DOI:
10.1039/C6QM00135A
(Review Article)
Mater. Chem. Front., 2017,
1, 807-822
Recent progress and advances in redox-responsive polymers as controlled delivery nanoplatforms
Received
21st July 2016
, Accepted 16th November 2016
First published on 23rd December 2016
Abstract
Stimuli-responsive polymeric nanosystems that can respond to biological stimuli such as pH, temperature, glucose, enzymes or redox conditions have been extensively explored for different biomedical applications. Among these, redox conditions should be the most useful stimulus in biological systems, which rely on the significantly different redox states in the circulation/extracellular fluids and intracellular compartments. By incorporation of redox-responsive linkages such as disulfide and diselenide into polymers, different redox-responsive polymeric nanosystems can be fabricated. In this review article, a number of redox-responsive polymeric therapeutic nanosystems and their design principles are included. Recent advances in these redox-responsive polymeric therapeutic nanosystems for controlled cytoplasmic delivery of a number of bioactive molecules (e.g. drugs, biological proteins, plasmid DNA, siRNA) are also highlighted. This review will provide useful information for the design and biomedical applications of redox-responsive polymeric therapeutic nanosystems, which will attract great research interest from scientists in chemistry, materials, biology, medicine and interdisciplinary areas.
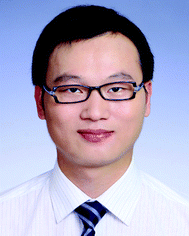
Xiaoyong Zhang
| Dr Xiaoyong Zhang was born in Jiangxi, China, in 1980. He received his BS (2004) from Wuhan University of Science and Technology and his PhD (2011) from Shanghai Institute of Applied Physics (SINAP), Chinese Academy of Sciences (CAS). After a Postdoc at the Department of Chemistry, Tsinghua University with Professor Yen Wei, he took a position at Nanchang University as an associate professor. He has published more than 160 papers with total citations over 4600 and an H index of 39. His research is focused on AIE based materials and their biomedical applications, mussel-inspired chemistry, and carbon nanomaterials. |
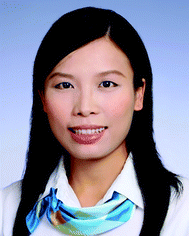
Meiying Liu
| Dr Meiying Liu was born in Jiangxi, China, in 1981. She received her BS (2004) from Medical College of Nanchang University and her PhD (2011) from Shanghai Institute of Micro-system and Information Technology (SIMIT), Chinese Academy of Sciences (CAS). After a Postdoc at the Institute of Chemistry, CAS with Professor Lei Jiang, she took a position at Nanchang University as an associate professor. She has published about 90 papers with total citations over 2300 and an H index of 30. Her research is focused on nanobiosensors, bio-inspired smart nanochannels, mussel-inspired chemistry, AIE based materials and their biomedical applications. |
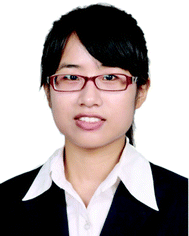
Ke Wang
| Dr Ke Wang received her PhD degree in Analytical Chemistry from the Institute of Chemistry, Chinese Academy of Sciences in 2014 under the supervision of Prof. Yi Chen. She is presently working as a postdoctoral fellow in the group of Prof. Yen Wei at Tsinghua University, China. Her research interests focus mainly on the design and synthesis of functional water-dispersible fluorescent nanoparticles and the exploration of their applications in sensing, imaging and therapy. |
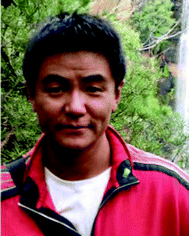
Lei Tao
| Dr Lei Tao received his Bachelor’s and Master’s degrees from the University of Science & Technology of China (USTC) in 1999 and 2002, respectively. He received his PhD degree in 2006 from the University of Warwick (Prof. David Haddleton), and then moved to UCLA and the University of New South Wales as a research fellow. In 2010, Dr Tao joined the Department of Chemistry, Tsinghua University as an associate professor. Dr Tao's research interests include new polymerization methodologies, self-healing hydrogels and well-defined polymers for bio-applications. Dr Tao has published more than 110 SCI papers and his H-index is 40. |
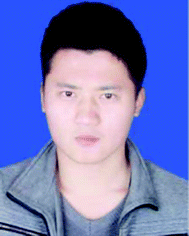
Qing Wan
| Qing Wan was born in Jiangxi, China in 1992. He received his BS (2014) in Chemistry from Shangrao Normal University. He is studying at the College of Chemistry of Nanchang University with Professor Xiaoyong Zhang. He has published about 40 papers with over 300 citations. His research interests mainly focus on mussel-inspired chemistry and AIE based materials and their biomedical applications. |
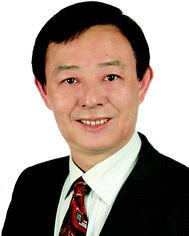
Yen Wei
| Dr Yen Wei is a Chair Professor of Chemistry and Director of the Tsinghua Center for Frontier Polymer Research at Tsinghua University in China. He received his undergraduate diploma (1979) and MS (1981) at Peking University, and his PhD at City University of New York (1986). After postdoctoral work at MIT, he joined Drexel University in 1987, where he became Full Professor in 1995. He has coauthored more than 790 articles with over 21 000 citations and an H-index of 73. He joined Tsinghua University in November 2009, and his current research focuses on polymers and nano-materials for bioscience, biomedicine and energy technology. |
1. Introduction
With recent advances in understanding of the molecular mechanism of disease and the controllable manipulation of materials at the nanoscale, nanotechnology holds tremendous promise for the detection, prevention and treatment of disease.1–4 Nanomaterials with size at the nanometer scale have exhibited many remarkable properties for biomedical applications.5–21 It has been demonstrated that nanocarriers with high specific surface area can be used to load a large amount of biologically active agents such as drugs, proteins, DNA, siRNA, antisense oligonucleotides etc. through noncovalent encapsulation and covalent conjugation.22–39 On the one hand, these nanocarriers can effectively protect biologically active agents from being degraded by enzymes and deliver them to target sites through active targeting or by the enhanced permeation and retention (EPR) effect.40–45 On the other hand, many other functional components such as radionuclides, fluorescent dyes, magnetic nanoparticles, polymers and targeting agents (e.g. folic acids, antibodies, peptides, aptamers) can also be integrated into these nanocarriers, and thus multifunctional nanocarriers can be fabricated.46–64
To date, a number of nanocarriers composed of organic polymers, inorganic nanomaterials and organic–inorganic hybrids have been developed.65–89 Among them, polymeric nanocarriers with stimuli responsive behavior have attracted the most research attention due to the good designability of polymers and their multifunctional potential, good performance and stimulus responsiveness.90–116 Various external and internal stimuli including temperature, ultrasonic, magnetism, light, electricity, pH, redox, glucose, and enzymes etc. have been used for controlled therapeutic agent delivery.51,94,117–156 Like many other stimuli such as pH, temperature, glucose and enzymes, redox conditions present a promising stimuli for controlled delivery applications owing to the significantly different redox states between extracellular and intracellular spaces.157–174 It is well-known that the glutathione (GSH) level in intracellular spaces is 100–1000-fold higher than that in blood circulation and extracellular milieu. Therefore, these polymeric therapeutic nanocarriers are stable in blood circulation and extracellular milieu, however, when they accumulate in targeted sites and are internalized by cells, these polymeric nanosystems will be disassembled and release their cargo.175–177 To date, a series of redox-responsive nanosystems including redox-responsive copolymers, nanocapsules, branched polymers, biodegradable polymers and redox-responsive poly(amino acid)s have been developed.178–181 The criterion for redox-responsive polymeric systems is that the polymers can respond to reduction or oxidation stimuli. Therefore, redox-responsive systems can typically be divided into reduction-responsive systems and oxidation-responsive systems. Herein, we will mainly focus on redox polymeric systems that can potentially respond to redox stimuli from biological systems. The basic principle for designing these redox-responsive polymeric nanosystems is the incorporation of redox-responsive units such as disulfide, diselenide or ditellurium bonds into these polymers.182–185 These bonds embedded in polymers will break when the polymers are internalized by cells. This will lead to the disassembly of the polymeric nanosystems in intracellular spaces, releasing the biologically active agents. Taking advantage of the redox-responsiveness, different redox-responsive therapeutic systems can be elegantly fabricated for the potential delivery of cargo on demand.
