DOI:
10.1039/C7QM00319F
(Research Article)
Mater. Chem. Front., 2017,
1, 2261-2264
New method for the synthesis of a highly-conjugated acene material and its application in Perovskite solar cells†
Received
13th July 2017
, Accepted 31st July 2017
First published on 1st August 2017
Abstract
Kita-type coupling and Scholl-type oxidative cyclodehydrogenation to afford acene from binaphthalene and mesitylene is as an effective alternative to the reported approaches. Acene may enable the broader application of small organic molecules in electronic and optical devices. Therefore, this novel synthetic method would promote the development of acene chemistry.
Introduction
Polycyclic aromatic hydrocarbons (PAHs), which have large π-areas, have received significant attention in the past two decades. They have potential applications as active components in field-effect transistors,1 photovoltaic devices,2 light-emitting diodes,3 and magnetic devices.10 The pioneering work on PAHs was reported by Scholl,4 Clar,5 and Zander.6 Compared with the traditional synthetic methods that use harsh conditions such as high temperature and pressure, methods that use mild conditions and provide better regioselectivity and higher yields have been developed in recent years. Since Müllen et al. synthesized p-HBC7 in 1995, several more complex PAHs, even graphene nanoribbons (GNRs) up to 12 nm in length,9 with different shapes and sizes have been reported.8 In the past few years, Diels–Alder [4+2] cycloaddition10 and Suzuki cross-coupling11 have been the well-established methods to construct polyphenylene derivatives. However, the challenge of finding a practical procedure applicable to PAHs has received significant attention.12 In 1912, Scholl and Seer achieved the formation of biaryls from arenes in the presence of stoichiometric FeCl3.13 Kita and co-workers found that the promotion of direct C–C coupling could be achieved by a combination of BF3·Et2O with [bis(trifluoroacetoxy)iodo] benzene (PIFA).14 Ar–Ar coupling between naphthalenes and polyalkybenzenes was achieved at the 1 position of naphthalene. Linear tetraarene with a binaphthalene core was prepared through direct oxidative Kita-type coupling between naphthalene and substituted benzene.15 Then, in the latter mode, the tetraenes were converted into a series of linear octiarenes.16 Oligonaphthalenes could be synthesized through a remarkably direct one-step via a regioselective intermolecular four/six-component assembly, and they could serve as precursors to long aromatic systems.17
Herein, we report the rapid transformation of oligonaphthalenes to nanographene-like structures, which have different conjugation lengths. Compared with the traditional synthetic methods, such as bromination and Suzuki coupling, used to synthesize acene,18 this synthetic route is shorter since it has only two steps. This research could be applied in the synthesis of nanographene materials. Moreover, acene is applied in perovskite solar cells, which exhibit excellent conductivity properties.
Results and discussion
Synthesis
The molecular structures of acene with an extended π-conjugation are shown in Scheme 1. Direct dehydrogenative coupling between the linear ter- and quaternaphthalenes and mesitylene was achieved under Kita conditions using the hypervalent PIFA/BF3·Et2O reagent to access 4,4′′-dimesityl-1,1′:4′,1′′-ternaphthalene (1a) and 4,4′′′-dimesityl-1,1′:4′,1′′:4′′,1′′′-quaternaphthalene (2a). Subsequently, oligonaphthalenes are used as the precursors for Scholl-type oxidative cyclodehydrogenation, in which FeCl3 is used as both a Lewis acid and an oxidant. The π-conjugation extended compounds 1 and 2 were obtained in 53% and 65% yield, respectively. They were characterized via1H and 13C nuclear magnetic resonance (NMR) spectroscopy and high-resolution mass spectrometry (MS).
