DOI:
10.1039/C6RA27665B
(Paper)
RSC Adv., 2017,
7, 10391-10396
High-quality CsPbBr3 perovskite nanocrystals for quantum dot light-emitting diodes†
Received
2nd December 2016
, Accepted 26th December 2016
First published on 7th February 2017
Abstract
Metal halide perovskites, such as CsPbX3 (X = Cl, Br, and I), have gained extensive attention due to their increasing demand in optoelectronic applications such as solar cells and lighting-emitting devices. Herein, we report a versatile approach to synthesize high-quality CsPbBr3 perovskite nanocrystals (sized 5–15 nm) by ligand-assisted reprecipitation at room temperature. The monodispersed CsPbBr3 nanocube perovskites displayed relatively high photoluminescence quantum yields of 50–80%. By virtue of the quantum size effects, the bandgap energies were manipulated from blue to green spectral regions (410–530 nm). In addition, through compositional modulations of the anion exchange technique, the bright photoluminescence could be almost tuned over the entire visible spectral region (450–650 nm). Furthermore, the photoluminescence of the CsPbBr3 nanocrystals was characterized by narrow emission line widths of 15–50 nm and radiative lifetimes of 5–15 ns. Finally, by taking advantage of these outstanding merits, the CsPbBr3 perovskites were successfully utilized in the application of highly fluorescent patterning and color-purity light-emitting diodes.
Introduction
Halide perovskites have attracted significant interests in a variety of optoelectronic applications, including solar cells,1,2 light-emitting diodes (LEDs),3,4 photodetectors,5,6 and lasers,7,8 due to their high photoluminescence,9 excellent stability,10 and low-threshold lasing.11 Halide perovskites mainly contain two categories: organic–inorganic hybrid perovskites (APbX3, where A stands for the small molecular organic amine, such as CH3NH3+, and X represents a halide, such as Br, I, and Cl) and all-inorganic halide perovskites (CsPbX3). For the organic–inorganic hybrid perovskites,12–14 the facile and low-cost preparation process can be utilized to yield high-quality perovskite nanocrystals (NCs). Wong et al. reported a versatile strategy to prepare color-tunable CH3NH3PbBX3 perovskite nanorod arrays through an anion exchange technique at low temperature.15 Zhang et al. fabricated brightly luminescent colloidal CH3NH3PbX3 NCs with an absolute photoluminescence (PL) quantum yield (QY) of upto 70% at room temperature via a ligand-assisted reprecipitation (LARP) strategy.16 However, the relatively low PLQYs of organic–inorganic hybrid perovskites severely discourage their further development and potential application.
Recently, all-inorganic halide perovskites (such as CsPbX3, X = Cl, Br, and I), which can present relatively higher PLQYs compared with organic–inorganic hybrid perovskites,17,18 are emerging in perovskites family. In this respect, Protesescu et al. reported a facile colloidal synthesis of monodispersed CsPbX3 nanocubes at high temperature, which displayed a high PLQY of upto 90%, as well as a bright emission with a wide color gamut. The PL emission spectra of CsPbX3 perovskites could be tuned over the entire visible spectral region (410–700 nm).19 In addition, Song et al. synthesized high-quality CsPbX3 quantum dots (QDs) through a hot injection method and first developed the quantum dots-based QLEDs with sharp emissions.20 To further achieve the high-quality CsPbX3 perovskites at low temperature, Li et al. developed a supersaturated recrystallization method for the synthesis of CsPbX3 perovskites in the application of white LEDs. Excellent PL with high QY upto 95%, white LEDs with tunable color temperature, and a wide color gamut were obtained.21 It is because of the high PLQY that CsPbX3 perovskites are widely used in optoelectronic applications.
