DOI:
10.1039/C7RA00337D
(Paper)
RSC Adv., 2017,
7, 18650-18657
Two pairs of Zn(II) coordination polymer enantiomers based on chiral aromatic polycarboxylate ligands: synthesis, crystal structures and properties†
Received
9th January 2017
, Accepted 23rd March 2017
First published on 28th March 2017
Abstract
Two pairs of Zn-based chiral coordination polymers (CCPs), namely, [Zn((R)-cbca)·H2O], [Zn((S)-cbca)]·H2O], [Zn((R)-cna)·2H2O] and [Zn((S)-cna)·2H2O] {H2cbca = (R) or (S)-4′-(1-carboxyethoxy)-[1,1′-biphenyl]-4-carboxylic acid, H2cna = (R) or (S)-6-(1-carboxyethoxy)-2-naphthoic acid} were synthesized under hydrothermal conditions. Their structures were determined by single-crystal X-ray diffraction analysis and further characterized by elemental analysis, infrared spectroscopy, powder X-ray diffraction, circular dichroism (CD) spectroscopy. CCPs 1–4 crystallized in chiral space groups and the CD spectra demonstrate obvious positive or negative Cotton effects. Second-harmonic generation (SHG) measurements show that CCPs 1–4 are SHG active and the SHG efficiencies are 0.6, 0.6, 0.5 and 0.5 times as much as that of urea, respectively. CCPs 1–4 exhibit relatively high luminescence properties originating from ligand centered emission.
Introduction
As an emerging class of molecule-based hybrid materials, coordination polymers (CPs) have presented a promising prospect for various applications, including catalysis, recognition of small molecules, separation, magnetism, and luminescence.1 In particular, chiral coordination polymers (CCPs) appear to be excellent candidates in the fields of enantiomorph separation, drug delivery, asymmetric catalysis, luminescence and sensors for their tunable structures, active sites with different strengths and their uniform size of channels or cavities present in the frameworks.2 It is believed that the properties of CCPs have a direct relationship with their structures. However, the rational design and construction of coordination polymers (CPs) is still a continuing challenge, representing an ambitious goal for chemists.
At present, adopting homochiral ligands as building blocks has been proved to be the most reliable and effective way in the construction of CCPs.3 Accordingly, how to design and obtain enantiopure ligands is of particular importance. Among different types of organic linkers, carboxylate donor ligands with strong coordination ability could satisfy various geometric requirements of metal centers by their diverse coordinating modes, which are capable of generating intriguing frameworks with high dimensions and interesting topologies.4 The design and synthesis of new polycarboxylate based ligands have been one of ongoing research in our laboratory.5 As an inexpensive, nontoxic and readily available material, lactic acid could be used as an ideal chiral source to incorporate chiral functional group to an organic ligand.6
Taking these into account, we presented here two pairs of chiral dicarboxylic acid enantiomers, namely, (R) or (S)-4′-(1-carboxyethoxy)-[1,1′-biphenyl]-4-carboxylic acid and (R) or (S)-6-(1-carboxyethoxy)-2-naphthoic acid ((R)-H2cbca, (S)-H2cbca, (R)-H2cna and (S)-H2cna) (Scheme 1), which are synthesized from homochiral lactic acid reacting with methyl 4′-hydroxy-[1,1′-biphenyl]-4-carboxylate and methyl 6-hydroxy-2-naphthoate, respectively. The design of the two pairs of new chiral ligands is also based on the following consideration: two structural units of naphthalene and diphenyl groups are introduced into the two pairs of ligands for comparison, as such structural diversity endows the two ligands different lengths and rigidness. It might be interesting and meaningful to investigate whether such slight difference in the ligands could have certain impact on the structures and properties of the eventual CCPs or not. We have now successfully prepared two pairs of Zn-based CCPs, namely, [Zn((R)-cbca)]·H2O], [Zn((S)-cbca)]·H2O], [Zn((R)-cna)·2H2O] and [Zn((S)-cna)·2H2O] via the hydrothermal method. Herein, we report their synthesis, crystal structures, thermal stabilities, CD spectra, luminescence and second-order nonlinear optical properties.