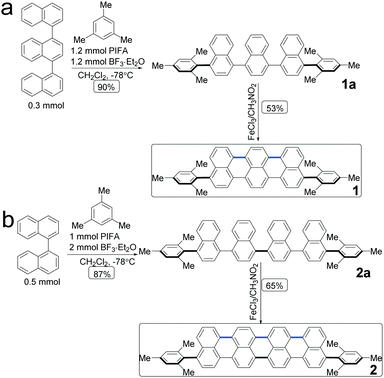 |
| Scheme 1 Synthetic route to acene: 1,8-dimesityltribenzo[de,kl,rst]pentaphene (1) and 1,10-dimesityldibenzo[kl,rst]benzo[10,5]anthra[9,1,2-cde]pentaphene (2). | |
Photophysical and electrochemical properties of 2
We obtained the ultraviolet-visible absorption spectra and fluorescence spectra of compound 2 in a CH2Cl2 solution. Fig. 1a shows the absorption and fluorescence spectra of compound 2 in CH2Cl2 (1 × 10−5 M). Both the absorption and emission spectra show well-resolved vibrational structures. Absorption bands appear in the range of 300–400 nm and 400–600 nm. The three peaks in the range of 400–600 nm are attributed to the 0 → 0, 0 → 1 and 0 → 2 vibration absorptions. The bands at around 287 nm are associated with the π–π* transition of the mesitylene unit. The three bands in the visible region are assigned to the π–π* transition in the naphthalene core. The fluorescence spectrum of compound 2 was obtained in solution, in which the maximum emission band was located at 604 nm upon excitation at 508 nm.
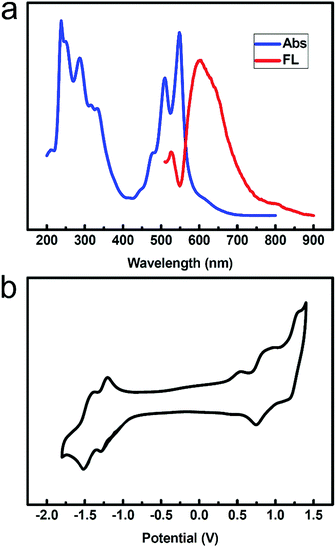 |
| Fig. 1 (a) Absorption (blue) and emission (red) spectra of compound 2 in CH2Cl2, and (b) CV trace of compound 2 in CH2Cl2 (0.1 mM) at room temperature. Scan rate: 100 mV s−1; working electrode: glassy carbon; reference electrode: Ag wire; and electrolyte: TBAPF6. | |
Cyclic voltammetry (CV) was used to examine the electrochemical behaviour of compound 2 in CH2Cl2 containing 0.1 M TBAPF6 as the supporting electrolyte (Fig. 1b). The cyclic voltammogram was remarkable, displaying three oxidation potentials at +0.28 V, +0.69 V, and +1.13 V, all as fully reversible waves (versus Fc/Fc+). Moreover, the compound exhibited two reduction potentials at −1.36V and −1.56 V.
We performed DFT calculations at the B3LYP/6-31G(d,P) level to examine the electronic structures of compound 2 (Fig. 2). The calculated frontier orbitals indicate different components. The HOMO (4.37 eV) and LUMO (2.53 eV) orbitals of compound 2 are mainly localized in the central oligonaphthalene unit. We observed nodal planes perpendicular to the molecular skeleton in the HOMOs and LUMOs with the electron densities distributed symmetrically on the two sides of the nodal plane. The time-depended DFT calculations suggest that the low-energy band could be assigned to a symmetry-allowed HOMO–LUMO transition.