Herein, we report a high-quality colloidal CsPbBr3 perovskites NCs (sized 5–15 nm) synthesized at room temperature using a new precursor: cesium bromide (CsBr). The monodispersed CsPbBr3 nanocube perovskites exhibited relatively high PLQY (50–80%) and size-tunability of their bandgap energies that were manipulated from the blue to green spectral regions (410–530 nm). The PL of CsPbBr3 NCs also possessed narrow emission line widths of 15–50 nm and radiative lifetimes of 5–15 ns. In addition, via utilizing the anion exchange reaction technique, the bright PL could nearly be tuned over the entire visible spectral region (450–650 nm). Moreover, when considering cation exchange (MA+ and Cs+), the hybrid perovskites exhibited a high QY and narrow emission. Finally, we successfully designed highly luminescent patterning and color-purity LEDs using CsPbBr3 perovskite NCs, where the LED maintained the low turn-on voltages and narrow full-width at half maxima (FWHM) emission.
Experimental
Materials
Lead(II) bromide (PbBr2, 99%), methylamine (CH3NH2, 33 wt% in absolute ethanol), n-octylamine (99%), dodecylamine (98%), oleylamine (90%), hydrochloric acid (HCl, 37 wt%), hydrobromic acid (HBr, 48 wt%), hydriodic acid (HI, 57 wt%), and oleic acid (90%) were purchased from Aladdin Chemistry Co., Ltd. N,N-Dimethylformamide (DMF), cesium bromide (CsBr), toluene, cyclohexane, dichloromethane, and chloroform were purchased from Sinopharm Chemical Reagent Co., Ltd (Shanghai, China). All reagents were used without further purification.
Fabrication of the colloidal perovskite CsPbBr3 NCs
Colloidal perovskite CsPbBr3 NCs were fabricated by the LARP technique, which has been described as follows. In a typical synthesis, a mixture of 0.1 mmol CsBr and 0.2 mmol PbBr2 was dissolved in 5 mL of DMF via ultrasonication, and then 20 μL of n-octylamine and 0.5 mL of oleic acid were added to form a precursor solution. A 250 μL of precursor solution was added to 10 mL of toluene under vigorous stirring. A bright green solution could be immediately observed. After centrifugation at 12
000 rpm for 10 min to discard the precipitates, a bright blue-green colloidal solution was obtained. Other CsPbBr3 NCs were fabricated using different solvents, precursors, and ligands based on a similar LARP strategy.
Synthesis of CH3NH3X (MAX, X = Cl and I)
CH3NH3X (X = Cl and I) was prepared using an approach described in the literature.22 First, methylamine was dissolved in absolute ethanol under stirring at 0 °C for 2 h. Then, the corresponding acid was added to the abovementioned solution and it was left to react for 2 h at 0 °C under continuous stirring. After this, the solvent was removed by rotary evaporation with a pressure of 0.1 MPa at 50 °C. After rotary evaporation, the precipitate powders were collected and washed three times (diethylether) and dried in a vacuum oven (60 °C, 5 h).
Ion-exchange reactions
Ion-exchange with solid methylamine halide salts (MAX, X = Cl and I) were performed by adding different quantities (normally ranging from 1 to 2 mmol) of MAX solid salt to a rapidly stirred crude colloidal perovskite CsPbBr3 NCs solution (10 mL toluene).
Device fabrication
Solutions of ZnO nanocrystals were spin-coated on the ITO-coated glass substrates at 4000 rpm for 50 s and annealed in air at 200 °C. Perovskite solutions were spin-coated on the ZnO films to prepare a perovskite film (in the range of 125–150 nm). Then, a 25 nm thin layer of TFB was spin-coated using a m-xylene solution (10 mg mL−1) at 2500 rpm. Finally, the MoOx/Au electrodes were deposited using a thermal evaporation system.
