous study.24 Briefly, cultures of E. coli S17-1 (donor) and R. sphaeroides GY-2 (recipient) were grown to mid-log phase, harvested by centrifugation (8000g, 5 min) and resuspended in fresh LB medium. The E. coli S17-1 and R. sphaeroides GY-2 cell suspensions were mixed at 1
:
6 and 1
:
10 ratios, the resulting mixtures of the two strains harvested, transferred to an LB agar plate, and incubated for 20–24 h at 30 °C. The resulting bacterial lawns were harvested by washing with 0.1 ml pre-cooled improved Sistrom's minimal medium25 and spread on LB agar plates containing 200 mg l−1 K2TeO3 and 50 mg ml−1 kanamycin. Kanamycin-resistant black colonies were isolated, verified, and utilized for further experiments. The recombinant R. sphaeroides GY-2 harboring pBBR1MCS2-tac-RFP was used as the control.
Western blot analysis
Western blot analysis was employed to detect the expression of the recombinant PcaK in R. sphaeroides GY-2. Briefly, R. sphaeroides cells cultured for 24 h in the specific medium containing 50 mg l−1 kanamycin were collected, re-suspended, and washed with sterile water. Membrane proteins of the recombinant strains were extracted using the Membrane Extraction Kit (Sigma-Aldrich, St Louis, US) according to the manufacturer's protocol. The resulting crude membrane extracts were further separated by SDS-PAGE on 12% polyacrylamide gels and transferred to an Immun-Blot assay kit membrane (Bio-Rad, Beijing, China) via semi-dry electrotransfer. The six histidine-tagged fusions of PcaK were probed with a mouse anti-His6 antibody and a secondary HRP-labeled goat anti-mouse IgG (1 μg ml−1; Amersham, Beijing, China). The PVDF membrane was washed with 1× TBST (0.1% Tween 20) 4 times and the blotting result was visualized using a near-infrared fluorescence detector (Bio-Rad, Beijing, China).
CoQ10 production in shake-flask cultures
The recombinant R. sphaeroides GY-2 strains were inoculated and grown in the specific medium at pH 6.4 and 32 °C, under constant orbital shaking at 200 rpm for 24 h. The strains were then transferred to 500 ml baffled flasks containing 150 ml of fermentation medium at 32 °C and pH 6.3–6.4 under constant orbital shaking 220 rpm for 48 h. After the fermentation process, 0.5–1 ml aliquots of the fermentation broth were harvested, combined 200 μl HCl solution (6 mol l−1), and incubated at 65 °C for 30 min. Subsequently, 2 ml acetone and 100 μl of a 30% hydrogen peroxide solution were added and mixed well. The mixture was collected, filled up to 10 ml with ethanol, and incubated in an ultrasonic bath at 4 °C for 45 min. Pellets were harvested and centrifuged at 8000g for 10 min. The resulting supernatants were collected and examined for CoQ10 production.
HPLC analysis
CoQ10 production and 4HBA consumption were determined using an UltiMate TM 3000 HPLC system (Thermo-Fisher Scientific, Waltham, US) equipped with a 250 mm × 4.6 mm × 5 μm, C18-reversed phase column (Thermo-Fisher Scientific, Beijing, China) and a photo-diode array detector. Methanol/isopropyl alcohol (HPLC grade, Sigma-Aldrich, Shanghai, China) at a ratio of 3
:
1 (v/v) was used as the mobile phase at a flow rate of 0.8 ml min−1 and the column was kept at 30 °C. CoQ10 was detected at 275 nm and 254 nm. CoQ10 concentrations were determined using a standard curve based on an HPLC-grade authentic CoQ10 standard (Sigma-Aldrich, Shanghai, China). 4HBA was resolved using 15% acetonitrile with 0.1% trifluoroacetic acid (TFA) in water at a flow rate of 0.8 ml min−1, on a HyperREZ XP 300 mm × 7.7 mm × 8 μm column (Thermo-Fisher Scientific, Beijing, China) which was kept at 65 °C, and detected at 254 nm. The concentration of 4HBA was quantified using a standard curve based on authentic standard material (Sigma-Aldrich, Shanghai, China).