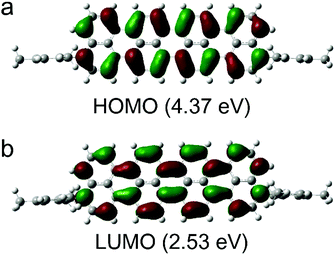 |
| Fig. 2 Frontier orbitals analysis of compound 2 calculated at the B3LYP/6-31G(d,p) level. | |
Application in perovskite solar cells
The device structure of the PHJ perovskite solar cells in this study was ITO/P3CT-K/Pervoskite/PC61BM/ZnO/Al,19 as shown in Fig. 3a. The resultant current density (J)–voltage (V) characteristics based on this device structure under AM 1.5G conditions (100 mW cm−2) are displayed in Fig. 3b, and the relevant parameters are summarized in Table 1 (reference devices based on PC61BM have also been fabricated and measured). The control device based on a pure PC61BM interlayer exhibited a Jsc of 22.54 mA cm−2, an open-circuit voltage (Voc) of 0.987 V, and a fill factor (FF) of 0.678 with a corresponding PCE of 15.07%. However, the device obtained using PC61BM:compound 2 as an electron transport layer displayed a Jsc of 25.2 mA cm−2, Voc of 1.032 V, and FF of 0.656, yielding a PCE of 17.07%. A remarkable higher PCE was observed for the PC61BM:compound 2-based devices with a 13% enhancement as compared to that based on pure PC61BM. Furthermore, both enhanced Jsc and FF were observed for the PC61BM:compound 2-based device. The enhanced device performances indicate an improvement in film conductivity and interfacial coverage based on compound 2 doping, which resulted in the high PCE of the devices and data repeatability. The enhancement of Jsc was obviously due to the improved transport networks by the delocalized π-system of the acene material.
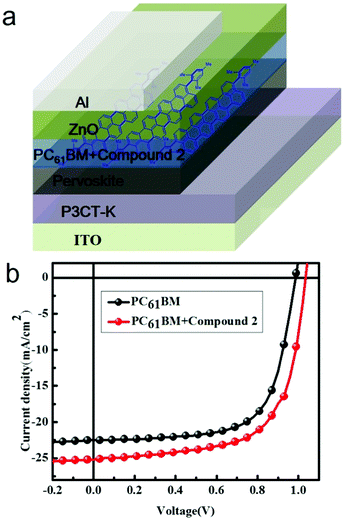 |
| Fig. 3 (a) Device architecture of the perovskite solar cell and (b) J–V characteristic curves of pure PC61BM and PC61BM:compound 2 based perovskite solar cells under AM 1.5G 100 mW cm−2 simulated solar light. | |
Table 1 Parameters of the optimized devices based on different electron transport layers
ETL |
V
oc (V) |
J
sc (mA cm−2) |
FF |
PCE (%) |
R at Voc |
Pure PC61BM |
0.987 |
22.54 |
67.8 |
15.07 |
5.6 |
PC61BM:compound 2 |
1.032 |
25.20 |
65.6 |
17.07 |
4.2 |
Conclusion
In conclusion, we have presented a facile and efficient approach based on Kita-type coupling and Scholl-type oxidative cyclodehydrogenation to afford acene from 1,1′-binaphthalene/1,1′:4′,1′′-ternaphthalene and mesitylene, which is an effective alternative to the reported approaches. The total synthesis is composed of 2 steps conducted under mild conditions and results in good to excellent yields. This method shortens the synthetic route for acene. We investigated the electronic structures of the synthesized compounds in the ground state using various experimental techniques and DFT calculations. Moreover, compound 2 as a dopant in the PC61BM ETL layer was successfully applied in perovskite solar cells for the first time. The high efficiency of 17.07% was demonstrated for the device based on the PC61BM:compound 2 ETL. Furthermore, these compounds may enable the broader applications of small organic molecules in high-performance electronic and optical devices. Thus, we believe that this method will promote the development of acene chemistry.
Experimental
4,4′′-Dimesityl-1,1′:4′′,1′′-ternaphthalene (1a) and 4,4′′′-dimesityl-1,1′:4′,1′′:4′′,1′′′-quaternaphthalene (2a) were synthesized according to literature reports.15,16
General procedure for the synthesis of 1 and 2
To a stirred solution of oligonaphthalenes (0.1 mmol) in dichloromethane (50 mL), a solution of iron(III) chloride (1 mmol) in nitromethane (10 mL) was added dropwise. A nitrogen stream was bubbled through the mixture throughout the course of the reaction. After stirring for 2 h at 40 °C, the reaction was quenched with methanol (10 mL). The resulting mixture was poured into water and extracted with DCM. The extract was purified by column chromatography to afford the corresponding compounds 1 and 2.