This review presents recent progress and advances in redox-responsive polymeric therapeutic nanosystems, with a special focus on the design and fabrication of various redox-responsive polymeric therapeutic systems that can respond to biological redox stimuli. The biomedical applications of these polymeric nanosystems for controlled delivery of drugs, proteins, plasmid DNA, siRNA etc. have also been included in this review article. Although many review articles have been published over the past few decades, novel redox-responsive linkages such as disulfide and diselenide have attracted limited attention. The fabrication strategies and types of redox-responsive polymeric nanosystems have not been well classified. We anticipate that this review will inspire novel ideas for designing new stimuli-responsive therapeutic polymeric nanosystems, and promote the further design and development of sophisticated polymeric therapeutic nanosystems.
2. Types of redox-responsive polymeric nanotherapeutic systems
Cancer is one of the most fatal diseases, and poses a significant threat to human health. Although many chemotherapeutic agents such as doxorubicin (DOX), cisplatin, paclitaxel (PTX), camptothecin (CPT), plasmid DNA, siRNA etc. have been extensively used for cancer treatment, they have also exhibited some shortcomings, including hydrophobic nature, poor bioavailability, degradation in vivo, deactivation by enzymes, low therapeutic index and side effects on normal tissues. These issues have forced scientists to design and fabricate suitable delivery carriers to overcome these drawbacks.97 With the recent development of nanoscience and nanotechnology, a large number of nanocarriers based on organic, inorganic and organic–inorganic hybrids have been fabricated and examined for these purposes.186,187 Great progress and advances have also been achieved in controlled delivery nanosystems. Among these, stimuli-responsive polymeric delivery nanosystems have become the focus of many researchers.188 In the first part of this review, various redox-responsive polymeric nanosystems including redox-responsive copolymers, nanocapsules, branched polymers and biodegradable polymers will be introduced.
2.1 Redox-responsive copolymers
Given advantages such as good biocompatibility, water dispersibility and designability of structures, the encapsulation of hydrophobic drugs into copolymers with both hydrophobic and hydrophilic segments has been extensively explored for therapeutic agent delivery.189,190 Due to their amphiphilicity, copolymers will self-assemble into different nanostructures with different morphologies and sizes based on the types of hydrophobic and hydrophilic segments adopted and the ratio of hydrophobic and hydrophilic segments. By incorporation of dynamic linkages such as disulfide bonds into these copolymers, redox-responsive polymeric nanocarriers can be fabricated.191–194 Alginate sodium is the sodium salt of alginic acid, an anionic natural polysaccharide distributed widely in the cell walls of brown seaweed, which is composed of homopolymeric blocks of (1-4)-linked glycoside. Alginate sodium has been demonstrated to be food- and skin-safe, and has been widely used across a range of industries, including textile printing, food and pharmaceuticals. In a recent report, Li and colleagues fabricated redox-responsive polymeric nanocarriers for controlled delivery of DOX.195 The redox-responsive polymeric nanocarriers were fabricated in a facile manner via covalent linking of the carboxyl groups of alginate and the amino groups of cystamine using an EDC/NHS reaction. The chemotherapeutic agent (DOX) was readily loaded into the redox-responsive nanogels through electrostatic interactions between the anionic alginate and cationic DOX (Fig. 1A). The DOX-loaded polymeric carriers could be quickly internalized by CAL-72 cells, leading to higher DOX intracellular accumulation and enhanced cell killing effects (Fig. 1B). More importantly, because the DOX was concentrated in the polymeric nanocarriers, the side effects of DOX to normal tissues could thus be avoided or at least reduced through the EPR effect or functionalization with targeting agents. Therefore, the DOX could be effectively internalized by cells and released from the carriers into the cytoplasm, owing to the high GSH concentration in intracellular spaces.
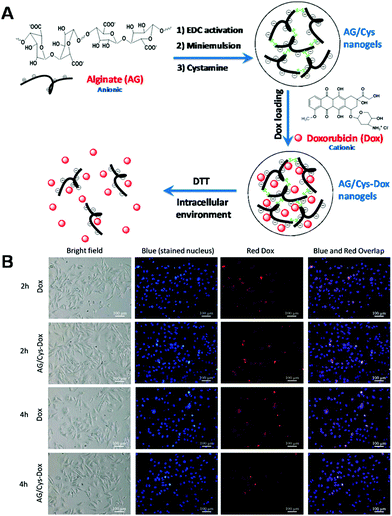 |
| Fig. 1 (A) Schematic illustration of the formation and drug release of DOX-loaded alginate/cystamine (AG/Cys-DOX) nanogels. (B) Bright field and fluorescence microscope images of CAL-72 cells. (Reprinted with permission from ref. 195.) | |
The direct conjugation of hydrophobic drugs to polymers is a very important route for the fabrication of polymeric controlled drug delivery systems. In these drug delivery systems, the drug is protected by hydrophilic polymers, which cover the surface of the polymeric nanocarriers. In a recent study, Cheng et al. fabricated a core cross-linked polymeric drug delivery system via covalent linkage of CPT with redox-responsive core cross-linked polymers, which were synthesized by ring opening-polymerization of Tyr(alkynyl)-OCA and then cross-linked with disulfide-containing azide compounds via azide–alkyne click chemistry (Fig. 2A).168 The drug release profiles showed that the drug release behavior could be controlled well by DTT, indicating the biomedical potential for on-demand drug delivery (Fig. 2B). On the other hand, the authors also demonstrated the drug release behavior of CCL micelles was highly dependent on the composition of the linkages (Fig. 2C). When both the drug (CPT) and the cross-linkages contained redox-responsive bonds (denoted CCL1), CPT could be rapidly released from the micelles in the presence of 10 mM DTT. After the linkages were changed to non-redox-responsive bonds but maintained the CPT conjugation with redox-responsive properties (CCL2), the micelles exhibited only limited redox-responsive behavior in the presence of a certain concentration of DTT. However, no redox-responsive behavior was observed since both the CPT and the cross-linkages were lacking redox-responsive bonds (CCL3). These results suggested that the redox-responsiveness of micelles can be adjusted by designing the composition of the micelles. Therefore, various micelles with different redox-responsiveness could be fabricated by programming the percentages of redox-responsive bonds. This strategy holds great potential for the design of micelles for on-demand drug delivery.
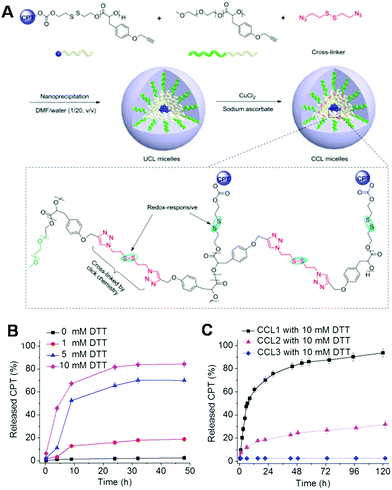 |
| Fig. 2 (A) Preparation of UCL and CCL micelles and (B) in vitro CPT release profiles of CCL1 in phosphate buffered saline (PBS). (C) In vitro CPT release profiles of CCL1, CCL2, and CCL3 in PBS. (Reprinted with permission from ref. 168. Redox-responsive, core-cross-linked micelles capable of on-demand, concurrent drug release and structure disassembly.) | |
2.2 Redox-responsive polymeric nanocapsules
Polymeric nanocapsules, or hollow polymer capsules of nanometer size, have recently attracted significant research attention for potential biomedical applications because of their large hollow space for encapsulation of guest molecules and multifunctional potential via surface design.196 Much research effort has been devoted to fabricating various polymeric nanocapsules through the template-directed layer by layer strategy, template-free supermolecular assembly, or the recently developed template-directed mussel-inspired strategy.100,197,198 Among these, the template-free supermolecular assembly strategy has attracted much attention recently and many polymeric nanocapsules with different compositions and responsive behaviors such as electricity, CO2 and redox responsiveness have emerged.199,200 In order for nanocapsules to be desirable for drug delivery applications, they should fulfill the following requirements: excellent biocompatibility, simple preparation, facile surface functionalization, low cost and responsiveness. In a recent report, Kim and colleagues designed redox-responsive polymeric nanocapsules through a template-free strategy that relied on the host–guest interactions between cucurbit[6]uril(CB[6]) and polyamines (Fig. 3).201 More importantly, many other functional agents such as targeting ligands and imaging probes could be facilely incorporated into these polymer nanocapsules by linking them with polyamines. In this work, both redox-responsive and non-redox-responsive polymeric nanocapsules could be facilely prepared via covalent linkage of CB[6] with redox-responsive (containing disulfide bonds) or non-redox-responsive (without disulfide bonds) small molecules. These polymeric nanocapsules could be used to encapsulate carboxyfluorescein (CF), and the release behavior of these polymeric nanocapsules, denoted CF@4 (linked with disulfide bonds) and CF@5 (linked with C–C single bonds), was investigated. The results suggested that CF@4 exhibited good redox responsiveness in the presence of 100 mM DDT, while CF@5 showed negative responsiveness towards DDT. Furthermore, targeting ligands could be facilely introduced onto the surface of the polymeric nanocapsules via host–guest interactions to form CF@4∩6 and CF@5∩6 (Fig. 3). The cell uptake results suggested that CF@4∩6 bearing glucose could be easily internalized by HepG2 cells and release the cargo due to the high redox state in intracellular spaces. Although the polymeric nanocapsules CF@5∩6 could also be internalized by cells, almost no cargo release was observed due to their lack of redox-responsive bonds. As compared with core–shell polymeric nanocarriers, these nanocapsules demonstrated a larger space for the encapsulation of cargo and more facile surface functionalization of other components.