Results and discussion
Expression of three different PcaK homologs in R. sphaeroides GY-2
The PcaK proteins derived from Acinetobacter calcoaceticus and Klebsiella pneumoniae have been functionally identified as plasma membrane transporter of 4HBA.19,26 Since PcaK-mediated transport is active and energized by the proton motive force, we hypothesized that this protein would significantly increase 4HBA transport in other bacteria as well. In this study, three PcaK homologs, derived from Acinetobacter calcoaceticus, Klebsiella pneumoniae, and Corynebacterium glutamicum, were used to improve CoQ10 production and introduced on plasmids into R. sphaeroides GY-2 via the bi-parental conjugation method. However, high-level expression of PcaK led to excessive accumulation of 4HBA, resulting in inhibition of cell growth and CoQ10 production (Fig. 1). Because tac acts as a strong promoter, the transcriptional level of the PcaK genes could be weakened when they were inserted behind an RFP open reading frame. Expression of each PcaK homolog in R. sphaeroides GY-2 was verified by PCR and western blot assays. The recombinant three R. sphaeroides strains expressing CgPcak, KpPcak, and AcPcak were named as RS-CgPcaK, RS-KpPcaK, and RS-AcPcaK, respectively. The R. sphaeroides GY-2 containing pBBR1MCS2-tac-RFP (RS-GY2) was used as the control.
As shown in Fig. 2A, the results clearly show the presence of the recombinant PcaK in each of the three R. sphaeroides GY-2 strains. Fig. 2B illustrates the three bands corresponding to the respective PcaK proteins, each with a molecular weight of about 32 kDa. Even though the observed molecular weight was substantially lower than the theoretical prediction of 47 kDa, it was consistent with a report by Pernstich et al.26 The results indicate that none of the heterologous proteins were significantly modified by R. sphaeroides GY-2. In other words, their normal biological function should not be affected in this host organism.
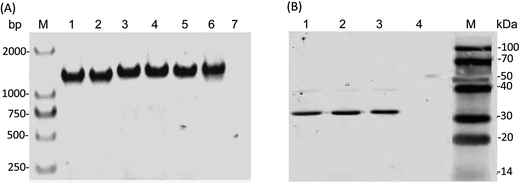 |
| Fig. 2 Integration of three PcaK transporters into R. sphaeroides GY-2. (A) PCR verification of PcaK genes from the three recombinant R. sphaeroides GY-2 strains. Lanes 1–2 represent gene of Cg-Pcak; lanes 3–4 represent gene of Ac-Pcak; lanes 5–6 represent gene of Kp-Pcak; lane 7 represents the control; M: DNA marker. (B) Detection of PcaK in the three recombinant R. sphaeroides GY-2 strains by western blot. Lanes 1–3 represent CgPcaK, AcPcaK, and KpPcaK, respectively. | |
Effect of 4HBA on biomass accumulation
Most Gram-positive and some Gram-negative bacteria were found to be considerably sensitive to 4HBA.20 Generally, it has been observed that the growth of bacteria is markedly inhibited when the concentration of 4HBA rises above 1 mol l−1. To elucidate the effect of 4HBA on the growth of R. sphaeroides, the cells were cultured in the specific medium supplemented with 0.5, 1, 2, and 4 mol l−1 of 4HBA, respectively (Fig. 3). It was found that all three recombinant strains expressing foreign PcaK proteins were more sensitive to 4HBA than RS-GY2, which was used as control. Among the three recombinant strains, the growth of RS-CgPcaK was most susceptible to inhibition by 4HBA. The biomass yield of RS-CgPcaK was lower by 12.8–19.1% at the different 4HBA concentrations, compared to the control. Interestingly, a low concentration of 4HBA (0.5–1 mol l−1) contributed to increased biomass accumulation of all the R. sphaeroides strains. However, biomass ceased to increase and began to decline when more than 1 mol l−1 of 4HBA was added to the medium.
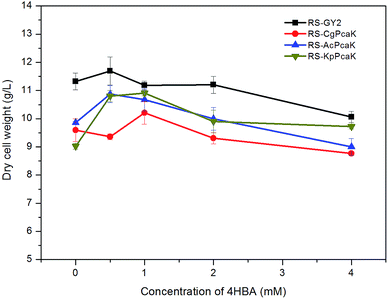 |
| Fig. 3 Effect of different concentrations of 4HBA on cell growth of the recombinant R. sphaeroides GY-2 strains. The R. sphaeroides GY-2 containing pBBR1MCS2-tac-RFP (RS-GY2) was used as the control strain. Data were presented as means ± standard deviation (SD). | |
In fact, the biological function of CgPcaK, AcPcaK, and KpPcaK could be demonstrated via the increased 4HBA transport efficiencies and consumption rates exhibited by the three recombinant R. sphaeroides GY-2 strains. Theoretically, the PcaK with the highest transport efficiency should most effectively facilitate the transmembrane transportation of 4HBA, and consequently, the cell growth of the corresponding R. sphaeroides GY-2 strain should be inhibited the most. As expected, all the recombinant strains expressing PcaK showed higher rates of growth inhibition at different concentrations of 4HBA than the control strain (Fig. 3). Thus, it can be concluded that the PcaK proteins from different sources indeed mediate 4HBA transport in R. sphaeroides GY-2. CgPcaK appeared to have the highest transport efficiency, since its growth was inhibited the most at the different initial concentrations of 4HBA. This conclusion was confirmed by the observation that RS-CgPcaK also consumed significantly more 4HBA than the control strain (Fig. 4). All the data in this study was compared using t-test statistics.
