DOI:
10.1039/C7QM00170C
(Research Article)
Mater. Chem. Front., 2017,
1, 2027-2030
Self-adaptive water vapor permeability and its hydrogen bonding switches of bio-inspired polymer thin films†
Received
18th April 2017
, Accepted 8th June 2017
First published on 15th June 2017
Abstract
Developing materials with self-adaptive water vapor permeability is fueled with industrial demands. Polymers have enabled such a behavior with thermal-responsive water vapor permeability reported in the literature, but little work is reported on the moisture-sensitive water vapor permeability which is even more important for many applications, such as clothing comfort, since sweating can be enormous when actively exercising in the cold. In this paper, a polymer inspired by a leaf guard-cell and designed by introducing supramolecular hydrogen-bonding switches and moisture-sensitive self-adaptive water vapor permeability has been achieved. It is suggested that hydrogen bonding can become a moisture-responsive switch when its bonding energy is smaller than the energy between the water molecule and the switch itself, but much bigger than that of water.
Self-Adaptive Water Vapor Permeability (SAWVP) is desired in many areas, ranging from garments,1 wound dressing,2 gas separation,3 food packaging4 and green houses to buildings.5 Taking garments as an example, our human body is covered by a porous skin that perspires all the time since it has more than 60% water under a core temperature of 37 °C. It is essential for a garment to be able to transport such water vapor promptly to the outside from the skin, known as water vapor permeability (WVP), to maintain physical and psychological health. Our garments are normally porous, thus performing such a function. However, under circumstances such as wet weather, in order to provide protection against rain and wind, it is difficult to achieve sufficient WVP when a fabric is coated or laminated with an extra polymer. Different approaches have been used, like applying microfibers, tuning fabric structures,6 finishing,7 coating8 and laminating9 polymer films to a fabric for an improved WVP. Since different working conditions, such as temperature and exercising intensity, lead to different levels of perspiration, a new trend has been fueled by technological needs in the recent decade,6,8,10–12 that is, textiles with a SAWVP for enhanced comfort and physiological safety are capable of supporting the abrupt microclimate changes of wearers in extreme environmental conditions. Such a SAWVP could be realized with polymers possessing stimulus-responsive functions. Block shape memory polyurethanes were reported to have SAWVP due to a thermally-responsive free volume change in their soft-segments (SS).9,13 Below its transition temperature (Ttrans), the mobility of SS is low and the polymer acts as an insulator for maintaining warmth and moistening skin along with a low WVP. When the polymer is used above the Ttrans, the SS becomes ‘active’ and allows the transportation of more water vapor, thus keeping the body dry and comfortable.14–17 However, compared with heat, the moisture level is equally or even more important in governing the comfort of clothing, as people can sweat both in high and low temperatures when undertaking a high degree of workload.9,18,19 Thus, textiles with thermal-sensitive WVP have limitations as they cannot deal with a sweating situation under a cold climate (say, skating) since a relative humidity (RH) of only between 30–60% is recommended as a comfortable zone (neither too dry nor too wet).20 Thus, the ideal textile should allow a low WVP at a low RH while become highly permeable to water vapor at a high RH as shown in Fig. 1(a). Therefore, developing materials with a moisture-responsive SAWVP has a strong industrial demand.
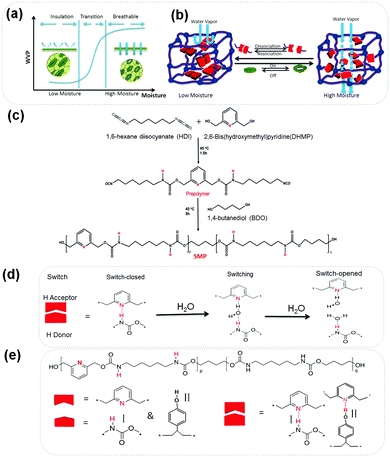 |
| Fig. 1 Design of the self-adaptive water vapor permeability of SMP films. (a) SAWVP requirement of garments and moisture management of guard-cells in a plant leaf. (b) Bio-inspired SMP film as a moisture-responsive material. (c) The synthesis procedures and chemical structures of the raw materials. (d) Moisture-responsive switching of HBs in a SMP film. (e) Molecular structure and switches of SAWVP films. | |
Learning from our previous work, the hydrogen bonding (HB), acting as a switch among proton donors (PD) and acceptors (PA) could be reversibly broken and rebuilt by moisture.21,22 Inspired by plant leaves, whose guard-cell could close and open-up according to the external environment to sustain a water balance (Fig. 1(a)),23 we have designed a supra-molecular polymer (SMP) with a moisture-responsive function, as shown in Fig. 1(b). It is hypothesized that the introduction of moisture-sensitive HBs as a switch can lead the SMP to have a SAWVP, as in Fig. 1(a), namely, at a high RH, the sweat should be transported away to avoid water condensation in cold weather or heat accumulation in hot conditions, while at a low RH, a garment needs insulation for warmth retention and/or skin moistening. In the middle levels of RH, there should be a switching or rapid transition to ensure physiological and psychological comfort under a critical environment.