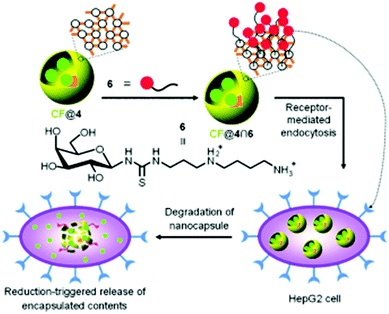 |
| Fig. 3 Schematic representation of the noncovalent surface modification of the CF-encapsulating polymer nanocapsule (CF@4) with 6 through host–guest interactions, receptor-mediated endocytosis, and autonomous triggered release of the encapsulated CF into the cytosol. (Reprinted with permission from ref. 201.) | |
2.3 Redox-responsive biodegradable polymers
The biodegradability of biomaterials is very important for their biomedical applications.202,203 In particular, biodegradable drug delivery carriers can effectively avoid the accumulation and toxicity of materials in living organisms.204 Over the past few decades, various biodegradable polymers including many polysaccharides (e.g. chitosan, starch, dextran and hyaluronic acid) and synthetic degradable polymers (e.g. polylactic acid (PLA), polycaprolactone (PCL) and polycarbonate) have been considered as candidates for drug delivery.205–209 Through the incorporation of stimuli-responsive linkages into these biodegradable polymers, drug delivery carriers with both responsiveness and biodegradability could be fabricated. Zhu et al. recently reported the preparation of polymeric drug delivery nanocarriers via a “one-pot” strategy, and examined the redox-responsive properties of these polymeric carriers.210 As shown in Fig. 4A, an amphiphilic triblock copolymer was synthesized via polycondensation of the hydroxyl group of PEG with the carboxyl group of DL-thiomalic acid to obtain poly(oligo(ethylene glycol)thiomalicate) (POEGTMA) with multiple pendant mercapto groups, and then its terminal hydroxyl groups further served as initiators for the ring-opening polymerization of 3-caprolactone. An amphiphilic copolymer, denoted PCL-b-POEGTMA-b-PCL, could thus be obtained. Due to the existence of biodegradable hydrophobic segments (PCL), the amphiphilic triblock copolymer could be readily self-assembled into spherical nanoparticles with a size of about 45 nm (Fig. 4B). After the therapeutic drug (PTX) was loaded into these micelles, the size of the micelles increased to about 65 nm according to the TEM images (Fig. 4C). The increase in size of the micelles could likely be ascribed to the loading of PTX, which would increase the hydrophobic nature of the micellar core. Moreover, the amphiphilic copolymer still kept its structure in dimethylformamide (DMF), which could dissolve both the hydrophobic and the hydrophilic segments of the copolymers (Fig. 4D). The above results suggested that these micelles were perfectly cross-linked by disulfide bonds. The in vitro release of PTX from these micelles was further determined. It could be observed that almost no drugs were released from the micelles in the absence of DTT. However, the PTX was rapidly released from the micelles in the presence of 10 mM DTT. These results confirmed that PCL-b-POEGTMA-b-PCL is a promising redox-responsive drug carrier with biodegradable properties. To date, many other strategies have also been adopted to fabricate biodegradable polymers with stimuli-responsive properties.124 These types of polymeric drug delivery systems should provide important platforms for various biomedical applications.
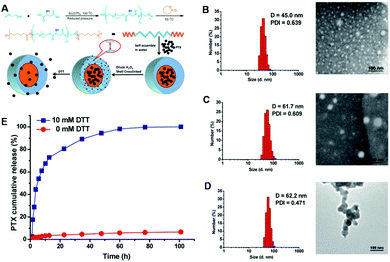 |
| Fig. 4 (A) Preparation of SCL micelles for redox-responsive drug release. Sizes, distributions and morphologies of SCL micelles were measured by DLS and TEM: (B) SCL micelles in water, (C) PTX-loaded SCL micelles in water and (D) SCL micelles in DMF. (E) In vitro PTX release profiles from SCL micelles in aqueous solution with and without DTT. (Reprinted with permission from ref. 210.) | |
2.4 Redox-responsive poly(amino acid)s
Amino acids are small biomolecules, which are expected to possess good biocompatibility as compared with many other monomers due to their biogenic nature. The polymerization of amino acids to afford poly(amino acid)s has therefore become a focus in the field of biomedicine.211 To date, a number of amino acids have been converted to their N-carboxyanhydride (NCA) form, which can be further polymerized with other components to obtain poly(amino acid)s with diverse side functional groups through ring-opening polymerization.212 These copolymers containing poly(amino acid)s are expected to be promising candidates for various biomedical applications such as gene and drug delivery.212–214 For example, Zhuang and colleagues have recently prepared PEG coated poly(amino acid)s and examined their DOX delivery properties.215 In this work, the copolymers were fabricated via one-step ring-opening polymerization of two NCAs and an amino-terminated PEG. One of these NCAs contained disulfide bonds and the other NCA possessed an aromatic ring. The thus obtained amphiphilic copolymers could form nanometer spheres in pure aqueous solution, which could be effectively loaded with DOX through electrostatic interactions and then release their cargo into the cytoplasm due to the rupture of the disulfide bonds. The results demonstrated that these poly(amino acid)s possessed well-controlled release behavior, which was dependent on the concentration of DTT. The cell internalization and intracellular behavior of the DOX-loaded polymeric carriers were monitored by CLSM. Compared with cells that had not undergone pretreatment with GSH (Fig. 5A and C), cells pretreated with GSH (Fig. 5B and D) showed much stronger DOX fluorescence in cells. These results suggested that the nanocarriers could also respond at the cellular level because the GSH treatment could improve the intracellular concentration of DOX. Besides DOX, other therapeutic agents such as cisplatin, DNA and siRNA could also be delivered into cells in a controlled manner using the poly(amino acid)-based polymeric delivery carriers by designing their chemical properties and composition.216
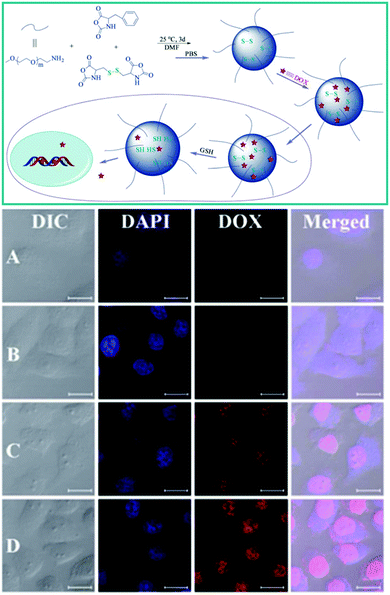 |
| Fig. 5 Schematic illustration of the preparation, drug loading and intracellular release of PEG–poly(amino acid)s NGs. Representative CLSM images of HeLa cells incubated with DOX-loaded NG-3. (Reprinted with permission from ref. 215.) | |
2.5 Redox-responsive branched polymers
The architecture of polymers is very important for their microstructure and properties. Branched polymers are an important type of polymer that possess different properties from linear polymers.217,218 In particular, branched polymers may not only exhibit different physicochemical properties from linear ones, but also have promising properties for biological applications.219–221 Various branched polymers such as dendrimers, branched polyethylenimine (PEI), star polymers, brush polymers, dumbbell and comb polymers have been prepared.222–224 Among these, branched PEI is well known for gene delivery due to its excellent DNA binding capability and cell uptake behavior. However, the DNA binding capability of PEI is mainly dependent on its molecular weight. High molecular weight PEI exhibits better DNA binding capability and transfection efficiency, and significant toxicity. Chemical modification of low molecular weight PEI is a general route to reduce toxic effects while maintaining the high DNA binding capability and transfection efficiency. Great efforts have been devoted to this field and a large number of gene transfection agents with better performance have been developed.225 Apart from high DNA binding capability, another important factor influencing the transfection efficiency must be avoided, which is the degradation of DNA by lysosomes or endosomes. Therefore, the incorporation of stimuli-responsive bonds into these low molecular weight PEI-based gene delivery carriers plays a crucial role in improving their transfection efficiency.226,227 Bae et al. recently reported the preparation of reducible PEI gene carriers via incorporation of disulfide bonds into gene carriers.179 In this work, the primary amino groups of low molecular weight branched PEI were first reacted with 2-iminothiolane to obtain thiolated low molecular weight branched PEI. Then, the thiolated low molecular weight branched PEI was further polymerized into high molecular weight branched PEI in the presence of DMSO, and terminated with cysteine (Fig. 6A). The high molecular weight reducible PEI could release its cargo in intracellular milieu via fragmentation and ion-exchange. As compared with the high molecular weight branched PEI (PEI25kDa), the high molecular weight reducible branched PEI showed much lower cytotoxicity and even better transfection efficiency (Fig. 6B). These results suggested that the incorporation of redox-responsive bonds into branched PEI may be a very efficient way to improve the gene transfection efficiency and reduce the toxicity of gene carriers at the same time. On the other hand, the strategy described in this work might also be extended to fabricate many other redox-responsive polymeric carriers for controlled drug delivery and gene transfection.