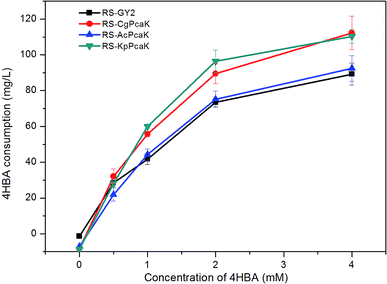 |
| Fig. 4 4HBA consumption of the recombinant R. sphaeroides GY-2 strains. RS-GY2 was used as the control strain. Data were presented as means ± standard deviation (SD). | |
4HBA consumption by the recombinant R. sphaeroides
To determine the transport efficiency of the heterologously expressed PcaK, the 4HBA consumption and utilization by the recombinant R. sphaeroides strains was studied. Fig. 4 shows the 4HBA consumption of the recombinant R. sphaeroides GY-2 strains at four different 4HBA concentrations. The consumption was calculated based on the difference between the initial and remaining concentrations of 4HBA after a fixed period of time. The four R. sphaeroides strains exhibited a similar pattern whereby the 4HBA consumption dramatically increased at the lower concentrations of 0.5–2 mol l−1, and increased slowly at 2–4 mol l−1 (Fig. 4). The reduced efficiency of 4HBA utilization at higher concentrations was probably due to inhibition of cellular metabolism. Both RS-CgPcaK and RS-KpPcaK consumed significantly more 4HBA than the control strain (P < 0.05) using t-test statistics. About 20% more 4HBA was transported into the cells contributing to CoQ10 biosynthesis at initial 4HBA concentrations of 1–4 mol l−1. However, there was no significant difference between RS-AcPcaK and the control strain for 4HBA consumption. Interestingly, a small amount of 4HBA could be detected even in the culture supernatants to which no 4HBA was supplemented. The improved 4HBA consumption of RS-CgPcaK and RS-KpPcaK can be easily understood in terms of the higher 4HBA trans-membrane transport efficiency of CgPcaK and KpPcaK compared to AcPcaK.
CoQ10 production
Batch fermentations were carried out in shake flasks to determine the specific contents of CoQ10 produced by the recombinant R. sphaeroides strains. The results illustrate that enhanced CoQ10 productivity has indeed been achieved in the RS-CgPcaK and RS-KpPcaK strains at an initial 4HBA concentration of 0.5 mol l−1, and was significantly higher than what was observed for any other concentration (P < 0.05) (Fig. 5B and D). After anaerobic fermentation for 48 h, the highest CoQ10 productivity values for RS-CgPcaK and RS-KpPcaK reached 17.45 mg g−1 DCW and 18.06 mg g−1 DCW, respectively. In contrast, 4HBA supplementation did not contribute to improved CoQ10 productivity in either the RS-AcPcaK strain or the RS-GY2 control strain (Fig. 5A and C). Furthermore, the CoQ10 productivity of the four R. sphaeroides GY-2 strains decreased rapidly at higher levels (≥1 mol l−1) of 4HBA, probably due to suppressed cell growth. Taken together, the results indicate that above a certain threshold, the higher the initial concentration of 4HBA that was added, the lower the CoQ10 content that was obtained.