Characterization
Ultraviolet-visible (UV-vis) absorption spectra were obtained using a Perkin-Elmer Lambda 950 spectrometer. Photoluminescence (PL) measurements were carried out using a Varian Cary Eclipse spectrophotometer. The photoluminescence quantum yield (PLQY) was estimated according to a standard procedure using appropriate dye molecules for the blue, green, and red spectral regions (coumarin 343, fluorescein, and rhodamine 6G). X-ray diffraction (XRD) was performed via a Rigaku Corporation D/max-rC rotating anode X-ray powder diffractometer using a copper target. Transmission electron microscopy (TEM) images were obtained using a Tecnai G2 F30 S-TWIN transmission electron microscope. X-ray photoelectron (XPS) spectra were acquired using an ES-CAIAB250 XPS system with an Al/Kα source, and the energy step size was set at 0.100 eV. Energy dispersive X-ray (EDX) analysis was performed using two scanning electron microscopes (Zeiss Gemini 1530 and Hitachi, S-4800). TCSPC data were performed using an Edinburgh FL 900 photo counting system. LED devices were measured using a Keithley 2400 source meter and a fiber integration sphere (FOIS-1) coupled with a QE-6500 spectrometer was used for room temperature measurements under ambient conditions.
Results and discussion
The colloidal CsPbBr3 NCs were synthesized via a simple and versatile LARP technique and a schematic of the detailed operation is shown in Fig. 1. A mixture of CsBr, PbBr2, oleic acid, and the assisted-ligand of n-octylamine was dissolved in N-dimethylformamide (DMF), ultrasonicated, and a uniform free-standing layered precursor solution was formed (Fig. 1a).23 Then, the precursor solution was gradually added into a vigorously stirred non-polar solvent (toluene, dichloromethane, etc.). After this, a blue-green colloidal solution was formed, implying an aggregation process of the precursor into nanoparticles.22 It was also revealed that the crystallization process was controlled by supersaturation, which was initially induced by the solubility change with solvent mixing.18 Finally, a high-quality colloidal solution was achieved, which is clearly seen from the optical image shown in Fig. 1b. Via utilizing the high PL properties of CsPbX3 perovskites, a “taiji” patterning (Fig. 1c) and LEDs (Fig. 1d) with saturated color-purity were obtained.
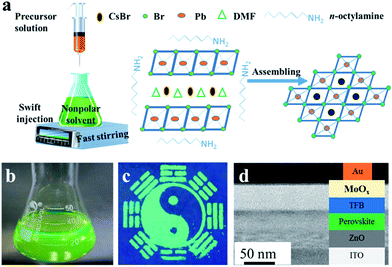 |
| Fig. 1 (a) Schematic of the prepared process for colloidal CsPbBr3 perovskite NCs, and the precursor solution and assembly into NCs. (b) The optical image of the colloidal nanocrystal solution. (c) The highly luminescent CsPbBr3 NCs-PMMA polymer patterning, and (d) LEDs using CsPbBr3 NCs. | |
The morphology, phase structure, and composition features are shown in Fig. 2. TEM image of the CsPbBr3 NCs is illustrated in Fig. 2a, which displays the cube shape and an average diameter of 7.45 nm with a size deviation of ±0.5 nm (Fig. 2b). The phase structure of the as-synthesized samples was further characterized by HRTEM and XRD patterns. It was observed that the lattice fringes of the NCs were 0.55 nm (Fig. 2c), corresponding to the (100) crystal faces.19 From the XRD patterns (Fig. 2d), the (100), (110), and (200) peaks demonstrated the cubic crystal structure of CsPbBr3 NCs.9,24 Fig. 2e shows the molecular structural model of CsPbBr3 NCs, which clarified that the ideal Cs
:
Pb
:
Br atomic ratio was close to 1
:
1
:
3 for the perovskite NCs. The detailed EDX result, as shown in Fig. 2f, reveals that the atomic ratio of the NCs was 1
:
1
:
3.2 (see Table S1†), which was close to the theoretical value. The elemental analysis was carried out via XPS (Fig. S1a†). As described in Fig. S1b–d,† the XPS showed the Cs 3d peaks at 726 eV and 740 eV, Pb 4f peaks at 141 eV and 146 eV, and the fitted two peaks of Br 3d at 69 eV and 71 eV.