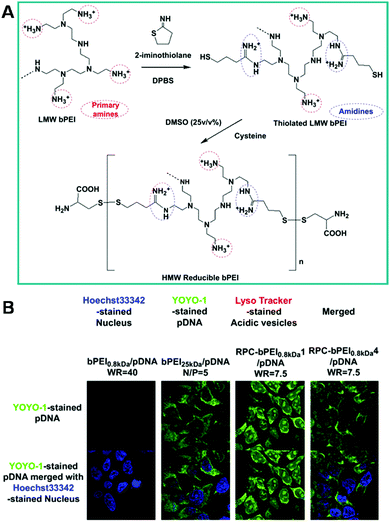 |
| Fig. 6 (A) The synthesis of bPEI-based reducible polycations (RPC-bPEI). (B) Intracellular localization of pDNA delivered by RPC-bPEI 0.8 kDa, bPEI 25 kDa, and bPEI 0.8 kDa in polyplex-transfected HEK293 cells 4 h after transfection. (Reprinted with permission from ref. 179.) | |
Dendritic polymers are another type of branched polymer that have received much attention for biomedical applications due to their special topological structure and promising physicochemical/biological properties.228,229 It has been demonstrated that dendrimers may exhibit significantly different viscosity and pharmacokinetical behavior in vivo.230 Unlike classical polymers, dendrimers are highly uniform with a narrow molecular weight distribution, and a specific size and shape. More importantly, a large number of functional groups exist on their surface, which are very important for the further functionalization of dendrimers.230 To date, diverse dendrimers with different terminal functional groups such as amino and hydroxyl groups have been precisely synthesized through well-developed synthetic strategies.231 The most well-known dendritic polymers are poly(amido amine) (PAMAM) dendrimers and boltorn hyperbranched polymers.232,233 Both have been extensively investigated for drug delivery and gene transfection applications. Combining the interesting properties of dendritic polymers and the redox-responsive properties of disulfide bonds, promising drug delivery carriers can be fabricated.230 In a recent report, Yan et al. demonstrated the fabrication of redox-responsive polymeric drug delivery systems via integration of a boltorn hyperbranched polymer (H40 star polymer), biodegradable PLA, polyphosphate and redox-responsive disulfide bonds.234 The intracellular delivery of chemotherapeutic agents (DOX) using the obtained polymers (H40-star-PLA-SS-PEP) was further conducted (Fig. 7A). The amphiphilic polymers could self-assemble into spherical nanoparticles with a size of about 70 nm in aqueous solution (Fig. 7B). The GSH-mediated intracellular delivery of DOX was also demonstrated. As shown in Fig. 7C, the DOX-loaded H40-star-PLA-SS-PEP micelles showed negative cytotoxicity toward HeLa cells when the concentration of DOX was 0.18 μg mL−1. When the cells were pretreated with buthionine sulfoximine (BSO), the drug-loaded polymers showed even lower cytotoxicity toward the cells. However, greatly enhanced cytotoxicity was observed when the cells were pretreated with 10 mM GSH-OEt. These results suggested that the drug release of the drug-loaded polymers could be controlled well by the redox state of the cells. If these dendrimers were functionalized with other stimuli-responsive bonds, many other controlled drug delivery systems could also be fabricated. These dendritic polymer-based drug delivery systems are promising platforms for biomedical applications because of their interesting properties.
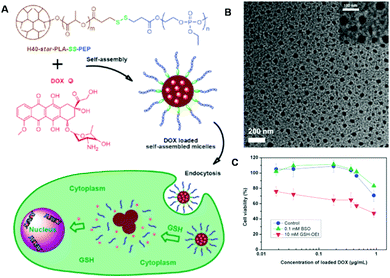 |
| Fig. 7 (A) Illustration of redox-responsive micelles based on H40-star-PLA-SS-PEP for intracellular DOX release triggered by GSH tripeptide. (B) TEM images of H40-star-PLA-SS-PEP micelles. (C) Viability of HeLa cells cultured with DOX-loaded H40-star-PLA-SS-PEP micelles at different concentrations for 48 h. (Reprinted with permission from ref. 234). | |
2.6 Redox-responsive organic–inorganic nanosystems
Organic–inorganic hybrid materials have attracted great interest for various biomedical applications over the past few decades. Novel material systems can be fabricated by combining the advantages of organic and inorganic components.235–243 Among organic–inorganic hybrids, nanocomposites based on mesoporous silica nanoparticles have attracted the most research attention due to their controllable preparation, ease of surface functionalization, high specific surface area, low cost and good biocompatibility. Since their discovery, mesoporous silica nanoparticles have been widely explored for diverse applications, such as drug delivery carriers, catalyst supports and highly efficient adsorption materials.9,244 In particular, the utilization of mesoporous silica nanoparticles as drug delivery carriers has been a focus for their application.245–247 Surface conjugation with polymers is often adopted to improve the properties and performance of mesoporous silica nanoparticles for biomedical applications.244 Surface functionalization with polymers can not only enhance the water dispersibility of mesoporous silica nanoparticles, but also provide responsive gates for controlling the release behavior of the cargo.138 A variety of gates such as responsive polymers, cyclodextrin, aptamers and nanoparticles have been reported.137,138,166 The underlying mechanism for controlled release behavior relies on the responsiveness of gates toward different stimuli. For example, Yang et al. have prepared controlled drug delivery systems via surface modification of mesoporous silica nanoparticles with redox-responsive polymers.248 In their work, mesoporous silica nanoparticles with C
C bonds were prepared via hydrolysis of tetraethyl orthosilicate (TEOS) using cetyltrimethylammonium bromide (CTAB) as a soft template. After removal of CTAB through ion exchange, the C
C bond-containing mesoporous silica nanoparticles were surface-functionalized with redox-responsive polymers through polymerization with methacrylic acid, N-vinylcaprolactam and N,N′-bis-(acryloyl)cystamine. To enhance the interactions between the mesoporous silica nanoparticles and the drug, carboxyl groups were also introduced into the controlled drug delivery system by reacting the amino groups of 3-aminopropyltriethoxysilane (APS) with succinic anhydride (Fig. 8A). The loading and controlled release of DOX from these functionalized mesoporous silica nanoparticles were also examined. Fig. 8B shows the CLSM images of cells that were incubated both with free DOX and with DOX-loaded mesoporous silica nanoparticles for 0.5 h and 2 h. These results demonstrated that mesoporous silica nanoparticles can significantly enhance the cell uptake capability of DOX and release the cargo in the cytoplasm. Based on these redox-responsive inorganic–organic drug delivery systems, other stimuli-responsive drug delivery systems could also be developed.