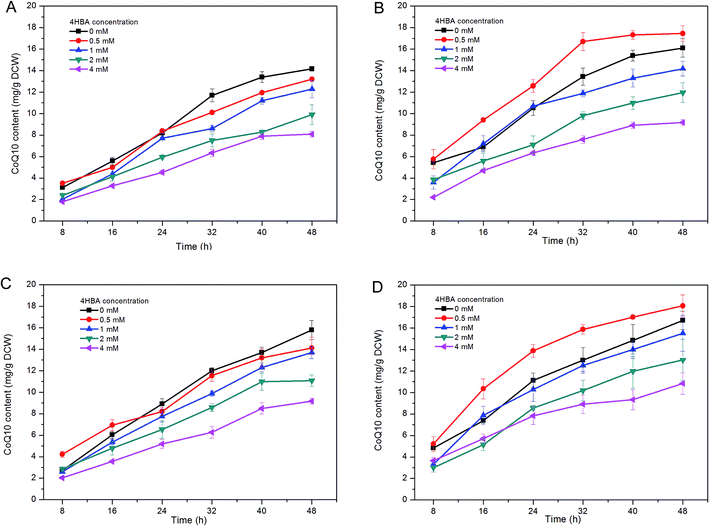 |
| Fig. 5 Batch fermentation results of the specific contents of CoQ10 produced by the four recombinant R. sphaeroides GY-2 strains at different concentration of 4HBA. (A) RS-GY2 (B) RS-CgPcaK (C) RS-AcPcaK (D) RS-KpPcaK. Data were presented as means ± standard deviation (SD). | |
The results in Fig. 5 illustrate the increased 4HBA consumption led to enhanced CoQ10 productivity. This is consistent with a previously published report that CoQ10 production could be improved by increasing the flux of precursors to the ubiquinone pathway in an engineered E. coli strain.2 RS-AcPcaK likely transports the most 4HBA into the cytoplasm, judging by the most significant inhibition of biomass accumulation compared to other recombinant R. sphaeroides strains (Fig. 3). However, we unexpectedly found that RS-AcPcaK failed to increase CoQ10 biosynthesis and 4HBA consumption. It is possible that the highly concentrated 4HBA transported by RS-AcPcaK is transformed to the inhibitory dihydroxybenzoic acid by undergoing ortho ring cleavage via the β-ketoadipate pathway,18 suggesting that the precursor flux to the ubiquinone pathway was not significantly increased and CoQ10 production was therefore also not enhanced.
High intracellular concentrations of aromatic carboxylic acids are not persistent in bacteria, since there is a highly-regulated efflux system that maintains the intracellular balance of 4HBA in E. coli and other microorganisms.27 Accordingly, when high-levels of 4HBA were transported into the R. sphaeroides cells, expression of the efflux system was likely activated, resulting in the removal of excess 4HBA and restoration of cellular homeostasis. In this study, a small amount (less than 8 mg l−1) of 4HBA was found in the culture supernatants not supplemented with external 4HBA, indicating that not all of the endogenously synthesized 4HBA was channeled into the ubiquinone pathway for CoQ10 production. As an intermediate, the transported 4HBA which accumulated to high intracellular levels prompted the reactions in the ubiquinone pathway. However, it was observed in this study that only an initial concentration of less than 0.5 mM 4HBA enhanced the biosynthesis of CoQ10 in R. sphaeroides. Excessive amounts of intracellular 4HBA are not only directly detrimental to cell growth and biomass accumulation, but probably need to be pumped out of the cells by the efflux system, introducing an additional energy drain.
Conclusions
Three membrane transport proteins, AcPcaK, KpPcaK, and CgPcaK, were individually heterologously expressed in R. sphaeroides GY-2 to enhance the uptake of extracellular 4HBA, which is an important intermediate for CoQ10 synthesis. The transport efficiency and consumption of 4HBA by these three recombinant R. sphaeroides GY-2 strains has been studied. To our best knowledge, this is the first reported instance of improved supplementation of an intermediate of the ubiquinone pathway to enhance CoQ10 production in the natural producer R. sphaeroides. This study confirms the effectiveness of the strategy used for improving CoQ10 production and will broaden its applications for further improvements.
Acknowledgements
This work was financially supported by National Natural Science Foundation of China (No. 21406130) and Natural Science Foundation of Fujian Province of China (No. 2016J01148; 2016J01147).
Notes and references
- W. M. Bader, T. Xie, C. A. Yu and J. C. Bardwell, J. Biol. Chem., 2000, 275, 26082–26088 CrossRef PubMed.
- C. P. Cluis, A. Ekins, L. Narcross, H. Jiang, N. D. Gold, A. M. Burja and V. J. Martin, Metab. Eng., 2011, 13, 733–744 CrossRef CAS PubMed.
- F. L. Crane, J. Am. Coll. Nutr., 2001, 20, 591–598 CrossRef CAS PubMed.
- D. A. Groneberg, B. Kindermann, M. Althammer, M. Klapper, J. Vormann, G. P. Littarru and F. Doring, Int. J. Biochem. Cell Biol., 2005, 37, 1208–1218 CrossRef CAS PubMed.