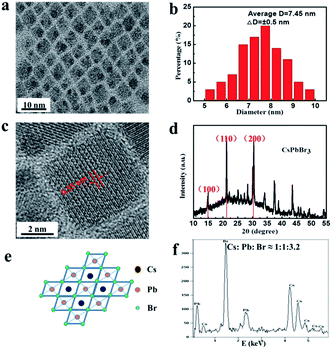 |
| Fig. 2 (a) TEM image of the colloidal CsPbBr3 NCs. (b) Size distribution analysis for the sample. (c) High-resolution TEM image of the colloidal CsPbBr3 NCs. (d) XRD patterns of the colloidal CsPbBr3 NCs. (e) Molecular structure model, and (f) EDX spectrum of the colloidal CsPbBr3 NCs. | |
The optical properties of the colloidal CsPbBr3 perovskite NCs were further investigated utilizing UV-vis absorption and PL spectroscopies. As described in Fig. 3a, the colloidal CsPbBr3 NCs present the absorption (red) and emission (black) spectra. A band edge at about 500 nm could be clearly observed in the UV-vis absorption spectrum. The CsPbBr3 NCs displayed a sharp emission peak position centered at 518 nm with a narrow FWHM value of only 18 nm from the PL spectrum, suggesting superior color saturation of the NCs (see the inset of Fig. 3a). The PLQY of the colloidal CsPbBr3 NCs was estimated to be 50–80%, which could be compared to the coated chalcogenide NCs.25,26 We further investigated the time-resolved PL decay of the CsPbBr3 NCs (Fig. 3b). The PL decay curve was fitted to the biexponential decay functions, and the radiative lifetimes were in the range of 5–20 ns.
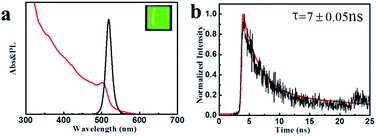 |
| Fig. 3 (a) UV-vis absorption and PL emission spectra (inset: image (λex = 365 nm) CsPbX3 NCs). (b) Time-resolved PL decay and fitting curve for the colloidal CsPbBr3 NCs sample. | |
The LARP method is a versatile technique to fabricate NCs via a solvent mixing approach and it is also a useful strategy to manipulate the size and morphology of the NCs via introducing capped ligands on the nanoparticles surface. It was reported that long-chain ligands could control the NCs size and the solvent polarity had a role in restraining the aggregation effects. Hence, we comprehensively investigated the solvent polarity, long-chain ligands (alkylamine with long chains), and the amount of ligands and precursor to affect the fluorescence properties (Fig. S2†). As illustrated in Fig. S2a,† PL showed a red shift effect with the increasing solvent polarity. Additionally, the red shift effect was obviously seen by increasing the alkyl chains of the capping ligand, as shown in Fig. S2b.† Furthermore, with the increasing amount of precursor, the red shift effect of PL was seriously decreased (Fig. S2c†). However, the blue shift effect of the PL intensity was increased after increasing the amount of ligand (Fig. S2d†). Thus, the added solvent polarity, ligand, and the amount of ligands and precursor could significantly influence the PL properties.
To further tune the wide spectra of the NCs PL, we adopted ion-exchange to realize the color of NCs by mixing the as-prepared CsPbBr3 NCs and MAX (X = Cl and I). We simultaneously investigated the effects of anion-exchange (halide source) and cation-exchange (MA+ and Cs+). The size and shape characteristics of the CsPbX3 NCs by ion-exchange manipulation can be clearly seen in Fig. 4. It is superior to those directly synthesized with mixed-halides CsPbX3 at low temperature, which obtained either bulk or poorly polydispersed non-luminescent crystallites. It was also suggested that our ion-exchanged samples could even be comparable to the directly synthesized mixed-halides CsPbX3 sample at high temperature. The molar ratios between MA+ ions and Cs+ ions were further determined by EDX measurements (Table S2†). Therefore, we considered that the reaction between MAX and CsPbBr3 in a non-stoichiometric ratio resulted in the formation of (Cs)x(MA)1−xPb(Cl/Br)3 or (Cs)x(MA)1−xPb(Br/I)3 intermediate complex.