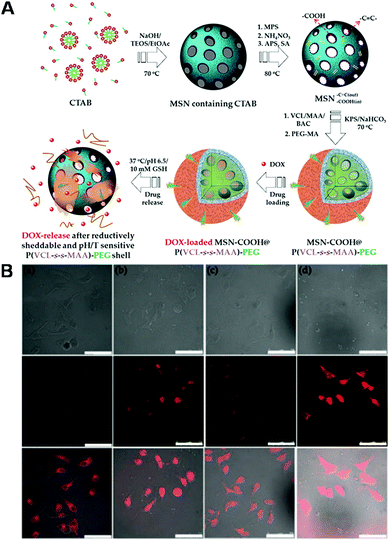 |
| Fig. 8 (A) Illustration of the synthetic process for core/shell hybrid mesoporous silica nanoparticles coated with a reductively sheddable and thermo/pH-sensitive P(VCL-s-s-MAA)–PEG shell by precipitation polymerization. (B) CLSM images of intracellular DOX release from MSN–COOH@P(VCL-s-s-MAA)–PEG composites using MCF-7 cells. (Reprinted with permission from ref. 248.) | |
2.7 Dual-responsive polymeric systems
Stimuli-responsive polymeric therapeutic nanosystems have attracted considerable attention for their prospective biomedical applications.249 These polymeric delivery nanosystems can undergo reversible changes in response to different stimuli such as pH, temperature, redox conditions, enzymes or glucose.250 Great progress has been made in the design and biomedical applications of stimuli-responsive polymeric delivery systems, however, most of them could only respond to a single stimulus. The development of polymeric delivery systems that can simultaneously respond to multiple stimuli independently and precisely control the release of cargo is of great interest.251–257 Yuan and colleagues have recently developed dual redox- and temperature-responsive polymeric delivery systems for multi-controlled drug release.258 The preparation of polymeric delivery systems is shown in Fig. 9A. First, the diblock copolymer was obtained via atom transfer radical polymerization (ATRP) using disulfide bond-containing PEG as a macroinitiator and with 2-(N,N-dimethylamino)ethyl methacrylate (DMAEMA) as the monomer. Then, the diblock copolymer could further interact with α-cyclodextrin to form supermolecular micelles (PRX-SS-PDMAEMA) with multi-responsive behavior. The controlled release of cargo from these supermolecular micelles was further investigated. The drug release could be controlled well by the temperature and the concentration of GSH (Fig. 9B and C). As compared with single-responsive drug delivery systems, dual-responsive delivery systems can more precisely control the cargo release behavior. Therefore, more effective drug delivery systems can be fabricated by designing responsiveness to multiple stimuli.
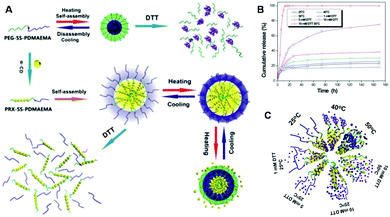 |
| Fig. 9 (A) Self-assembly, disassembly and multi-responsiveness of PEG-SS-PDMAEMA and PRX-SS-PDMAEMA. (B) Controlled release of DOX from the micelles at 25 °C (△), 40 °C (□), 50 °C (▽), with 1 mM DTT (○), 5 mM DTT (◇), 10 mM DTT (◁) and 10 mM DTT at 50 °C (☆). (C) Schematic illustration for the controlled release of DOX with different stimuli. (Reprinted with permission from ref. 258.) | |
Besides temperature- and redox-responsive drug delivery systems, other dual-responsive polymeric drug delivery systems have also been developed recently.259 For example, Liu et al. have reported the preparation of redox- and pH-responsive drug delivery polymeric nanocarriers and investigated their controlled drug delivery behavior.260 The polymeric drug delivery systems were fabricated by cross-linking benzaldehyde group-containing amphiphilic diblock copolymers (PCL-b-P(OEGMA-co-MAEBA)) using disulfide bond-containing dithiolbis(propanoic dihydrazide) in aqueous solution (Fig. 10). The loading and controlled release of anticancer agents (CPT or DOX) using the obtained shell cross-linked polymeric micelles were further studied. The results suggested that only 20% of the drug was released from the shell cross-linked micelles over 100 h, however, the drug release ratio was almost 80% for non-cross-linked micelles. When the pH value was changed to 5.0, the drug release from the shell cross-linked micelles was increased to greater than 60%. On the other hand, if the cross-linked micelles were treated with 10 mM DTT, the drug release percentage was 55%. The drug release behavior was very similar to the non-cross-linked micelles when cross-linked micelles were treated with 10 mM DTT at pH 5.0. The above results suggested that drug release from cross-linked micelles can be controlled well using both pH and redox conditions. These dual-responsive polymeric drug delivery systems with precise drug release behavior are very promising for the design of better drug delivery vehicles and smart nanocarriers.
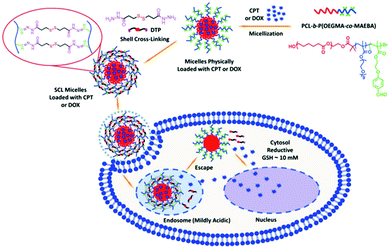 |
| Fig. 10 Schematic illustration for the fabrication of shell cross-linked (SCL) micelles of PCL-b-P(OEGMA-co-MAEBA) diblock copolymers physically loaded with CPT or DOX within a hydrophobic PCL core. The obtained dynamic covalent SCL micelles exhibited thiol and pH dual-modulated drug release characteristics due to the dual-sensitive nature of the DTP crosslinker. (Reprinted with permission from ref. 260.) | |
2.8 Multi-responsive polymeric systems
Multi-responsive polymeric drug delivery systems have also been designed by some groups.261–263 For example, Jiang et al. have fabricated triple stimuli-responsive copolymers for therapeutic delivery.264 As shown in Fig. 11, the multi-responsive polymeric nanocarriers were composed of a hydrophilic PEG, polymers containing imidazole groups, disulfide bonds, and a small molecular weight PEG monomer. When the amphiphilic copolymers were dispersed in aqueous solution, they could self-assemble into nanoparticles with a size of about 60 nm, thus promoting the accumulation of carriers in tumor tissues by the EPR effect. The hydrophilic segment (mPEG5000) as well as the polymerized small molecular weight PEG monomer were spread over the surface of these nanocarriers as the corona, resulting in prolonged circulation in the blood. When the micelles accumulated in tumor sites (weak acidic conditions), the particle surface became positively charged because of the ionization of the imidazole group, which could facilitate cellular uptake through endocytosis. When the micelles were internalized by cells and in the endosomes, they were dissociated and destabilized, leading to complete ionization of the imidazole groups and rapid release of the cargo inside the cytoplasm. On the other hand, the micelles could also respond to the redox conditions and the existence of S–S bonds. The micelles would also be dissociated due to the greater abundance of reductive GSH in the intracellular compartments, resulting in significantly enhanced intracellular drug release. PEG is a novel temperature-responsive polymer with good water solubility and biocompatibility. Therefore, the micelles containing PEG segments could also respond to temperature. The detailed responsiveness of these micelles toward pH, redox conditions and temperature was further investigated. As shown in Fig. 11A, the release behavior of PTX from the micelles at different pH values (5.0 and 7.4) was compared. It can be seen that PTX release from the micelles at pH 5.0 was much faster than at pH 7.4. The redox-responsiveness of the micelles is shown in Fig. 11B. It can be seen that the release of the drug from the micelles was rather slow in the absence and presence of 10 μM DTT. Much faster drug release could be observed when the drug-loaded carriers were dispersed in the presence of 10 mM DTT. Furthermore, the thermo-responsiveness of the micelles was also investigated. The results showed that these micelles exhibited a more rapid phase transition at 37 °C than 25 °C, indicating the thermo-response behavior of the copolymers. These results suggested that multi-responsive drug delivery systems, which can respond to pH, temperature and redox conditions, can be fabricated by integrating imidazole groups, disulfide bonds and PEG into copolymers. This type of polymeric drug delivery system can control the release of cargo well by taking advantage of the difference between tumor and normal tissues, extracellular and intracellular spaces. This should be of great research interest for the fabrication of next-generation drug delivery systems.