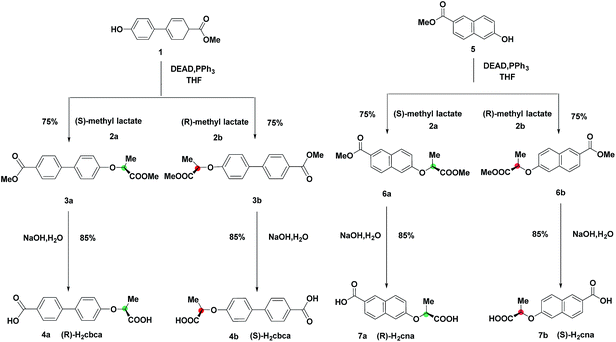 |
| Scheme 1 Synthetic routes of the ligands (R)-H2cbca, (S)-H2cbca, (R)-H2cna and (S)-H2cna. | |
Experimental
Materials and measurements
All commercially available chemicals were reagent grade and used as received. Elemental analyses of C, H and N were performed on a Perkin-Elmer 2400 elemental analyzer. IR spectra were recorded with a Spectrum one Fourier transform infrared (FT-IR) spectrometer using KBr pellets in the range of 400–4000 cm−1. The power X-ray diffractions (PXRD) data of the samples was collected on a Rigaku D/MAX-3B diffractometer using Cu-Kα radiation (λ = 1.5418 Å) and 2θ ranging from 5 to 50°. Thermal analyses were conducted on a Perkin-Elmer STA 6000 with a heating rate of 10 °C min−1 in a temperature range from 48 to 800 °C under air atmosphere. The circular dichroism spectra (CD) of CCPs 1–4 were recorded at room temperature with a Jasco J-810(S) spectropolarimeter (KBr pellets). The luminescent spectrum was taken on a Perkin Elemer Corporation Model Fluorescence Spectrometer LS 55 PL. PL spectra were performed in solid samples after the crystals were solved in methanol. The second-order nonlinear optical intensities were estimated by measuring microcrystalline samples relative to urea by a Spectra Physics Quanta Ray Prolab 170 Nd:YAG laser using the first-harmonics output of 1064 nm with a pulse width of 10 ns and a repetition rate of 10 Hz.7
Synthesis
Synthesis of methyl 4′-((1-methoxy-1-oxopropan-2-yl)oxy)-[1,1′-biphenyl]-4-carboxylate (3a and 3b). Methyl 4′-hydroxy-[1,1′-biphenyl]-4-carboxylate (1, 4.6 g, MW = 228.25, 0.020 mol), (S)-methyl lactate or (R)-methyl lactate (2, 2.2 g, MW = 104.11, 0.021 mol), triphenylphosphine (5.8 g, MW = 262.29, 0.022 mol) were mixed in dry 50 mL THF, to which diethyl diazocarboxylate (3.8 g, MW = 174.15, 0.022 mol) in 10 mL THF was dropwise added over 10 min at 0 °C. After stirring for 2 h at the same temperature, the reaction mixture was concentrated. Then, the residue was purified by flash column chromatography on silica gel eluting with 20% ethyl acetate in petroleum ether to give 3a or 3b (4.8 g, MW = 314.34, 0.015 mol, 76%) as a white solid.
Synthesis of 4′-(1-carboxyethoxy)-[1,1′-biphenyl]-4-carboxylic acid (4a and 4b). 4′-((1-Methoxy-1-oxopropan-2-yl)oxy)-[1,1′-biphenyl]-4-carboxylate (3a or 3b, 6.3 g, MW = 314.34, 0.020 mol), 10 mL methanol, 40 mL water and solid sodium hydroxide (1.2 g, 0.030 mol) were added to a 100 mL round-bottomed flask containing a stirring bar. The reaction mixture was stirred and heated at 50 °C for 10 h, then the result solution was acidified to pH 1–2 with concentrated aqueous HCl in an ice bath. The precipitated solid was separated by filtration to give pure compound 4a or 4b (4.9 g, MW = 286.28, 0.017 mol, 85%) as a white solid. 1H NMR (400 MHz, DMSO-d6), δ (ppm): 1.53 (d, J = 6.78 Hz, 3H) 4.91 (q, J = 6.69 Hz, 1H) 6.98 (d, J = 8.78 Hz, 2H) 7.61–7.80 (m, 4H) 7.98 (d, J = 8.28 Hz, 2H) 13.00 (br. s., 1H).
Synthesis of methyl 6-((1-methoxy-1-oxopropan-2-yl)oxy)-2-naphthoate (6a and 6b). To a solution of methyl 6-hydroxy-2-naphthoate (5, 4.0 g, MW = 202.21, 0.020 mol), (S)-methyl lactate or (R)-methyl lactate (2, 2.2 g, MW = 104.11, 0.021 mol) and triphenylphosphine (5.8 g, MW = 262.29, 0.022 mol) in dry 50 mL THF, diethyl diazocarboxylate (3.8 g, MW = 174.15, 0.022 mol) solution in 10 mL THF was dropwise added over 10 min at 0 °C. After stirring for 2 h at the same temperature, the reaction mixture was concentrated. Then, the residue was purified by flash column chromatography on silica gel-eluting with 20% ethyl acetate in petroleum ether to give 6a or 6b (4.3 g, MW = 288.30, 0.015 mol, 75%) as a white solid.
Synthesis of 6-(1-carboxyethoxy)-2-naphthoic acid (7a and 7b). Methyl 6-((1-methoxy-1-oxopropan-2-yl)oxy)-2-naphthoate (6a or 6b, 5.8 g, MW = 288.30, 0.020 mol), 10 mL methanol, 40 mL water, and solid sodium hydroxide (1.2 g, 0.030 mol) were added to a 100 mL round-bottomed flask containing a stirring bar. The reaction mixture was stirred and heated at 50 °C for 10 h, then the result solution was acidified to pH 1–2 with concentrated aqueous HCl in an ice bath. The precipitated solid was separated by filtration to give pure compound 7a or 7b (4.2 g, MW = 260.25, 0.016 mol, 80%) as a white solid: 1H NMR (400 MHz, DMSO-d6), δ (ppm): 1.58 (d, J = 6.78 Hz, 3H) 5.06 (q, J = 6.69 Hz, 1H) 7.21–7.31 (m, 2H) 7.77–7.88 (m, 1H) 7.88–7.96 (m, 1H) 8.03 (d, J = 8.78 Hz, 1H) 8.52 (s, 1H).