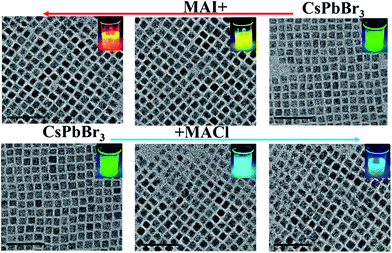 |
| Fig. 4 TEM images of the CsPbBr3 NCs after treatment with various quantities of MAI (up) and MACl (down). The insets show the evolution of emission colors (under a UV lamp, λex = 365 nm) from crude CsPbBr3 NCs to composited perovskite NCs. Scale bars correspond to 50 nm. | |
The bright PL of perovskite NCs (Fig. S3a†) could be tuned over almost the entire visible spectral region (450–650 nm). As observed from the PL spectra, as shown in Fig. 5a and b, the resulting composition of the ion-exchanged NCs were Stokes-shifted with respect to the optical absorption spectra and the NCs possessed a narrow FWHM of 10–40 nm, as previously reported.19 The PLQY of the samples generally dropped from the starting value of 82% after mixing the as-prepared CsPbBr3 NCs with either MACl or MAI (Fig. S3b†). Time-resolved photoluminescence decays of the resulting composition indicated the radiative lifetimes in the range of 1–20 ns. In general, the lifetimes of the resulting composition were increased with an increase of I-ions and decreased with an increase of Cl-ions (Fig. 5c).
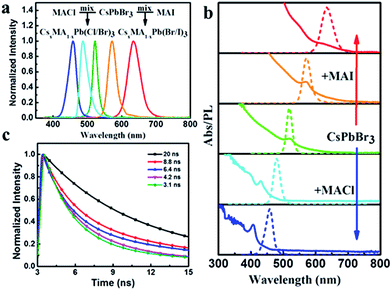 |
| Fig. 5 (a) An overview of the PL spectra of the samples obtained by mixing CsPbBr3 NCs with either MACl or MAI NCs in various ratios (λex = 365 nm for all). (b) Evolution of the optical absorption (solid lines) and PL (dashed lines) spectra of CsPbBr3 NCs with the increasing quantities of MAX (X = Cl and I), added as ion-exchange sources. (c) Evolution of the time-resolved PL decay spectra of CsPbBr3 NCs and mixing with either MACl or MAI NCs at various ratios. | |
It is well-known that the high speed of anion-exchange in the perovskite NCs is rooted in the ionic conductivity of halides.27 However, after using MAX (X = Cl and I), the cation (MA+) could be also engaged in ion-exchange reactions and raised a further complication, resulting in the replacement of Cs+ (1.81 Å) ions with the larger MA+ (2.70 Å) ions. According to the Fourier transform infrared spectroscopy (FTIR) analysis, we could obviously see the peaks intensity significantly strengthened at 2800 and 2900 cm−1, corresponding to stretching vibration of N–H (Fig. S4†).28 It was revealed that Cs+ ions replaced MA+ ions. To further demonstrate the ion-replacement, XRD was performed (Fig. S5†). From the lattice parameters change of the XRD patterns, we could infer the replacement of Cs+ ions with MA+ ions. However, the ion-exchange reactions almost had no influence on the shape and cubic structure of the starting CsPbBr3 sample under low temperature of the ion-exchange reaction.