Compound 1.
Brown powder; yield: 53%; DCM/petroleum ether = 1/5, Rf = 0.21. 1H NMR (500 MHz, CDCl2CDCl2, 373.2 K) δ ppm 8.83 (d, J = 5.0 Hz, 1H), 8.61–8.57 (m, 1H), 8.47 (d, J = 10.0 Hz, 1H), 8.38 (d, J = 5.0 Hz, 1H), 8.08 (d, J = 10.0 Hz, 1H), 7.98 (s, 1H), 7.68 (t, J = 10.0 Hz, 2H), 7.54–7.39 (m, 6H), 7.09 (d, J = 15.0 Hz, 4H), 2.45 (d, J = 10.0 Hz, 6H), 2.01 (d, J = 10.0 Hz, 12H). 13C NMR (125 MHz, CDCl2CDCl2, 373.2 K) δ ppm 140.76, 138.98, 137.09, 136.76, 136.56, 136.56, 133.24, 132.17, 131.53, 130.90, 130.15, 128.76, 128.06, 127.72, 127.70, 127.06, 126.85, 126.60, 126.44, 125.57, 125.13, 124.94, 124.40, 121.89, 121.81, 121.05, 120.48, 120.39, 74.00, 73.89, 73.78, 73.56, 29.39, 20.88, 20.10. HRMS (MALDI-TOF) m/z calcd for C48H36 [M]+ 612.2817, found: 612.2819.
Compound 2.
Purple powder; yield: 65%; DCM/petroleum ether = 1/2, Rf = 0.34. 1H NMR (500 MHz, CDCl2CDCl2, 373.2 K) δ ppm 8.71–8.36 (m, 6H), 8.24 (d, J = 10.0 Hz, 1H), 8.02–7.89 (m, 2H), 7.81 (t, J = 10.0 Hz, 2H), 7.66–7.38 (m, 7H), 7.11 (s, 4H), 2.49 (s, 6H), 2.07 (s, 12H). 13C NMR (125 MHz, CDCl2CDCl2, 373.2 K) δ ppm 137.58, 136.67, 132.78, 131.42, 130.97, 128.08, 126.92, 126.83, 125.35, 124.75, 124.51, 124.25, 122.45, 122.11, 121.71, 121.05, 74.00, 73.78, 73.56, 59.53, 31.01, 29.39, 20.89, 20.09, 20.04, 0.71. HRMS (MALDI-TOF) m/z calcd for C58H40 [M]+ 736.3130, found: 736.3128.
Sample characterization
1H and 13C NMR spectra were obtained using a Bruker AVANCE III 500 WB instrument at a constant temperature of 373.2 K. Chemical shifts are reported in parts per million from low-to-high field and referenced to TMS. Matrix-assisted laser desorption/ionization Fourier transform ion cyclotron resonance (MALDI-FT-ICR-MS) MS was performed using a Bruker Solarix 9.4T FT-ICR-MS mass spectrometer. The CV experiment was performed using an electrochemical analyser with the conventional three-electrode configuration: glassy carbon electrode as the working electrode and Ag/AgCl as the reference electrode. All experiments were performed in CH2Cl2 with 0.1 M of nBu4NPF6 as the supporting electrolyte and ferrocene/ferrocenium ion (Fc/Fc+) as the inter-reference.
Theoretical calculations
DFT calculations were performed using the Gaussian 09 program.20 Geometry was optimized in the gas-phase using the B3LYP functional and 6-31g(d,p) basis set on all atoms.