The description of the synthesis procedure in Fig. 1(c) can be found in the ESI.†Fig. 1(d) illustrates the evolution of the HB switch with water in the SMP. To achieve a self-adaptive water vapor permeability, we chose two HB switches, switch I is between 2,6-dihydroxymethyl pyridine (DHMP) and hexamethylene diisocyanate (HDI), switch II is from DHMP and poly(4-vinyl phenol) (PVP).24 Moisture-responsive shape memory polyurethanes containing a fraction of pyridine moieties as PAs were synthesized from DHMP, HDI and 1,4-butanediol (BDO) (Fig. 1(e)). DHMP was chosen as the PA because of its stronger Lewis base nature compared to the of N,N-bis(2-hydroxylethyl) isonicotinamide (BINA),21 where the carboxyl group at the p-position may compromise the electron density of the nitrogen on the pyridine ring. The HDI-BDO segment was employed to develop netpoints and tune the mechanical properties. Thanks to the reaction between NCO from HDI and the OH group from DHMP, the resident hydrogen on the amino group could serve as a PD to make switch I, or by mixing additional PVP to form switch II. Then the WVP was studied systematically in terms of its relationships with film thickness, RH, and HB energy in shape memory polyurethanes, both experimentally and theoretically using simulation.25–27
Fourier transform infrared spectroscopy (FTIR) can reflect changes of HB behavior.21,28 With the increase in HB strength, the peak of the IR absorption will move to a lower frequency and vice versa. The full FTIR spectrum of the SMP with switch I is depicted in Fig. 2(a) at both low RH (switch-close) and high RH (switch-open) states. The detailed characteristics of the related peaks and their shifts are registered in Fig. 2(b)–(d) for this switch. An observable shift trend from a low wave number to a high wave number exists for peaks related to the pyridine ring under a high RH, indicating the breaking of the HB between DHMP and the amino group after the penetration of water vapor. Stable HBs of the pyridine ring with the N–H in the urethane group are formed (switch closed) at a low RH, thus a low WVP is allowed. Such HBs may be broken by the penetration of water vapor at a high RH, leading to an opening of the HBs and thus a higher WVP. The WVP vs. RH relationship was measured. In Fig. 2(e), the WVP increases with RH almost linearly for thick SMP films (around 0.1 mm). Even this is the correct tendency for moisture management; it is still not ideal because we wish to have a quick transition at a critical range of RH for clothing comfort. Then, the thickness of a film was reduced significantly to 0.001 mm, the switching effect of the WVP was boosted at around 65%. This means, when the RH is below 65%, the WVP increases very slowly, while when the RH is above 65%, the WVP of the films increases dramatically, indicating a successful SAWVP for moisture management. This may be caused by a shorter path for the water molecular transportation with thinner films. The dependence of WVP on the film thickness was further tested at 95% RH, Fig. 2(f), demonstrating that the ideal thickness should be below 25 μm for industrial applications. The repeatability and reversibility of the SAWVP was validated on the SMP with a film thickness of 0.015 mm for 3 cycles, Fig. 2(g), with almost the same switch RH at around 65%.