Light-emission applications29 generally demand compatibility of the NC-emitters with matrix materials. To demonstrate this miscibility, the CsPbBr3 NCs were embedded into poly-(methylmethacrylate) (PMMA) forming well dispersed composites where the composites yielded excellent optical clarity and bright emission. Concomitantly, the versatile fluorescent patterns were further achieved by silk screen printing, which is a widely used and effective technique.30 As shown in Fig. 6a, a mixture of PMMA and CsPbBr3 NCs acting as printing ink was cast onto a mask (160 meshes screen), and the ink was infiltrated through the patterned mask onto the substrate by scraping the ink. Fig. 6b–d show the designed patterns of “NJTU” and “taiji”. The pattern was invisible under daylight (Fig. 6c), whereas it was remarkably visible under UV light (Fig. 6d). It was revealed that the patterns possessed both “vis-invisible” and “UV-visible” properties, which has potential application in the anti-counterfeit and labelling fields.
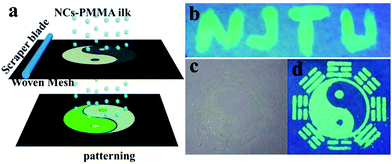 |
| Fig. 6 (a) The schematic of patterning by screen printing. (b), (d) Images (λex = 365 nm) and (c) (under daylight) of the patterning obtained using the mixture of CsPbBr3 NC-PMMA. | |
To design the practical application of the as-synthesized CsPbBr3 perovskite in optoelectronic devices, bright green electroluminescence (EL) LEDs were fabricated. The LED device consisted of multiple layers of ITO/ZnO/CsPbBr3/TFB/MoOx/Au (Fig. 7a): indium tin oxide (ITO) was coated on the glass substrate, 40 nm ZnO NCs were employed as electron-transporting and hole-blocking layers, the 25 nm poly(9,9-dioctyl-fluorene-co-N-(4-butylphenyl)diphenylamine) (TFB) served as the hole-transporting and electron-blocking interlayers, 10 nm molybdenum trioxide, and 100 nm gold (Au) electrode acted as a hole selective contact. Fig. 7b shows the EL spectra of the device under a bias of 4 V. The EL emission peak at 510 nm with a narrow FWHM of 20 nm and the shape of the EL spectrum did not change, indicating that the carriers were well confined within the perovskite layers. Fig. 7c presents the current density and luminescence versus driving voltage. The device displayed a turn on voltage of 3 V at room temperature. The current density and luminance rapidly increased after turning on the voltage, suggesting a very low series resistance and efficient operation of the LED device. The CsPbBr3 perovskite LEDs device presented a peak external quantum efficiency (EQE) at around 0.06% at ∼4 V (Fig. 7d), which is comparable to the previously reported studies.4,31
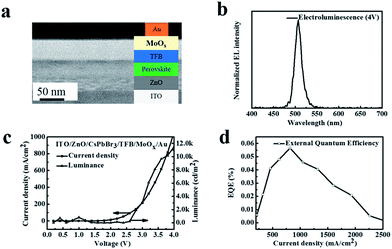 |
| Fig. 7 (a) Device structure of the CsPbBr3 perovskite LEDs. Schematic and the cross-sectional TEM image of the device: ITO/ZnO/perovskite/TFB/MoOx/Au. (b) Normalized EL spectrum of the CsPbBr3 perovskite thin film-based LEDs at the applied voltage of 4 V. (c) Current density and luminance for the CsPbBr3 perovskite LED measured at room temperature. (d) The external quantum efficiency (EQE) versus current density curves of this LED. | |
Conclusions
In summary, we synthesized highly luminescent colloidal CsPbBr3 NCs with high QY (QY = 50–80%) and highly bright blue and green emission (410–530 nm) using a simple and versatile LARP technique. Additionally, the post synthetic chemical transformations (ion-exchange, (MAX, X = Cl, I)) approach was utilized to achieve a wide variety of complex perovskite NCs, which displayed a visible spectral region of the PL spectrum (450–650 nm), and the PL is also affected by both the cation and anion exchange processes. Furthermore, we have constructed high color-purity LED using CsPbBr3 perovskite nanocrystals, which maintains the low turn-on voltages and narrow full-width at half maxima (FWHM) emission. We believe our results will guide the development of perovskites and open an avenue towards the design of high-performance optoelectronic devices.