Perovskite device fabrication
Presented in the ESI.†
Conflicts of interest
There are no conflicts to declare.
Acknowledgements
This work was supported by the National Key Research and Development Project of China (2016YFA0200104), the Key Program of Chinese Academy of Science (QYZDY-SSW-SLH015), the National Nature Science Foundation of China (21322301, 21672222), the NSFC-DFG joint fund (21261130581), and the Strategic Priority Research Program of the Chinese Academy of Sciences (XDA09020302, XDB12010300).
Notes and references
- L. B. Roberson, J. Kowalik, L. M. Tolbert, C. Kloc, R. Zeis, X. L. Chi, R. Fleming and C. Wilkins, J. Am. Chem. Soc., 2005, 127, 3069–3075 CrossRef CAS PubMed.
- D. Adam, P. Schuhmacher, J. Simmerer, L. Haussling, K. Siemensmeyer, K. H. Etzbach, H. Ringsdorf and D. Haarer, Nature, 1994, 371, 141–143 CrossRef CAS.
- V. de Halleux, J. P. Calbert, P. Brocorens, J. Cornil, J. P. Declercq, J. L. Bredas and Y. Geerts, Adv. Funct. Mater., 2004, 14, 649–659 CrossRef CAS.
- R. Scholl, C. Seer and R. Weitzenbock, Ber. Dtsch. Chem. Ges., 1910, 43, 2202–2209 CrossRef CAS.
-
E. Clar, Polycyclic Hydrocarbon, Academic Press, New York, 1964, vol. 1, p. 2 Search PubMed.
-
M. Zander, Handbook of Polycyclic Aromatic Hydrocarbons, Dekker, New York, 1983 Search PubMed.
- A. Stabel, P. Herwig, K. Mullen and J. P. Rabe, Angew. Chem., Int. Ed. Engl., 1995, 34, 1609 CrossRef CAS.
-
(a) L. Schmidt-Mende, A. Fechtenkotter, K. Müllen, E. Moons, R. H. Friend and J. D. MacKenzie, Science, 2001, 293, 1119–1122 CrossRef CAS PubMed;
(b) M. Kastler, W. Pisula, D. Wasserfallen, T. Pakula and K. Müllen, J. Am. Chem. Soc., 2005, 127, 4286–4296 CrossRef CAS PubMed;
(c) L. F. Doessel, V. Kamm, I. A. Howard, F. Laquai, W. Pisula, X. Feng, C. Li, M. Takase, T. Kudernac, S. De Feyter and K. Müllen, J. Am. Chem. Soc., 2012, 134, 5876–5886 CrossRef CAS PubMed;
(d) M. Yin, J. Shen, W. Pisula, M. Liang, L. Zhi and K. Müellen, J. Am. Chem. Soc., 2009, 131, 14618–14619 CrossRef CAS PubMed;
(e) M. Kastler, J. Schmidt, W. Pisula, D. Sebastiani and K. Müellen, J. Am. Chem. Soc., 2006, 128, 9526–9534 CrossRef CAS PubMed.
- X. Yang, X. Dou, A. Rouhanipour, L. Zhi, H. J. Raeder and K. Müellen, J. Am. Chem. Soc., 2008, 130, 4216–4217 CrossRef CAS PubMed.
- X. F. Shen, D. M. Ho and R. A. Pascal, Org. Lett., 2003, 5, 369–371 CrossRef CAS PubMed.
- D. Meng, H. Fu, C. Xiao, X. Meng, T. Winands, W. Ma, W. Wei, B. Fan, L. Huo, N. L. Doltsinis, Y. Li, Y. Sun and Z. Wang, J. Am. Chem. Soc., 2016, 138, 10184–10190 CrossRef CAS PubMed.