Synthesis of [Zn((R)-cbca)]·H2O] (CCP-1). A mixture of (R)-H2cbca (28.6 mg, 0.1 mmol), Zn(NO3)2·6H2O (29.7 mg, 0.1 mmol), 8 mL acetonitrile and distilled 2 mL water was stirred for 1 h in air and then transferred and sealed in a Teflon reactor (20 mL), which was heated at 120 °C for 3 days, then cooled to room temperature at a rate of 10 °C h−1. Colorless crystals of CCP-1 were obtained in 57% yield (based on Zn(NO3)2·6H2O). Elemental analysis (%) calcd for CCP-1 (C16H14O6Zn) (367.64): C, 52.27; H, 3.84. Found: C, 52.11; H, 3.71. IR (KBr, cm−1): 3269.5 (m), 1573.3 (s), 1528.1 (s), 1466.6 (s), 1424.2 (s), 1336.9 (m), 1245.7 (s), 1205.2 (m), 1085.6 (m), 1042.5 (m), 882.4 (w), 838.1 (m), 781.1 (m), 713.7 (w).
Synthesis of [Zn((S)-cbca)·H2O] (CCP-2). The preparation of CCP-2 was same as that of CCP-1 except that (S)-H2cbca (28.6 mg, 0.1 mmol) was used instead of (R)-H2cbca. Colorless crystals of CCP-2 were obtained in 53% yield (based on Zn(NO3)2·6H2O). Elemental analysis (%) calcd for CCP-2 (C16H14O6Zn) (367.64): C, 52.27; H, 3.84. Found: C, 52.09; H, 3.69. IR (KBr, cm−1): 3278.4 (m), 1581.6 (s), 1534.5 (s), 1473.6 (s), 1433.4 (s), 1343.2 (m), 1254.1 (s), 1210.4 (m), 1091.9 (m), 1051.5 (m), 890.5 (w), 846.4 (m), 789.1 (m), 722.3 (w).
Synthesis of [Zn((R)-cna)·2H2O] (CCP-3). A mixture of (R)-H2cna (26.0 mg, 0.1 mmol), Zn(NO3)2·6H2O (29.7 mg, 0.1 mmol), 8 mL acetonitrile and 2 mL distilled water was stirred for 1 h in air and then transferred and sealed in a teflon reactor (20 mL), which was heated at 140 °C for 3 days, then cooled to room temperature at a rate of 10 °C h−1. Colorless crystals of CCP-3 were obtained in 59% yield (based on Zn(NO3)2·6H2O). Elemental analysis (%) calcd for CCP-3 (C14H14O7Zn) (359.62): C, 46.76; H, 3.92. Found: C, 46.51; H, 3.73. IR (KBr, cm−1): 3386.2 (s), 3199.1 (s), 1558.5 (s), 1475.6 (s), 1408.1 (s), 1326.1 (m), 1215.2 (s), 1145.7 (m), 1103.3 (m), 1039.6 (m), 960.6 (w), 887.3 (m), 802.4 (w).
Synthesis of [Zn((S)-cna)·2H2O] (CCP-4). The preparation of CCP-4 was the same as that of CCP-3 except that (S)-H2cna (26.0 mg, 0.1 mmol) was used instead of (R)-H2cna. Colorless crystals of CCP-4 were obtained in 53% yield (based on Zn(NO3)2·6H2O). Elemental analysis (%) calcd for CCP-4 (C14H14O7Zn) (359.62): C, 46.76; H, 3.92. Found: C, 46.64; H, 3.65. IR (KBr, cm−1): 3373.4 (s), 3190.8 (s), 1549.4 (s), 1486.1 (s), 1400.9 (s), 1334.6 (m), 1205.7 (s), 1132.2 (m), 1114.6 (m), 1043.5 (m), 957.9 (w), 891.1 (m), 808.3 (w).
X-ray crystallography. Single-crystal X-ray diffraction data for CCPs 1–4 were collected on a Rigaku R-AXIS RAPID imaging plate diffractometer with graphite-monochromated Mo Kα (λ = 0.71073 Å) at 291 K. Empirical absorption corrections based on equivalent reflections were applied. The structures of 1–4 were solved by direct methods and refined by full-matrix least-squares methods on F2 using SHELXS-97 crystallographic software package.8 The crystal parameters, data collection and refinement results for 1–4 are summarized in Table 1. Selected bond lengths and angles of 1–4 are listed in Table S1† and the H-bond lengths and angles are shown in Table S2 (see the ESI†).