Acknowledgements
This work was supported by National Natural Science Foundation of China (21474052) and Priority Academic Program Development of Jiangsu Higher Education Institutions (PAPD). This work was supported by the National Key Research and Development Program of China (project No. 2016YFB0401700).
Notes and references
- N. J. Jeon, J. H. Noh, Y. C. Kim, W. S. Yang, S. Ryu and S. I. Seok, Nat. Mater., 2014, 13, 897–903 CrossRef CAS PubMed.
- D. Li, P. Liao, X. Shai, W. Huang, S. Liu, H. Li, Y. Shen and M. Wang, RSC Adv., 2016, 6, 89356–89366 RSC.
- Z. K. Tan, R. S. Moghaddam, M. L. Lai, P. Docampo, R. Higler, F. Deschler, M. Price, A. Sadhanala, L. M. Pazos, D. Credgington, F. Hanusch, T. Bein, H. J. Snaith and R. H. Friend, Nat. Nanotechnol., 2014, 9, 687–692 CrossRef CAS PubMed.
- Y. Ling, Z. Yuan, Y. Tian, X. Wang, J. C. Wang, Y. Xin, K. Hanson, B. Ma and H. Gao, Adv. Mater., 2016, 28, 305–311 CrossRef CAS PubMed.
- L. Dou, Y. M. Yang, J. You, Z. Hong, W. H. Chang, G. Li and Y. Yang, Nat. Commun., 2014, 5, 5404 CrossRef CAS PubMed.
- L. Su, Z. X. Zhao, H. Y. Li, J. Yuan, Z. L. Wang, G. Z. Cao and G. Zhu, ACS Nano, 2015, 9, 11310–11316 CrossRef CAS PubMed.
- G. Xing, N. Mathews, S. S. Lim, N. Yantara, X. Liu, D. Sabba, M. Gratzel, S. Mhaisalkar and T. C. Sum, Nat. Mater., 2014, 13, 476–480 CrossRef CAS PubMed.
- Y. Fu, H. Zhu, C. C. Stoumpos, Q. Ding, J. Wang, M. G. Kanatzidis, X. Zhu and S. Jin, ACS Nano, 2016, 10, 7963–7972 CrossRef CAS PubMed.
- A. Swarnkar, R. Chulliyil, V. K. Ravi, M. Irfanullah, A. Chowdhury and A. Nag, Angew. Chem., Int. Ed., 2015, 54, 15424–15428 CrossRef CAS PubMed.
- S. Huang, Z. Li, L. Kong, N. Zhu, A. Shan and L. Li, J. Am. Chem. Soc., 2016, 138, 5749–5752 CrossRef CAS PubMed.
- Y. Wang, X. Li, X. Zhao, L. Xiao, H. Zeng and H. Sun, Nano Lett., 2016, 16, 448–453 CrossRef CAS PubMed.
- Y. S. Zhao, H. Fu, A. Peng, Y. Ma, D. Xiao and J. Yao, Adv. Mater., 2008, 20, 2859–2876 CrossRef CAS.
- F. Zhu, L. Men, Y. Guo, Q. Zhu, U. Bhattacharjee, P. M. Goodwin, J. W. Petrich, E. A. Smith and J. Vela, ACS Nano, 2015, 9, 2948–2959 CrossRef CAS PubMed.
- C. Lee, J. Hong, A. Stroppa, M.-H. Whangbo and J. H. Shim, RSC Adv., 2015, 5, 78701–78707 RSC.