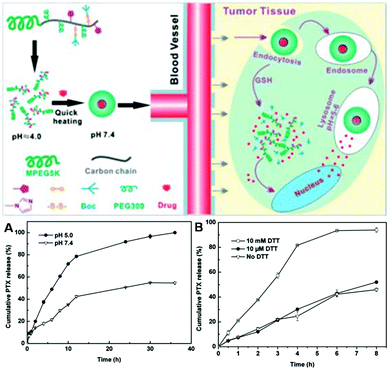 |
| Fig. 11 Schematic illustration of drug loading and stimuli-responsive release from p(PEG-MEMA-co-Boc-Cyst-MMAm-co-VI)-b-PEG micelles. (A) pH-dependent PTX release from PPBV micelles at different pH values in PBS buffer. (B) DTT-dependent PTX release from PPBV micelles in PBS buffer (containing 0.1% Tween 80) with and without DTT. Data represent the mean and standard deviation of three independent experiments. (Reprinted with permission from ref. 264.) | |
2.9 Other redox-responsive polymeric systems
Similarly to disulfide bonds, diselenide bonds have also been utilized for the fabrication of redox-responsive polymeric nanosystems. Apart from redox-responsiveness, selenium-containing agents have also been previously used as antioxidants in pharmacochemistry.182,184,185 As compared with disulfide bonds, diselenide bonds can respond to both oxidants and reductants. It has been demonstrated that diselenide bonds can be oxidized to seleninic acid after oxidation and reduced to selenol in the presence of a reducing environment. Therefore, polymers containing diselenide bonds are expected to be more sensitive to both oxidation and reduction stimuli. Over the past few years, Profs Zhang and Xu at Tsinghua University have developed many redox-responsive diselenide bond-containing polymers.182,184 The responsiveness of these polymers towards various reduction and oxidation stimuli has also been investigated in detail.265–271 The cleavage energy of diselenide bonds is lower than that of disulfide bonds, and therefore diselenide bond-containing polymers will exhibit higher sensitivity towards stimuli as compared with disulfide bond-containing polymers. For example, Xu and colleagues have prepared dual redox-responsive diselenide bond-containing copolymers.182 In this work, small molecules containing diselenide bonds and diol structures were first polymerized with toluene diisocyanate (TDI) and reacted with PEG monomethyl ether. The final triblock copolymers with PEG and diselenide bonds were denoted as PEG–PUSeSe–PEG. They showed amphiphilic properties and dissolved well in common solvents (scheme in Fig. 12). After these copolymers were dispersed in pure aqueous solution, they could self-assemble into spherical nanoparticles (Fig. 12A). The hydrodynamic size of these micelles was about 76 nm based on dynamic light scattering (DLS) measurements. Because the diselenide bonds were encapsulated in the core, these micelles were very stable in an ambient environment. Moreover, hydrophobic dyes (RB) could be loaded into these micelles via hydrophobic interactions owing to the amphiphilicity of the micelles (Fig. 12B). The release of RB from the redox-responsive micelles was also investigated. It could be observed that the release ratio of RB from the micelles was clearly dependent on the concentration of H2O2 and GSH (Fig. 12C and D). A much faster release ratio of RB from Se–Se cross-linked micelles could be observed at high concentrations of H2O2 (0.1%) and GSH (0.1 mg mL−1). These results suggested that diselenide bond-containing micelles exhibit excellent reduction and oxidation responsiveness with high sensitivity. As compared with disulfide bonds, diselenide bonds are expected to be more sensitive in terms of reduction and oxidation responsiveness. Therefore, diselenide bond-based copolymers are a novel type of redox-responsive platform, which could offer great potential for controlled drug release.
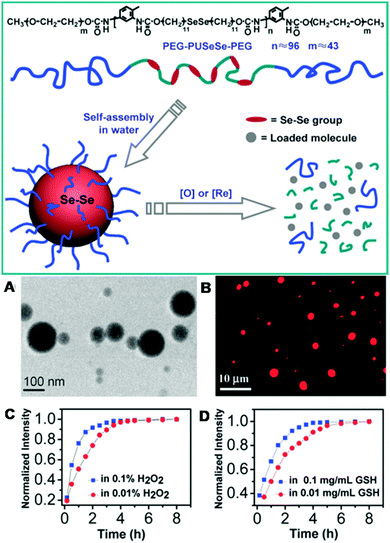 |
| Fig. 12 Structure of PEG–PUSeSe–PEG and schematic of the redox-responsive disassembly of PEG–PUSeSe–PEG micelles. (A) Cryo-TEM image of PEG–PUSeSe–PEG micelles. (B) FM image of RB-loaded micelles, and the release behavior of RB in (C) H2O2 and (D) GSH. (Reprinted with permission from ref. 182.) | |
Apart from reduction and oxidation responses, other stimuli such as γ-rays may also be utilized to trigger the degradation of diselenide bonds and the release of cargos.269 For example, Zhang and Xu et al. have recently reported an investigation of triblock copolymers containing repeat diselenide bonds for the γ-ray responsive release of DOX and the combined therapeutic effects of chemotherapy and radiotherapy (Fig. 13).269 In this work, the anticancer drug (DOX) was encapsulated in the amphiphilic triblock copolymers through hydrophobic interactions. After the drug-loaded copolymers were irradiated with different dosages of γ -rays, the copolymers showed dose responsive drug release behavior (Fig. 13A). Of note, about 50% of the cargos were released after irradiation with 50 Gy γ-rays for 2 h (Fig. 13B). More importantly, radiotherapy is a very important physical therapy strategy for cancer treatment. In this work, Xu et al. elegantly combined the effects of γ-rays for controlled drug release and therapeutics. Therefore, copolymers containing diselenide bonds may open a new avenue for combination of chemotherapy and radiotherapy. Apart from diselenide bonds, the redox- and ROS-responsiveness of tellurium-containing copolymers have also been developed by Xu et al. to examine their drug delivery potential.185
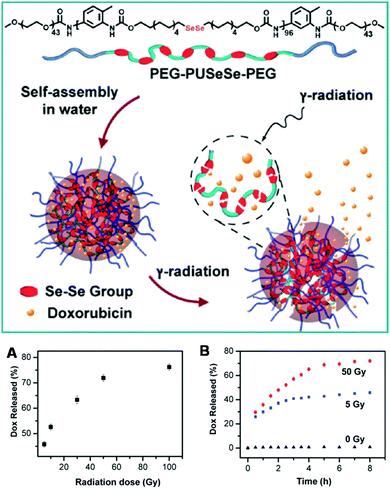 |
| Fig. 13 Schematic illustration of the disassembly and drug release of the radiation-sensitive PEG–PUSeSe–PEG aggregates under exposure to γ-radiation. (A) Relationship between the release percentage of DOX and γ-radiation dose and (B) release plots of DOX from the PEG–PUSeSe–PEG aggregates under exposure to γ-radiation doses of 5 and 50 Gy, with plots of 0 Gy for reference. The time in this figure represents the release time after γ-radiation. (Reprinted with permission from ref. 269.) | |
3. Conclusion
Stimuli-responsive polymeric nanocarriers have been intensively investigated over the past few decades. A large number of polymeric nanosystems that could respond to stimuli such as pH, redox conditions, temperature, enzymes etc. have been designed for various biomedical applications. Among these, redox-responsive polymeric nanocarriers have been one of the most adopted for biomedical applications due to the significantly different GSH concentrations between intracellular and extracellular spaces. In this review article, different redox-responsive polymeric nanosystems including redox-responsive copolymers, polymeric nanocapsules, redox-responsive poly(amino acid)s, branched polymers, redox-responsive organic–inorganic nanosystems, dual responsive polymeric systems, multi-responsive polymeric systems and many other redox-responsive polymeric systems have been discussed. The biomedical applications of these responsive polymeric nanosystems for drug, protein and gene delivery have also been highlighted. To date, these systems have relied on disulfide bonds for redox-responsiveness, and novel redox-responsive linkages such as diselenide bonds and ditellurium bonds should be explored. On the other hand, many of these redox-responsive delivery systems for biomedical applications have mainly focused on the in vitro stage. More in vivo and clinical studies are needed in the future.