Table 1 Crystal data and structure refinement for CCPs 1–4a,b
Crystal parameters |
CCP-1 |
CCP-2 |
CCP-3 |
CCP-4 |
R1 = (∑||Fo| − |Fc|)/∑|Fo|. wR2 = [∑w(Fo2 − Fc2)2/∑w(Fo2)2]1/2. |
Empirical formula |
C16H14O6Zn |
C16H14O6Zn |
C14H14O7Zn |
C14H14O7Zn |
Formula weight |
367.64 |
367.64 |
359.62 |
359.62 |
Crystal system |
Monoclinic |
Monoclinic |
Monoclinic |
Monoclinic |
Space group |
P21 |
P21 |
P21 |
P21 |
a (Å) |
5.7071(11) |
5.6961(11) |
6.0565(12) |
6.045(5) |
b (Å) |
7.8905(16) |
7.8959(16) |
7.3687(15) |
7.363(5) |
c (Å) |
15.868(3) |
15.870(3) |
15.825(3) |
15.846(5) |
α (°) |
90 |
90 |
90 |
90 |
β (°) |
97.78(3) |
97.98(3) |
96.60 |
96.636(5) |
γ (°) |
90 |
90 |
90 |
90 |
V (Å3) |
708.0(2) |
706.9(2) |
701.6(2) |
700.6(8) |
Z |
2 |
2 |
2 |
2 |
Dcaled (Mg cm−3) |
1.725 |
1.727 |
1.702 |
1.705 |
μ (mm−1) |
1.765 |
1.768 |
1.784 |
1.786 |
Collected/unique |
6910/3103 |
5257/2414 |
6577/2802 |
2471/2464 |
Rint |
0.0423 |
0.0265 |
0.0242 |
0.0176 |
GOF on F2 |
1.107 |
1.076 |
1.088 |
1.079 |
R (I > 2σ(I)) |
R1 = 0.0354 |
R1 = 0.0274 |
R1 = 0.0254 |
R1 = 0.0428 |
wR2 = 0.0915 |
wR2 = 0.0609 |
wR2 = 0.0567 |
wR2 = 0.1247 |
R (all data) |
R1 = 0.0419 |
R1 = 0.0302 |
R1 = 0.0274 |
R1 = 0.0445 |
wR2 = 0.1064 |
wR2 = 0.0627 |
wR2 = 0.0575 |
wR2 = 0.1271 |
Results and discussion
Crystal structure of [Zn((R)-cbca)·H2O] (CCP-1) and [Zn((S)-cbca)·H2O] (CCP-2)
The single-crystal X-ray crystallographic analysis reveals that CCP-1 and CCP-2 crystallized in monoclinic system with a chiral space group of P21. As shown in Fig. 1(a), the asymmetric unit consists of one Zn(II) ion, one (R)-cbca2− anion and one coordinated water molecule. The Zn(II) ion is five-coordinated by four carboxylate oxygen atoms from three different (R)-cbca2− anions and one coordinated water molecule. The geometry around the Zn(II) center can be described as a trigonal bipyramid. The Zn–O bond lengths are in the range of 1.963(3)–2.398(3) Å, the O–Zn–O bond angles vary from 58.44(11) to 138.45(12)°, which are all in the reasonable range. The two carboxylate groups of the ligand employ bidentate chelating and bridging coordination modes, respectively.
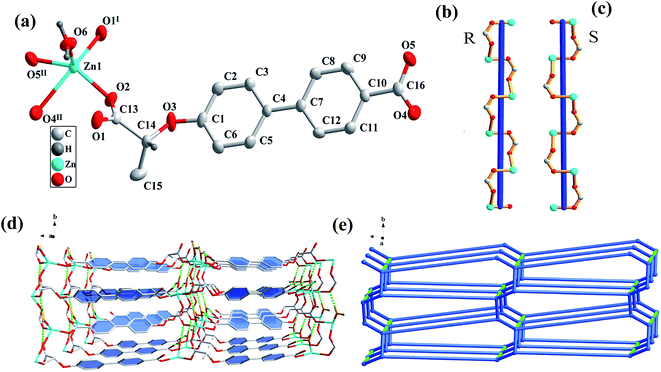 |
| Fig. 1 (a) Stick-ellipsoid representation of the asymmetric unit of CCP-1 (b) right-handed helical chain in CCP-1 (c) left-handed helical chain in CCP-2 (d) 2D grid network architecture made by an infinite right-handed helical chain running along b-axis. The layers linked together by hydrogen bonds to generate a 3D supramolecular architecture (e) schematic illustration of 3D 3,5-connected topological net with a point symbol of (63)(69·8), symmetry codes: (I) −x, y − 1/2, −z + 1; (II): x − 1, y, z − 1. | |
In CCP-1, an infinite right-handed helical chain along the b-axis is formed by a carboxylate group of the ligand bridging two Zn cations, comparatively, a homo left-handed helical chain is observed in CCP-2, the winding axis corresponds to the b axis and the pitch to the length of 7.896 Å. The adjacent chiral chains are further connected by the ligands to generate 2D wave-like layers (Fig. 2(b) and (c)). The 2D layers are further linked together by hydrogen bonds (O(6)–H(61)⋯O(4), O(6)–H(62)⋯O(2)) to eventually produce a 3D framework (Fig. 2(d)). The hydrogen bonding data are summarized in Table S1.† If the ligand is considered as a three-connected node, Zn(II) cation as a five-connected node and water molecule as a three-connected node, the framework of CCP-1 can be simplified as a 3,5-connected hms 3,5-conn topological structure with a point symbol of (63)(69·8) (Fig. 1(e)).