- H. S. Zahiri, S. H. Yoon, J. D. Keasling, S. H. Lee, K. S. Won, S. C. Yoon and Y. C. Shin, Metab. Eng., 2006, 8, 406–416 CrossRef CAS PubMed.
- J. M. Hodgson, G. F. Watts, D. A. Playford, V. Burke and K. D. Croft, Eur. J. Clin. Nutr., 2002, 56, 1137–1142 CrossRef CAS PubMed.
- H. N. Bhagavan and R. K. Chopra, Clin. Nutr., 2005, 24, 331–338 CrossRef CAS PubMed.
- C. Schmelzer, I. Lindner, C. Vock, K. Fujii and F. Doring, IUBMB Life, 2007, 59, 628–633 CrossRef PubMed.
- G. S. Choi, Y. S. Kim, J. H. Seo and Y. W. Ryu, Process Biochem., 2005, 40, 3225–3229 CrossRef CAS.
- N. B. Kien, I. S. Kong, M. G. Lee and J. K. Kim, J. Ind. Microbiol. Biotechnol., 2010, 37, 521–529 CrossRef CAS PubMed.
- W. Lu, L. Ye, H. Xu, W. Xie, J. Gu and H. Yu, Biotechnol. Bioeng., 2014, 111, 761–769 CrossRef CAS PubMed.
- W. Lu, Y. Shi, S. He, Y. Fei, K. Yu and H. Yu, Biochem. Eng. J., 2013, 72, 42–47 CrossRef CAS.
- S. J. Kim, M. D. Kim, J. H. Choi, S. Y. Kim, Y. W. Ryu and J. H. Seo, Appl. Microbiol. Biotechnol., 2006, 72, 982–985 CrossRef CAS PubMed.
- Y. C. Park, S. J. Kim, J. H. Choi, W. H. Lee, K. M. Park, M. Kawamukai, Y. W. Ryu and J. H. Seo, Appl. Microbiol. Biotechnol., 2005, 67, 192–196 CrossRef CAS PubMed.
- X. Lv, H. Xu and H. Yu, Appl. Microbiol. Biotechnol., 2012, 97, 2357–2365 CrossRef PubMed.
- M. J. Seo, M. C. Kook and S. O. Kim, J. Microbiol. Biotechnol., 2012, 22, 230–233 CrossRef CAS PubMed.
- Y. Yuan, Y. Tian and T. Yue, J. Biomed. Biotechnol., 2012, 2012, 607329 Search PubMed.
- N. N. Nichols and C. S. Harwood, J. Bacteriol., 1997, 179, 5056–5061 CrossRef CAS PubMed.
- J. L. Allende, M. Suarez, M. Gallego and A. Garrido-Pertierra, Arch. Biochem. Biophys., 1993, 300, 142–147 CrossRef CAS PubMed.
- J. Y. Cho, J. H. Moon, K. Y. Seong and K. H. Park, Biosci., Biotechnol., Biochem., 1998, 62, 2273–2276 CrossRef CAS PubMed.
- M. W. Wood, M. A. Jones, P. R. Watson, S. Hedges, T. S. Wallis and E. E. Galyov, Mol. Microbiol., 1998, 29, 883–891 CrossRef CAS PubMed.
- M. E. Kovach, P. H. Elzer, D. S. Hill, G. T. Robertson, M. A. Farris, R. M. II Roop and K. M. Peterson, Gene, 1995, 166, 175–176 CrossRef CAS PubMed.
- S. L. Porter, G. H. Wadhams and J. P. Armitage, Methods Enzymol., 2007, 423, 392–413 CAS.
- M. D. Moore and S. Kaplan, J. Bacteriol., 1992, 174, 1505–1514 CrossRef CAS PubMed.
- D. A. D'Argenio, A. Segura, W. M. Coco, P. V. Bünz and L. N. Ornston, J. Bacteriol., 1999, 181, 3505–3515 Search PubMed.
- C. Pernstich, L. Senior, K. A. MacInnes, M. Forsaith and P. Curnow, Protein Expression Purif., 2014, 101, 68–75 CrossRef CAS PubMed.
- T. K. Van Dyk, L. J. Templeton, K. A. Cantera, P. L. Sharpe and F. S. Sariaslani, J. Bacteriol., 2004, 186, 7196–7204 CrossRef CAS PubMed.
Footnote |
† Feng Qi and Limei Zou contributed equally to this work. |
|
This journal is © The Royal Society of Chemistry 2017 |