Acknowledgements
This research was supported by the National Science Foundation of China (No. 21201108, 51363016, 21564006, 21561022), the Natural Science Foundation of Jiangxi Province in China (No. 20161BAB203072, 20161BAB213066) and the National 973 Project (No. 2011CB935700).
References
- S. K. Sahoo and V. Labhasetwar, Drug Discovery Today, 2003, 8, 1112–1120 CrossRef CAS PubMed
.
- V. Delplace, P. Couvreur and J. Nicolas, Polym. Chem., 2014, 5, 1529–1544 RSC
.
- K. Liu, Z. Xu and M. Yin, Prog. Polym. Sci., 2015, 46, 25–54 CrossRef CAS
.
- M. Chen and M. Yin, Prog. Polym. Sci., 2014, 39, 365–395 CrossRef CAS
.
- A. K. Gupta and M. Gupta, Biomaterials, 2005, 26, 3995–4021 CrossRef CAS PubMed
.
- J. Zhu and X. Shi, J. Mater. Chem. B, 2013, 1, 4199–4211 RSC
.
- J. Zhang, J. Gao, M. Chen and Z. Yang, Antioxid. Redox Signaling, 2014, 20, 2179–2190 CrossRef CAS PubMed
.
- X. Ma, C. Teh, Q. Zhang, P. Borah, C. Choong, V. Korzh and Y. Zhao, Antioxid. Redox Signaling, 2014, 21, 707–722 CrossRef CAS PubMed
.
- X. Zhang, X. Zhang, S. Wang, M. Liu, Y. Zhang, L. Tao and Y. Wei, ACS Appl. Mater. Interfaces, 2013, 5, 1943–1947 CAS
.
- J. Hui, X. Zhang, Z. Zhang, S. Wang, L. Tao, Y. Wei and X. Wang, Nanoscale, 2012, 4, 6967–6970 RSC
.
- L. Alili, M. Sack, C. von Montfort, S. Giri, S. Das, K. S. Carroll, K. Zanger, S. Seal and P. Brenneisen, Antioxid. Redox Signaling, 2013, 19, 765–778 CrossRef CAS PubMed
.
- Y. Yuan, R. T. Kwok, B. Z. Tang and B. Liu, J. Am. Chem. Soc., 2014, 136, 2546–2554 CrossRef CAS PubMed
.
- D. Ding, K. Li, Z. Zhu, K.-Y. Pu, Y. Hu, X. Jiang and B. Liu, Nanoscale, 2011, 3, 1997–2002 RSC
.
- Y. Lu, B. He, J. Shen, J. Li, W. Yang and M. Yin, Nanoscale, 2015, 7, 1606–1609 RSC
.
- V. Wagner, A. Dullaart, A.-K. Bock and A. Zweck, Nat. Biotechnol., 2006, 24, 1211–1217 CrossRef CAS PubMed
.
- Y. Yang, Q. Zhao, W. Feng and F. Li, Chem. Rev., 2013, 113, 192–270 CrossRef CAS PubMed
.
- J. Yao, M. Yang and Y. Duan, Chem. Rev., 2014, 114, 6130–6178 CrossRef CAS PubMed
.
- C. Zhu, L. Liu, Q. Yang, F. Lv and S. Wang, Chem. Rev., 2012, 112, 4687–4735 CrossRef CAS PubMed
.
- M. Liu, J. Ji, X. Zhang, X. Zhang, B. Yang, F. Deng, Z. Li, K. Wang, Y. Yang and Y. Wei, J. Mater. Chem. B, 2015, 3, 3476–3482 RSC
.
- Q. Wan, M. Liu, D. Xu, H. Huang, L. Mao, G. Zeng, F. Deng, X. Zhang and Y. Wei, J. Mater. Chem. B, 2016, 4, 4033–4039 RSC
.
- Q. Wan, G. Zeng, Z. He, L. Mao, M. Liu, H. Huang, F. Deng, X. Zhang and Y. Wei, J. Mater. Chem. B, 2016, 4, 5692–5699 RSC
.
- A. Bianco, K. Kostarelos, C. D. Partidos and M. Prato, Chem. Commun., 2005, 571–577 RSC
.
- P. V. Pawar, S. V. Gohil, J. P. Jain and N. Kumar, Polym. Chem., 2013, 4, 3160–3176 RSC
.
- H. Liu, Y. Xu, S. Wen, J. Zhu, L. Zheng, M. Shen, J. Zhao, G. Zhang and X. Shi, Polym. Chem., 2013, 4, 1788–1795 RSC
.
- X. Ke, D. J. Coady, C. Yang, A. C. Engler, J. L. Hedrick and Y. Y. Yang, Polym. Chem., 2014, 5, 2621–2628 RSC
.
- D. J. Naczynski, M. C. Tan, R. E. Riman and P. V. Moghe, J. Mater. Chem. B, 2014, 2, 2958–2973 RSC
.
- M. Faisal, Y. Hong, J. Liu, Y. Yu, J. W. Lam, A. Qin, P. Lu and B. Z. Tang, Chem. – Eur. J., 2010, 16, 4266–4272 CrossRef CAS PubMed
.
- F.-m. Gong, Z.-q. Zhang, X.-d. Chen, L. Zhang, X.-s. Yu, Q.-h. Yang, X.-t. Shuai, B.-l. Liang and D. Cheng, Chin. J. Polym. Sci., 2014, 32, 321–332 CrossRef CAS
.
- N. Li, Y. Jin, L.-z. Xue, P.-l. Li, D.-y. Yan and X.-y. Zhu, Chin. J. Polym. Sci., 2013, 31, 530–540 CrossRef CAS
.
- P. Ren, Y.-b. Wu, W.-l. Guo, S.-x. Li and Y. Chen, Chin. J. Polym. Sci., 2013, 31, 285–293 CrossRef CAS
.
- Y. Zhang, X.-j. Wang, M. Guo, H.-S. Yan, C.-h. Wang and K.-l. Liu, Chin. J. Polym. Sci., 2014, 32, 1329–1337 CrossRef CAS
.
- N.-n. Zhao, W. Nie, J. Mao, W.-c. Wang and X.-l. Ji, Chin. J. Polym. Sci., 2013, 31, 1233–1241 CrossRef CAS
.
- X. Zhang, X. Zhang, S. Wang, M. Liu, L. Tao and Y. Wei, Nanoscale, 2013, 5, 147–150 RSC
.
- C. Lin, C.-J. Blaauboer, M. M. Timoneda, M. C. Lok, M. van Steenbergen, W. E. Hennink, Z. Zhong, J. Feijen and J. F. Engbersen, J. Controlled Release, 2008, 126, 166–174 CrossRef CAS PubMed
.
- S. Ganesh, A. K. Iyer, D. V. Morrissey and M. M. Amiji, Biomaterials, 2013, 34, 3489–3502 CrossRef CAS PubMed
.
- A. Singh, M. Talekar, T.-H. Tran, A. Samanta, R. Sundaram and M. Amiji, J. Mater. Chem. B, 2014, 2, 8069–8084 RSC
.
- J. Kost and R. Langer, Adv. Drug Delivery Rev., 2012, 64, 327–341 CrossRef
.
- S. E. Averick, E. Paredes, A. Irastorza, A. R. Shrivats, A. Srinivasan, D. J. Siegwart, A. J. Magenau, H. Y. Cho, E. Hsu and A. A. Averick, Biomacromolecules, 2012, 13, 3445–3449 CrossRef CAS PubMed
.
- X. Zhang, X. Zhang, S. Wang, M. Liu, Y. Zhang, L. Tao and Y. Wei, ACS Appl. Mater. Interfaces, 2013, 5, 1943–1947 CAS
.
- R. Wang and F. Zhang, J. Mater. Chem. B, 2014, 2, 2422–2443 RSC
.
- J. Wang, T. T. Wang, P. F. Gao and C. Z. Huang, J. Mater. Chem. B, 2014, 2, 8452–8465 RSC
.
- S. Joshi-Barr, C. de Gracia Lux, E. Mahmoud and A. Almutairi, Antioxid. Redox Signaling, 2014, 21, 730–754 CrossRef CAS PubMed
.
- K. Wang, X. Zhang, X. Zhang, B. Yang, Z. Li, Q. Zhang, Z. Huang and Y. Wei, J. Mater. Chem. C, 2015, 3, 1854–1860 RSC
.
- K. Wang, X. Zhang, X. Zhang, B. Yang, Z. Li, Q. Zhang, Z. Huang and Y. Wei, Colloids Surf., B, 2015, 126C, 273–279 CrossRef PubMed
.