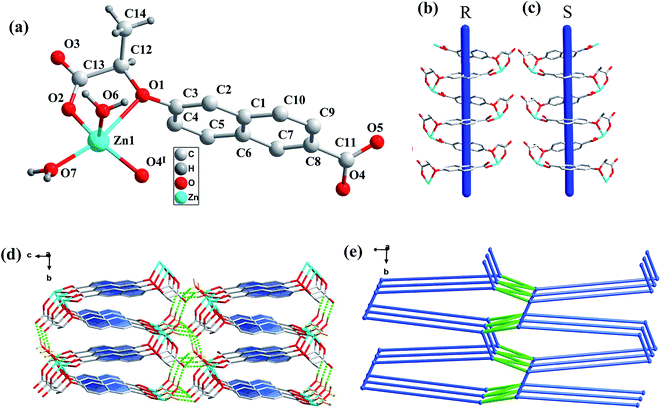 |
| Fig. 2 (a) Stick-ellipsoid representation of the asymmetric unit of CCP-3 (b) right-handed helical chain in CCP-3 (c) left-handed helical chain in CCP-4 (d) the right-handed helical chain generated by the connection of Zn(II) ion and the carboxylate group of the ligand and the three-dimensional supramolecular architecture constructed via hydrogen bonds interactions (e) schematic illustration of 3D 5-connected topological net with a point symbol of (44·66). Symmetry codes: (I) −x, y − 1/2, −z + 1. | |
Crystal structure of [Zn((R)-cna)·2H2O] (CCP-3) and [Zn((S)-cna)·2H2O] (CCP-4)
X-ray crystallographic analysis revealed that CCP-3 and CCP-4 crystallize in monoclinic system with chiral space group P21. The asymmetric unit in CCP-3 contains one Zn(II) ion, one (R)-cna2− anion and two coordinated water molecules. Each Zn(II) ion in CCP-3 was five-coordinated defined by two oxygen atoms from a carboxylate group and a alkoxyl group of one ligand, one oxygen atom from a carboxylate group of another ligand and two oxygen atoms from two coordinated water molecules. The Zn–O bond distances lie in the range of 1.9354(18)–2.4146(18) Å with the bond angles around each Zn(II) center ranging from 73.17(8) to 163.47(8)°, which are all in the normal range.
The outstanding structural feature of CCP-3 and CCP-4 is two types of 1D helical chains. Two Zn cations were connected by the (R)-cna2− anions to generate an infinite left-handed helical chain running along the b-axis in CCP-3, a comparative right-handed helical chain was formed in CCP-4, the winding axis corresponds to the b axis and the pitch to the length of 7.3687 Å (Fig. 2(b) and (c)). The 1D chiral helical chains are further linked together by hydrogen bonds (O(6)–H(61)⋯O(3), O(6)–H(62)⋯O(5), O(7)–H(71)⋯O(3), O(7)–H(72)⋯O(3)) to produce a three-dimensional (3D) framework (Fig. 2(d)). The hydrogen bonding data are summarized in Table S2.† From the view of topology, if the Zn(II) atom is considered as a 5-connected node, cna2− is viewed as a 5-connector, the structure of CCP-3 can be simplified as a 5-connected network with a point symbol of (44·66) (Fig. 2(e)).
The most obvious difference between the CCPs constructed by the two different ligands is the coordination modes of the ligands. In the two pairs of CCPs built by (R) and (S)-H2cbca, (R) and (S)-H2cna, the central Zn(II) atoms are all five-coordinated, however, different coordinating atoms of the ligands are involved. In CCP-1 and 2, for the ligands (R) or (S)-H2cbca with biphenyl group, only the carboxylate oxygen atoms besides the coordinating water molecules are involved in the coordination, while in CCP-3 and 4, for the ligands (R) or (S)-H2cna with naphthalene ring, an ether oxygen atom of the ligand also coordinates with Zn(II) atom. In CCP-1 and 2, the chiral carboxylate groups bridging coordinate with Zn(II) in μ2-η1:η1 and μ1-η1:η1 modes with a pitch to the length of 7.896 Å, while in CCP-3 and 4 the chiral carboxylate groups chelating coordinate with Zn(II) by carboxylate group and ether group with a pitch to the length of 7.369 Å, which causes the helical repeating units changed from {–Zn1–O2–C13–O3–}n in CCP-1 and 2 to {–Zn1–(cna)2–}n in CCP-3 and 4, leading to the eventual structural differences.
Thermal analysis and PXRD results
The powder X-ray diffraction of CCPs 1–4 were checked for the crystalline samples at room temperature. As shown in Fig. S5 and S6,† the peak positions of experimental and simulated PXRD patterns match well, which confirms their phase purity. The differences in intensity may be owed to the preferred orientation of the crystal samples.
To study the thermal stabilities of these CCPs, thermal gravimetric analysis (TGA) of CCPs 1–4 was performed (Fig. S7–S10†). TGA curves have been obtained under an air atmosphere for crystalline samples in the temperature range 48–800 °C with a heating rate of 10 °C min−1, showing that the two pairs of CCPs are all quite stable. CCP-1 and CCP-2 have two steps of weight loss. The first weight loss of 5.31% in the range of 50–235 °C is consistent with the removal of coordination water molecules (calcd, 4.89%). The second step of 235–595 °C can be attributed to the release of the ligand (found, 77.84%; calcd, 77.25%). Finally, the remaining weight is assigned to ZnO. Similarly, CCP-3 and CCP-4 also demonstrate two weight-loss processes. In CCP-3 and CCP-4, the weight loss in the range of 48–210 °C corresponds to the removal of two coordination water molecules (found, 10.06%; calcd, 10.31%). At 300–545 °C, the weight loss can be attributed to the decomposition of the ligand. Finally, the remaining residue could be ascribed to the formation of ZnO (found, 22.11%; calcd, 22.52%).