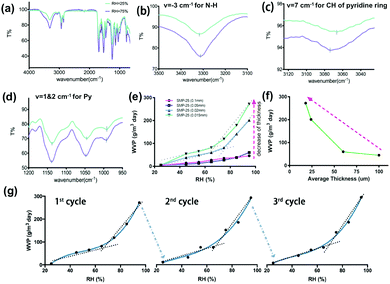 |
| Fig. 2 Self-adaptive WVP of moisture-responsive SMP films. (a–d), FTIR spectra of HB shifts in the SMP at switch-close (dry) and -open (wet) states: (a) total absorption of the SMP film, (b) N–H group, (c) CH of the pyridine ring, (d) Py group. (e) Relationship between WVP and RH of SMP films. (f) Relationship between WVP and film thickness. (g) Reversibility of SAWVP of the SMP thin films (0.012 mm). | |
The HB in switching was further simulated by inserting water molecules between PD and PA. In Fig. 3(a), the stable states of the intra-molecular bonds between PD, PA and water were calculated by quantum chemistry.29 The pyridine ring first formed a HB with a PD from shape memory polyurethanes at a dry state (switch-closed). At a very low RH, the closure of supra-molecular switches hinders the passing of water molecules, and thus leads to the very low WVP in Fig. 3(a1). With the increase of RH, water molecules participate in a competition of the HB switches, with one water molecule matching one HB, HBs hand-in-hand with the PD and PA lead to a PA⋯H2–O⋯PD structure, where the supra-molecular switch is still not yet open, leading to a relatively low, but preparing for a high WVP. This is the starting point of the switching or transition. When two water molecules were added into the hydrogen bonded system, these water molecules formed individual HBs with the PD and PA to create an open channel for water to pass through (switch-opened). Then, at a slightly higher RH, with fully opened supra-molecular switches, a large amount of water vapor can pass through the path easily, and thus a much higher WVP is reached. This mechanism of RH responsive WVP in the proposed SMP film is schematically shown in Fig. 3(b). Fig. 3(c) is a schematic illustration of the practical application of moisture management material in textile industries.
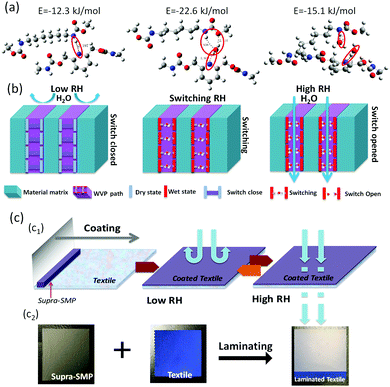 |
| Fig. 3 Mechanism of self-adaptive WVP in SMP thin films with a HB switch I. (a) Modeling of the hydrogen competition of a switch with water (a1, switch bond is closed due to a low RH with few water molecules, a2, switch bond is occupied by a water molecule at a critical RH and a3, switch opened due to breakage of the hydrogen bond by water). (b) Mechanism of moisture-sensitive WVP in the thin film (b1) vapor path is blocked due to the closure of the switch, b2 vapor path is occupied by water molecules and b3 vapor path is opened and water molecules can pass easily. (c) A Supra-SMP which can be (c1) coated or (c2) laminated to the fabric to serve the purpose of water resistance and provide reasonable water vapor permeability. | |
A strong HB can resemble a covalent bond with respect to the energy required to break the interaction, while the energy of a weak HB will be closer to a van der Waals force.30,31 This leads to complexity in deciding what HBs are suitable for smart materials as reversible switches in terms of bonding energy. To investigate this issue, more and stronger HBs were introduced, namely, external PDs from PVP were superimposed into the synthesized SMP by blending. It would be expected that more HBs would lead to a higher WVP, since PVP can easily form absorbent HBs24 in switch II. Surprisingly, the result is the opposite, that is, all the PVP blended SMP films have a lower WVP as shown in Fig. 4(a). With a smaller film thickness, the switching effect also disappeared, in Fig. 4(b), with the added switch II WVP. The result of the SMPU coated fabric in Fig. 4(c) indicates that it has an almost similar permeability compared with the SAWVP film. Fig. 4(d) shows the computed structure and bonding energy of relevant HB complexes. Our theoretical analyses revealed the order of the HB strength: HB-switch II > HB-HS > HB-DHMP-H2O > HB switch I > HB-PVP-H2O > HB-H2O. Thus, HB-switch II does not work because it is too strong for water to compete, and thus shows poor WVP. In contrast, switch I has a moderate HB strength, and thus the water can form competitive HBs with the two components of the switch, with a good SAWVP.