-
(a) J. Li and Q. Zhang, Synlett, 2013, 686–696 Search PubMed;
(b) J. Li and Q. Zhang, ACS Appl. Mater. Interfaces, 2015, 7, 28049–28062 CrossRef CAS PubMed;
(c) Z. Wang, P. Gu, G. Liu, H. Yao, Y. Wu, Y. Li, G. Rakesh, J. Zhu, H. Fu and Q. Zhang, Chem. Commun., 2017, 53, 7772–7775 RSC;
(d) P. Gu, Z. Wang, G. Liu, H. Yao, Z. Wang, Y. Li, J. Zhu, S. Li and Q. Zhang, Chem. Mater., 2017, 29, 4172–4175 CrossRef CAS;
(e) P. Gu, Z. Wang, F. Xiao, Z. Liu, R. Song, Q. Xu, J. Lu, B. Liu and Q. Zhang, Mater. Chem. Front., 2017, 1, 495–498 RSC.
-
(a) R. Scholl and C. Seer, Liebigs Ann. Chem., 1912, 394, 111 CrossRef . For a review, see: ;
(b) P. Kovacic and M. B. Jones, Chem. Rev., 1987, 87, 357–379 CrossRef CAS . Also see: ;
(c) B. T. King, J. Kroulik, C. R. Robertson, P. Rempala, C. L. Hilton, J. D. Korinek and L. M. Gortari, J. Org. Chem., 2007, 72, 2279–2288 CrossRef CAS PubMed.
-
(a) T. Dohi, M. Ito, K. Morimoto, M. Wata and Y. Kita, Angew. Chem., Int. Ed., 2008, 47, 1301–1304 CrossRef CAS PubMed;
(b) Y. Kita, K. Morimoto, M. Ito, C. Ogawa, A. Goto and T. Dohi, J. Am. Chem. Soc., 2009, 131, 1668–1669 CrossRef CAS PubMed;
(c) A. Kar, N. Mangu, H. M. Kaiser, M. Beller and M. K. Tse, Chem. Commun., 2008, 386–388 RSC.
- E. Faggi, R. M. Sebastian, R. Pleixats, A. Vallribera, A. Shafir, A. Rodriguez-Gimeno and C. Ramirez de Arellano, J. Am. Chem. Soc., 2010, 132, 17980–17982 CrossRef CAS PubMed.
- W. Guo, E. Faggi, R. M. Sebastian, A. Vallribera, R. Pleixats and A. Shafir, J. Org. Chem., 2013, 78, 8169–8175 CrossRef CAS PubMed.
- N. G. Pschirer, C. Kohl, T. Nolde, J. Q. Qu and K. Müllen, Angew. Chem., Int. Ed., 2006, 45, 1401–1404 CrossRef CAS PubMed.
- Q. Zhang, H. Peng, G. Zhang, Q. Lu, J. Chang, Y. Dong, X. Shi and J. Wei, J. Am. Chem. Soc., 2014, 136, 5057–5064 CrossRef CAS PubMed.
-
(a) C. Kuang, G. Tang, T. Jiu, H. Yang, H. Liu, B. Li, W. Luo, X. Li, W. Zhang, F. Lu, J. Fang and Y. Li, Nano Lett., 2015, 15, 2756–2762 CrossRef CAS PubMed;
(b) N. Wang, K. Zhao, T. Ding, W. Liu, A. S. Ahmed, Z. Wang, M. Tian, X. W. Sun and Q. Zhang, Adv. Energy Mater., 2017, 1700522 CrossRef;
(c) P. Gu, N. Wang, C. Wang, Y. Zhou, G. Long, M. Tian, W. Chen, X. W. Sun, M. G. Kanatzidis and Q. Zhang, J. Mater. Chem. A, 2017, 5, 7339–7344 RSC.
-
M. J. Frisch, Gaussian 09, Revision E.01, Gaussian, Inc., Wallingford, CT, 2013 Search PubMed.
Footnote |
† Electronic supplementary information (ESI) available. See DOI: 10.1039/c7qm00319f |
|
This journal is © the Partner Organisations 2017 |