Circular dichroism spectra (CD) analysis
Considering that all the CCPs in this contribution crystallize in chiral space groups, their solid-state circular dichroism (CD) measurements have been performed to further demonstrate their homochirality (Fig. 3). As shown in Fig. 3(a), the CD spectrum for the bulk sample of CCP-1 exhibit a positive Cotton effect with peaks at 332 nm and a negative Cotton effect at 250 nm. The CD spectrum for the bulk sample of CCP-2 exhibits a negative Cotton effect with peak at 337 nm and a positive Cotton effect at 245 nm. The CD spectrum of CCP-3 exhibits a positive Cotton effect with peak at 320 nm and a negative Cotton effect at 260 nm. Meanwhile, a mirror image is observed for CCP-4 (as shown in Fig. 3(b)). In a word, the two pairs of CCPs have been confirmed to be enantiomers, respectively.
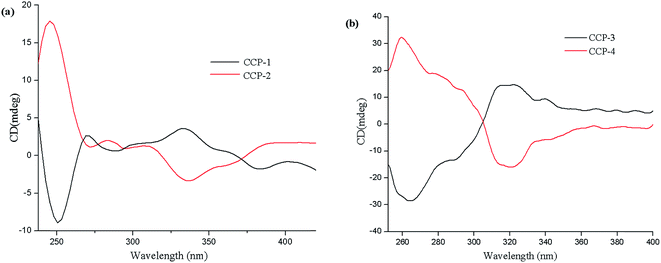 |
| Fig. 3 (a) Solid-state CD spectra of CCP-1 and CCP-2 (b) solid-state CD spectra of CCP-3 and CCP-4. | |
Second harmonic generation (SHG) efficiency
As Verbiest pointed out, supramolecular aggregates and chirality could significantly enhance the nonlinear optical properties.9 The nonlinear optical properties of Zn(II) based CCPs 1–4 are investigated since they are crystallized in the noncentrosymmetric chiral space groups as the colorless crystals. In general, the second-harmonic generation (SHG) efficiency can be measured according to the method proposed by Kurtz and Perry by using a powder technique. The SHG efficiency of a standard material, such as α-quartz, KH2PO4 (KDP), or urea are compared with the sample. The efficiency of KDP is 16× α-quartz, whereas that of urea is 400× α-quartz. The technologically important LiNbO3 is 600× α-quartz. In this work, the SHG efficiencies are compared with urea by using pure microcrystalline samples.
The preliminary experimental results indicated that the four CCPs have modest powder SHG efficiency up to 0.6, 0.6, 0.5, and 0.5 times, respectively, as much as that of urea. It was previously proposed that hydrogen bonding as a donor–acceptor system could significantly enhance the SHG response because hyperpolarizabilities strongly depend on the number molecules aggregated through H bonds.10 Most reported CCPs possessing SHG efficiency are built by Zn anions as metal centers. Compared with several reported Zn-based CCPs built by chiral ligands, as listed in Table 2, CCPs 1–4 show medium SHG activities. The SHG optical experimental result indicates that the four CCPs have the potential to be used as optical materials.11
Table 2 SHG activity comparison of Zn-based CCPs built from chiral ligands
CCPs |
Chemical formula |
Dimension |
Space group |
SHG |
Ref. |
1 |
[Zn((R)-cbca)·H2O] |
3D |
P21 |
0.6× urea |
This paper |
2 |
[Zn((S)-cbca)·H2O] |
3D |
P21 |
0.6× urea |
This paper |
3 |
[Zn((R)-cna)·2H2O] |
3D |
P21 |
0.5× urea |
This paper |
4 |
[Zn((S)-cna)·2H2O] |
3D |
P21 |
0.5× urea |
This paper |
5 |
[Zn(cpfa)] |
3D |
P212121 |
0.3× urea |
12 |
7 |
[Zn(cpca)(H2O)]2·(H2O) |
2D |
C2 |
0.5× urea |
13 |
8 |
Zn(SCMC)(H2O) |
2D |
P21 |
0.05× urea |
14 |
9 |
[Zn(bddp)·(H2O)4]2·H2O |
1D |
P21 |
0.6× urea |
15 |
Luminescent studies
Luminescence properties of metal–organic CCPs have been widely investigated, owing to their potential applications in chemical sensors, photochemistry, and electroluminescent displays.16 Therefore, in this work, the solid-state excitation (Fig. S13–S16†) and emission spectra for (R)-H2cbca, (R)-H2cna, CCP-1 and CCP-3 were studied in the solid state at room temperature (Fig. 4).