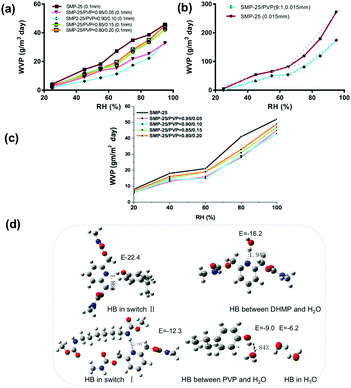 |
| Fig. 4 Hydrogen bonding energy and moisture switch. (a) WVP of SMP/PVP films (SMP mixed with PVP) and superimposed HBs. (b) WVP of thin SMP films with and without PVP superimposed HBs. (c) WVP result of SMPU coated fabric. (d) Bonding energy of HB complexes in the SMP films. (SMP-25: the content of hard-segment is 25%). | |
Conclusions
In summary, polymeric thin films with supra-molecular HB switches inspired by plant leaf guard-cells were fabricated. The HBs from DHMP-HDI (PA-PD) were found to have a moisture-responsiveness for SAWVP, particularly switching at around 65% RH. However, the HBs from DHMP-PVP did not show any benefit for an SAWVP, and thus cannot act as the switch. The mechanism of the moisture-responsive SAWVP for the former was illuminated by FTIR and quantum chemistry modeling. The bonding energy is used to judge whether a HB can be a water-responsive switch. Specifically, a HB switch responsive to water in smart materials should have a bonding energy smaller than that of the HB between water and the switch, but much larger than that of HBs in water. HBs formed between PVP and DHMP have a much larger bonding energy than that between PVP and water and even stronger than that in the hard segments. The thickness of the films plays a vital role in their responsiveness to humidity in SAWVP; it is considered that the too irregular water paths in thick films hinder vapor passage while the switching efficiency would be the highest with a single molecular layer in the film. The work presented here provides insights for the rational design of smart materials using HBs. The SMP with a HB switch formed with DHMP-HDI displayed a high potential for sportswear, wound dressing and food storage. Since H-bonded complexes are abundant in the universe, such as in the DNA double helix32 and beta-sheet crystals,33 the conclusion regarding the switchable HB may provide a new approach in the study of natural systems.
Acknowledgements
The authors of this paper would like to acknowledge the financial support from projects General Research Fund (GRF) (Project Number: 516111, 516212, 515813), Research Grants Council (RGC) of Hong Kong (Project Number: 500810), Development of Environmental Friendly and Self-Adaptive Breathable Polyurethane Film (GHX/001/16GD), and the Science and Technology Planning Project of Guangdong Province, China (Project Number: 2016A050503013).
Notes and references
- C. O'Brien, L. A. Blanchard, B. S. Cadarette, T. L. Endrusick, X. Xu, L. G. Berglund, M. N. Sawka and R. W. Hoyt, J. Occup. Environ. Hyg., 2011, 8, 588–599 CrossRef PubMed.
- J. S. Boateng, K. H. Matthews, H. N. Stevens and G. M. Eccleston, J. Pharm. Sci., 2008, 97, 2892–2923 CrossRef CAS PubMed.
- J. Li, Z. L. Su, H. J. Xu, X. D. Ma, J. Yin and X. S. Jiang, Macromolecules, 2015, 48, 2022–2029 CrossRef CAS.
- J. Gómez-Estaca, R. Gavara, R. Catalá and P. Hernández-Muñoz, Packag. Technol. Sci., 2016, 29, 203–224 CrossRef.
- S. Schiavoni, F. D'Alessandro, F. Bianchi and F. Asdrubali, Renewable Sustainable Energy Rev., 2016, 62, 988–1011 CrossRef.
- Y. Jhanji, D. Gupta and V. K. Kothari, J. Text. Inst., 2015, 106, 663–673 CrossRef CAS.
- M. B. Sampath, A. Aruputharaj, M. Senthilkumar and G. Nalankilli, J. Ind. Text., 2012, 42, 19–33 CrossRef.
- P. K. Lavric, M. M. C. G. Warmoeskerken and D. Jocic, Cellulose, 2012, 19, 257–271 CrossRef.
- G. R. Lomax, J. Mater. Chem., 2007, 17, 2775–2784 RSC.
- Y. Chen, Y. Liu, H. J. Fan, H. Li, B. Shi, H. Zhou and B. Y. Peng, J. Membr. Sci., 2007, 287, 192–197 CrossRef CAS.
- C. Y. Lin, K. H. Liao, C. F. Su, C. H. Kuo and K. H. Hsieh, J. Membr. Sci., 2007, 299, 91–96 CrossRef CAS.
- Y. Zhu, J. L. Hu, K. W. Yeung, K. F. Choi, Y. Q. Liu and H. M. Liem, J. Appl. Polym. Sci., 2007, 103, 545–556 CrossRef CAS.
- S. Mondal and J. L. Hu, Carbohydr. Polym., 2007, 67, 282–287 CrossRef CAS.
- X. M. Ding, J. L. Hu, X. M. Tao, C. P. Hu and G. Y. Wang, J. Appl. Polym. Sci., 2008, 107, 4061–4069 CrossRef CAS.
- S. Mondal and J. L. Hu, Polym. Eng. Sci., 2008, 48, 233–239 CAS.