- A. B. Wong, M. Lai, S. W. Eaton, Y. Yu, E. Lin, L. Dou, A. Fu and P. Yang, Nano Lett., 2015, 15, 5519–5524 CrossRef CAS PubMed.
- F. Zhang, H. Zhong, C. Chen, X.-g. Wu, X. Hu, H. Huang, J. Han, B. Zou and Y. Dong, ACS Nano, 2015, 9, 4533–4542 CrossRef CAS PubMed.
- J. Li, X. Yuan, P. Jing, J. Li, M. Wei, J. Hua, J. Zhao and L. Tian, RSC Adv., 2016, 6, 78311–78316 RSC.
- G. Nedelcu, L. Protesescu, S. Yakunin, M. I. Bodnarchuk, M. J. Grotevent and M. V. Kovalenko, Nano Lett., 2015, 15, 5635–5640 CrossRef CAS PubMed.
- L. Protesescu, S. Yakunin, M. I. Bodnarchuk, F. Krieg, R. Caputo, C. H. Hendon, R. X. Yang, A. Walsh and M. V. Kovalenko, Nano Lett., 2015, 15, 3692–3696 CrossRef CAS PubMed.
- J. Song, J. Li, X. Li, L. Xu, Y. Dong and H. Zeng, Adv. Mater., 2015, 27, 7162–7167 CrossRef CAS PubMed.
- X. Li, Y. Wu, S. Zhang, B. Cai, Y. Gu, J. Song and H. Zeng, Adv. Funct. Mater., 2016, 26, 2435–2445 CrossRef CAS.
- S. Sun, D. Yuan, Y. Xu, A. Wang and Z. Deng, ACS Nano, 2016, 10, 3648–3657 CrossRef CAS PubMed.
- Y. Niu, F. Zhang, Z. Bai, Y. Dong, J. Yang, R. Liu, B. Zou, J. Li and H. Zhong, Adv. Opt. Mater., 2015, 3, 112–119 CrossRef CAS.
- C. C. Stoumpos, C. D. Malliakas, J. A. Peters, Z. Liu, M. Sebastian, J. Im, T. C. Chasapis, A. C. Wibowo, D. Y. Chung, A. J. Freeman, B. W. Wessels and M. G. Kanatzidis, Cryst. Growth Des., 2013, 13, 2722–2727 CAS.
- P. Maity, T. Debnath and H. N. Ghosh, J. Phys. Chem. C, 2015, 119, 26202–26211 CAS.
- K. W. Song, R. Costi and V. Bulović, Adv. Mater., 2013, 25, 1420–1423 CrossRef CAS PubMed.
- J. Mizusaki, K. Arai and K. Fueki, Solid State Ionics, 1983, 11, 203–211 CrossRef CAS.
- S. Zhu, Q. Meng, L. Wang, J. Zhang, Y. Song, H. Jin, K. Zhang, H. Sun, H. Wang and B. Yang, Angew. Chem., Int. Ed. Engl., 2013, 52, 3953–3957 CrossRef CAS PubMed.
- F. Meinardi, A. Colombo, K. A. Velizhanin, R. Simonutti, M. Lorenzon, L. Beverina, R. Viswanatha, V. I. Klimov and S. Brovelli, Nat. Photonics, 2014, 8, 392–399 CrossRef CAS.
- S.-S. Liu, C.-F. Wang, C.-X. Li, J. Wang, L.-H. Mao and S. Chen, J. Phys. Chem. C, 2014, 2, 6477 CAS.
- N. Yantara, S. Bhaumik, F. Yan, D. Sabba, H. A. Dewi, N. Mathews, P. P. Boix, H. V. Demir and S. Mhaisalkar, J. Phys. Chem. Lett., 2015, 6, 4360–4364 CrossRef CAS PubMed.
Footnotes |
† Electronic supplementary information (ESI) available. See DOI: 10.1039/c6ra27665b |
‡ Contributed equally to this work. |
|
This journal is © The Royal Society of Chemistry 2017 |