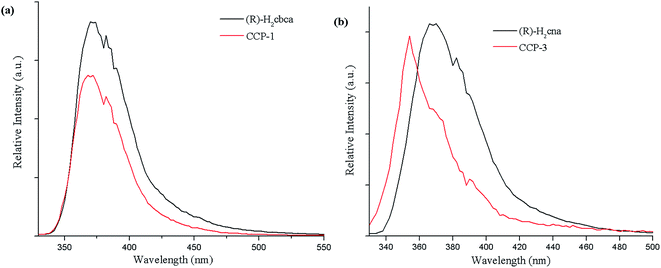 |
| Fig. 4 (a) Solid state emission spectra of (R)-H2cbca and CCP-1 (excited at 350 nm) (b) solid state emission spectra of (R)-H2cna and CCP-3 (excited at 345 nm). | |
The main emission peaks of (R)-H2cbca and (R)-H2cna are at 372 nm (λex = 350 nm) and 368 nm (λex = 345 nm). The emission peak of the free ligand is probably attributable to the π* → π or π* → n transitions as reported. The emission spectra for CCP-3 exhibit emission peak at 354 nm (λex = 345 nm). In comparison with (R)-H2cna, the blue shift of emission maxima in CCP-3 may be attributed to a charge transfer transition between ligand and metal center, Zn(II) ions with d10 electron configuration are rather stable and are difficultly oxidized or reduced. Thus, compared to the emission peak of H2cna ligand, the emissions of CCP-3 may be assigned to intraligand (n–π* or π–π*) emission. The similar emission energies between CCP-1 and the free ligands indicate that their most possible luminescent mechanism originates from the ligand centered emission. For CCPs 1–4 are highly thermally stable and insoluble in common solvents, they may be suitable as excellent candidates for the exploration of fluorescent materials.
Conclusion
In summary, two pairs of enantiomers (R) and (S)-H2cbca, (R) and (S)-H2cna with slight structural differences have been employed to construct two pairs of homochiral coordination polymers with Zn(II). The most obvious difference between the two pairs of CCPs lies in the different coordinating atoms of the ligands. Compared with CCP-1 and 2, an ether oxygen atom of the ligand in CCP-3 and 4 is also involved in the coordination besides the carboxylate oxygen atoms, leading to the differences of the pitch to the lengths of the helical repeating units. The SHG optical experimental results indicate that CCPs 1–4 have medium SHG activities which could be used as potential optical materials. The luminescent studies show that their most possible luminescent mechanism originates from the ligand centered emission.
Acknowledgements
This work is financially supported by the National Natural Science Foundation of China (no. 21371052 & 21272061), Scientific Research Fund of Heilongjiang Provincial Education Department (12531512).
References
-
(a) D. N. Dybtsev, A. L. Nuzhdin, H. Chun, K. P. Bryliakov, E. P. Talsi, V. P. Fedin and K. Kim, Angew. Chem., Int. Ed., 2006, 45, 916–920 CrossRef CAS PubMed;
(b) J.-Z. Gu, Y.-H. Cui, J. Wu and A. M. Kirillov, RSC Adv., 2015, 5, 78889–78901 RSC;
(c) S. R. Batten, N. R. Champness, X.-M. Chen, J. Garcia-Martinez, S. Kitagawa, L. Öhrström, M. O'Keeffe, M. P. Suh and J. Reedijk, CrystEngComm, 2012, 14, 3001 RSC;
(d) B. Chen, S. Xiang and G. Qian, Acc. Chem. Res., 2010, 43, 1115–1124 CrossRef CAS PubMed;
(e) Y. Cui, Y. Yue, G. Qian and B. Chen, Chem. Rev., 2012, 112, 1126–1162 CrossRef CAS PubMed.
-
(a) Y. Liu, W. Xuan and Y. Cui, Adv. Mater., 2010, 22, 4112–4135 CrossRef CAS PubMed;
(b) Q. Zhu, T. Sheng, R. Fu, C. Tan, S. Hu and X. Wu, Chem. Commun., 2010, 46, 9001–9003 RSC;
(c) W. J. Rieter, K. M. Pott, K. M. Taylor and W. Lin, J. Am. Chem. Soc., 2008, 130, 11584–11585 CrossRef CAS PubMed;
(d) D. Sun, Y. Ke, D. J. Collins, G. A. Lorigan and H.-C. Zhou, Inorg. Chem., 2007, 46, 2725–2734 CrossRef CAS PubMed;
(e) D. N. Dybtsev, M. P. Yutkin, E. V. Peresypkina, A. V. Virovets, C. Serre, G. Férey and V. P. Fedin, Inorg. Chem., 2007, 46, 6843–6845 CrossRef CAS PubMed.
-
(a) E. V. Anokhina, Y. B. Go, Y. Lee, T. Vogt and A. J. Jacobson, J. Am. Chem. Soc., 2006, 128, 9957–9962 CrossRef CAS PubMed;
(b) W. Xuan, M. Zhang, Y. Liu, Z. Chen and Y. Cui, J. Am. Chem. Soc., 2012, 134, 6904–6907 CrossRef CAS PubMed;
(c) R. Vaidhyanathan, D. Bradshaw, J. N. Rebilly, J. P. Barrio, J. A. Gould, N. G. Berry and M. J. Rosseinsky, Angew. Chem., Int. Ed., 2006, 45, 6495–6499 CrossRef CAS PubMed.
-
(a) L. Yu, X.-N. Hua, X.-J. Jiang, L. Qin, X.-Z. Yan, L.-H. Luo and L. Han, Cryst. Growth Des., 2015, 15, 687–694 CrossRef CAS;
(b) N. L. Strutt, H. Zhang and J. F. Stoddart, Chem. Commun., 2014, 50, 7455–7458 RSC;
(c) X.-L. Yang and C.-D. Wu, CrystEngComm, 2014, 16, 4907 RSC;
(d) H.-Y. An, E.-B. Wang, D.-R. Xiao, Y.-G. Li, Z.-M. Su and L. Xu, Angew. Chem., 2006, 118, 918–922 CrossRef;
(e) Z. X. Xu, Y. X. Tan, H. R. Fu, J. Liu and J. Zhang, Inorg. Chem., 2014, 53, 12199–12204 CrossRef CAS PubMed.