- Y. Chen, R. Wang, J. A. Zhou, H. J. Fan and B. Shi, Polymer, 2011, 52, 1856–1867 CrossRef CAS.
- H. T. Zhuo, J. L. Hu and S. J. Chen, Mater. Lett., 2008, 62, 2074–2076 CrossRef.
- J. Hu, H. Meng, G. Li and S. I. Ibekwe, Smart Mater. Struct., 2012, 21, 053001 CrossRef.
- S. X. Wang, Y. Li, H. Tokura, J. Y. Hu, Y. X. Han, Y. L. Kwok and R. W. Au, Text. Res. J., 2007, 77, 968–980 CrossRef CAS.
- C. Zhang, J. Hu, X. Li, Y. Wu and J. Han, J. Phys. Chem. A, 2014, 118, 12241–12255 CrossRef CAS PubMed.
- S. Chen, J. Hu, C. W. Yuen and L. Chan, Polymer, 2009, 50, 4424–4428 CrossRef CAS.
- S. Chen, J. Hu, C.-W. M. Yuen and L. Chan, Polym. Int., 2010, 59, 529–538 CrossRef CAS.
- T. A. Mansfield, Environ. Pollut., 1998, 101, 1–11 CrossRef CAS PubMed.
- Y. He, B. Zhu and Y. Inoue, Prog. Polym. Sci., 2004, 29, 1021–1051 CrossRef CAS.
- C. Tang, E. M. Lennon, G. H. Fredrickson, E. J. Kramer and C. J. Hawker, Science, 2008, 322, 429–432 CrossRef CAS PubMed.
- X. Zhang, L. Wang, T. Jiang and J. Lin, J. Chem. Phys., 2013, 139, 184901 CrossRef PubMed.
- C. Tang, S.-M. Hur, B. C. Stahl, K. Sivanandan, M. Dimitriou, E. Pressly, G. H. Fredrickson, E. J. Kramer and C. J. Hawker, Macromolecules, 2010, 43, 2880–2889 CrossRef CAS.
- Y. Zhu, J. L. Hu and K. Yeung, Acta Biomater., 2009, 5, 3346–3357 CrossRef CAS PubMed.
-
M. J. Frisch, G. W. Trucks, H. B. Schlegel, G. E. Scuseria, M. A. Robb, J. R. Cheeseman, V. G. Zakrzewski, J. A. J. MonTgomery, R. E. Stratmann, J. C. Burant, S. Dapprich, J. M. Millam, A. D. Daniels, K. N. Kudin, M. C. Strain, O. Farkas, J. Tomasi, V. Barone, M. Cossi, R. Cammi, B. Mennucci, C. Pomelli, C. Adamo, S. Clifford, J. Ochterski, G. A. Petersson, P. Y. Ayala, Q. Cui, K. Morokuma, D. K. Malick, A. D. Rabuck, K. Raghavachari, J. B. Foresman, J. Cioslowski, J. V. Ortiz, B. B. Stefanov, G. Liu, A. Liashenko, P. Piskorz, I. Komaromi, R. Gomperts, R. L. Martin, D. J. Fox, T. Keith, M. A. Al-Laham, C. Y. Peng, A. Nanayakkara, C. Gonzalez, M. Challacombe, P. M. W. Gill, B. G. Johnson, W. Chen, M. W. Wong, J. L. Andres, M. Head-Gordon, E. S. Replogle and J. A. Pople, Gaussian 2003W, Gaussian Inc., Pittsburgh PA, 2003 Search PubMed.
-
D. B. Varshey, J. R. G. Sander, T. Friščić and L. R. MacGillivray, in Supramolecular chemistry: from molecules to nanomaterials, ed. P. A. Gale and J. W. Steed, Wiley, New York, 2012, vol. 1, pp. 9–24 Search PubMed.
-
M. Anthamatten, in Supramolecular Polymer Networks and Gels, ed. S. Seiffert, Springer International Publishing, Cham, 2015 DOI:10.1007/978-3-319-15404-6_2, pp. 47–99.
- S. R. Holbrook, C. Cheong, I. Tinoco and S.-H. Kim, Nature, 1991, 353, 579–581 CrossRef CAS PubMed.
- S. Keten, Z. Xu, B. Ihle and M. J. Buehler, Nat. Mater., 2010, 9, 359–367 CrossRef CAS PubMed.
Footnote |
† Electronic supplementary information (ESI) available. See DOI: 10.1039/c7qm00170c |
|
This journal is © the Partner Organisations 2017 |
Click here to see how this site uses Cookies. View our privacy policy here.