-
(a) X. Xu, Y.-H. Yu, G.-F. Hou, X.-W. Li, C.-Y. Ren and D.-S. Ma, Polyhedron, 2016, 112, 61–66 CrossRef CAS;
(b) Y.-H. Yu, C.-Y. Ren, G.-F. Hou, H. Ye and J.-S. Gao, J. Coord. Chem., 2012, 65, 4137–4146 CrossRef CAS;
(c) Y.-H. Yu, H.-T. Ye, G.-F. Hou, C.-Y. Ren, J.-S. Gao and P.-F. Yan, Cryst. Growth Des., 2016, 16, 5669–5677 CrossRef CAS.
-
(a) Z.-X. Xu, Y. Xiao, Y. Kang, L. Zhang and J. Zhang, Cryst. Growth Des., 2015, 15, 4676–4686 CrossRef CAS;
(b) L.-H. Cao, Y.-L. Wei, Y. Yang, H. Xu, S.-Q. Zang, H.-W. Hou and T. C. W. Mak, Cryst. Growth Des., 2014, 14, 1827–1838 CrossRef CAS;
(c) Z.-X. Xu, L. Liu and J. Zhang, New J. Chem., 2016, 40, 1927–1929 RSC.
- S. K. Kurtz and T. T. Perry, J. Appl. Phys., 1968, 39, 3798–3813 CrossRef CAS.
- G. M. Sheldrick, Acta Crystallogr., Sect. A: Found. Crystallogr., 2008, 64, 112–122 CrossRef CAS PubMed.
- T. Verbiest, Science, 1998, 282, 913–915 CrossRef CAS PubMed.
- Y.-R. Xie, R.-G. Xiong, X. Xue, X.-T. Chen, Z. Xue and X.-Z. You, Inorg. Chem., 2002, 41, 3323–3326 CrossRef CAS PubMed.
-
(a) N. Chen, M.-X. Li, P. Yang, X. He, M. Shao and S.-R. Zhu, Cryst. Growth Des., 2013, 13, 2650–2660 CrossRef CAS;
(b) C. Wang, T. Zhang and W. Lin, Chem. Rev., 2012, 112, 1084–1104 CrossRef CAS PubMed;
(c) X. Jing, C. He, D. Dong, L. Yang and C. Duan, Angew. Chem., Int. Ed., 2012, 51, 10127–10131 CrossRef CAS PubMed;
(d) F. Song, C. Wang, J. M. Falkowski, L. Ma and W. Lin, J. Am. Chem. Soc., 2010, 132, 15390–15398 CrossRef CAS PubMed;
(e) Y. Q. Sun, J. C. Zhong, L. Ding and Y. P. Chen, Dalton Trans., 2015, 44, 11852–11859 RSC.
- H.-T. Ye, C.-Y. Ren, G.-F. Hou, Y.-H. Yu, X. Xu, J.-S. Gao, P.-F. Yan and S.-W. Ng, Cryst. Growth Des., 2014, 14, 3309–3318 CAS.
- L.-L. Liang, S.-B. Ren, J. Zhang, Y.-Z. Li, H.-B. Du and X.-Z. You, Cryst. Growth Des., 2010, 10, 1307–1311 CAS.
- Y.-T. Wang, H.-H. Fan, H.-Z. Wang and X.-M. Chen, J. Mol. Struct., 2005, 740, 61–67 CrossRef CAS.
- H.-T. Zhang, Y.-Z. Li, T.-W. Wang, E. N. Nfor, H.-Q. Wang and X.-Z. You, Eur. J. Inorg. Chem., 2006, 2006, 3532–3536 CrossRef.
-
(a) M. Q. Wang, K. Li, J. T. Hou, M. Y. Wu, Z. Huang and X. Q. Yu, J. Org. Chem., 2012, 77, 8350–8354 CrossRef CAS PubMed;
(b) S. Li, J. Song, J. C. Ni, Z. N. Wang, X. Gao, Z. Shi, F. Y. Bai and Y. H. Xing, RSC Adv., 2016, 6, 36000–36010 RSC;
(c) Y. Peng, A. J. Zhang, M. Dong and Y. W. Wang, Chem. Commun., 2011, 47, 4505–4507 RSC;
(d) X.-L. Tang, X.-H. Peng, W. Dou, J. Mao, J.-R. Zheng, W.-W. Qin, W.-S. Liu, J. Chang and X.-J. Yao, Org. Lett., 2008, 10, 3653–3656 CrossRef CAS PubMed;
(e) X.-X. Wang, X.-Q. Wang, X.-Y. Niu and T.-P. Hu, CrystEngComm, 2016, 18, 7471–7477 RSC.
Footnote |
† Electronic supplementary information (ESI) available: Tables S1 and S2 and Fig. S1–S16. CCDC 1526162–1526165 for 1–4. For ESI and crystallographic data in CIF or other electronic format see DOI: 10.1039/c7ra00337d |
|
This journal is © The Royal Society of Chemistry 2017 |