- K. Wang, X. Yuan, Z. Guo, J. Xu and Y. Chen, Carbohydr. Polym., 2014, 102, 699–707 CrossRef CAS PubMed
.
- O. C. Farokhzad, S. Jon, A. Khademhosseini, T.-N. T. Tran, D. A. LaVan and R. Langer, Cancer Res., 2004, 64, 7668–7672 CrossRef CAS PubMed
.
- G. Feng, C. Y. Tay, Q. X. Chui, R. Liu, N. Tomczak, J. Liu, B. Z. Tang, D. T. Leong and B. Liu, Biomaterials, 2014, 35, 8669–8677 CrossRef CAS PubMed
.
- Q. He, J. Zhang, J. Shi, Z. Zhu, L. Zhang, W. Bu, L. Guo and Y. Chen, Biomaterials, 2010, 31, 1085–1092 CrossRef CAS PubMed
.
- J. Li, Y. Zhu, W. Li, X. Zhang, Y. Peng and Q. Huang, Biomaterials, 2010, 31, 8410–8418 CrossRef CAS PubMed
.
- M. Liu, X. Zhang, B. Yang, F. Deng, Z. Huang, Y. Yang, Z. Li, X. Zhang and Y. Wei, RSC Adv., 2014, 4, 35137–35143 RSC
.
- Q. Wan, K. Wang, H. Du, H. Huang, M. Liu, F. Deng, Y. Dai, X. Zhang and Y. Wei, Polym. Chem., 2015, 6, 5288–5294 RSC
.
- Z. Wang, B. Xu, L. Zhang, J. Zhang, T. Ma, J. Zhang, X. Fu and W. Tian, Nanoscale, 2013, 5, 2065–2072 RSC
.
- Q. Wan, M. Liu, J. Tian, F. Deng, G. Zeng, Z. Li, K. Wang, Q. Zhang, X. Zhang and Y. Wei, Polym. Chem., 2015, 6, 1786–1792 RSC
.
- D. Ding, K. Li, B. Liu and B. Z. Tang, Acc. Chem. Res., 2013, 46, 2441–2453 CrossRef CAS PubMed
.
- D.-Q. Wu, B. Lu, C. Chang, C.-S. Chen, T. Wang, Y.-Y. Zhang, S.-X. Cheng, X.-J. Jiang, X.-Z. Zhang and R.-X. Zhuo, Biomaterials, 2009, 30, 1363–1371 CrossRef CAS PubMed
.
- M. R. Lorenz, V. Holzapfel, A. Musyanovych, K. Nothelfer, P. Walther, H. Frank, K. Landfester, H. Schrezenmeier and V. Mailänder, Biomaterials, 2006, 27, 2820–2828 CrossRef CAS PubMed
.
- A. V. Nascimento, A. Singh, H. Bousbaa, D. Ferreira, B. Sarmento and M. M. Amiji, Mol. Pharmaceutics, 2014, 11, 3515–3527 CrossRef CAS PubMed
.
- L. Liu, M. L. Fishman, J. Kost and K. B. Hicks, Biomaterials, 2003, 24, 3333–3343 CrossRef CAS PubMed
.
- A. V. Ambade, E. N. Savariar and S. Thayumanavan, Mol. Pharmaceutics, 2005, 2, 264–272 CrossRef CAS PubMed
.
- R. Tong, L. Yala, T. M. Fan and J. Cheng, Biomaterials, 2010, 31, 3043–3053 CrossRef CAS PubMed
.
- B. Sivakumar, R. G. Aswathy, Y. Nagaoka, S. Iwai, K. Venugopal, K. Kato, Y. Yoshida, T. Maekawa and D. N. S. Kumar, RSC Adv., 2013, 3, 20579–20598 RSC
.
- K. Wang, X. Y. Zhang, X. Q. Zhang, B. Yang, Z. Li, Q. S. Zhang, Z. F. Huang and Y. Wei, Macromol. Chem. Phys., 2015, 216, 678–684 CrossRef CAS
.
- K. Wang, X. Y. Zhang, X. Q. Zhang, B. Yang, Z. Li, Q. S. Zhang, Z. F. Huang and Y. Wei, Polym. Chem., 2015, 6, 1360–1366 RSC
.
- Z. Huang, X. Zhang, X. Zhang, B. Yang, Y. Zhang, K. Wang, J. Yuan, L. Tao and Y. Wei, Polym. Chem., 2015, 6, 2133–2138 RSC
.
- K. Li, Z. Zhu, P. Cai, R. Liu, N. Tomczak, D. Ding, J. Liu, W. Qin, Z. Zhao and Y. Hu, Chem. Mater., 2013, 25, 4181–4187 CrossRef CAS
.
- K. Li and B. Liu, Chem. Soc. Rev., 2014, 43, 6570–6597 RSC
.
- Y. Liu, D. Tu, H. Zhu and X. Chen, Chem. Soc. Rev., 2013, 42, 6924–6958 RSC
.
- M. Montalti, L. Prodi, E. Rampazzo and N. Zaccheroni, Chem. Soc. Rev., 2014, 43, 4243–4268 RSC
.
- O. S. Wolfbeis, Chem. Soc. Rev., 2015, 44, 4743–4768 RSC
.
- H. Xu, Q. Li, L. Wang, Y. He, J. Shi, B. Tang and C. Fan, Chem. Soc. Rev., 2014, 43, 2650–2661 RSC
.
- Q. Zhao, C. Huang and F. Li, Chem. Soc. Rev., 2011, 40, 2508–2524 RSC
.
- J. Zhou, Z. Liu and F. Li, Chem. Soc. Rev., 2012, 41, 1323–1349 RSC
.
- X. Xue, S. Jin, C. Zhang, K. Yang, S. Huo, F. Chen, G. Zou and X.-J. Liang, ACS Nano, 2015, 9, 2729–2739 CrossRef CAS PubMed
.
- K. Wang, X. Yuan, Z. Guo, J. Xu and Y. Chen, Carbohydr. Polym., 2014, 102, 699–707 CrossRef CAS PubMed
.
- Q. Wan, C. He, K. Wang, M. Liu, H. Huang, Q. Huang, F. Deng, X. Zhang and Y. Wei, Tetrahedron, 2015, 71, 8791–8797 CrossRef CAS
.
- Z. Zhong, Y. Song, J. F. Engbersen, M. C. Lok, W. E. Hennink and J. Feijen, J. Controlled Release, 2005, 109, 317–329 CrossRef CAS PubMed
.
- X.-Z. Zhang, P. J. Lewis and C.-C. Chu, Biomaterials, 2005, 26, 3299–3309 CrossRef CAS PubMed
.
- B. Lu, X.-D. Xu, X.-Z. Zhang, S.-X. Cheng and R.-X. Zhuo, Biomacromolecules, 2008, 9, 2594–2600 CrossRef CAS PubMed
.
- S. Lorenz, C. P. Hauser, B. Autenrieth, C. K. Weiss, K. Landfester and V. Mailänder, Macromol. Biosci., 2010, 10, 1034–1042 CrossRef CAS PubMed
.
- U. Paiphansiri, J. Dausend, A. Musyanovych, V. Mailänder and K. Landfester, Macromol. Biosci., 2009, 9, 575–584 CrossRef CAS PubMed
.
- U. Paiphansiri, P. Tangboriboonrat and K. Landfester, Macromol. Biosci., 2006, 6, 33–40 CrossRef CAS PubMed
.
- C. K. Weiss, M. R. Lorenz, K. Landfester and V. Mailänder, Macromol. Biosci., 2007, 7, 883–896 CrossRef CAS PubMed
.
- G. Baier, C. Costa, A. Zeller, D. Baumann, C. Sayer, P. H. Araujo, V. Mailänder, A. Musyanovych and K. Landfester, Macromol. Biosci., 2011, 11, 628–638 CrossRef CAS PubMed
.
- B. S. Tucker and B. S. Sumerlin, Polym. Chem., 2014, 5, 1566–1572 RSC
.
- M. Motornov, Y. Roiter, I. Tokarev and S. Minko, Prog. Polym. Sci., 2010, 35, 174–211 CrossRef CAS
.
- O. Onaca, R. Enea, D. W. Hughes and W. Meier, Macromol. Biosci., 2009, 9, 129–139 CrossRef CAS PubMed
.
- S. Chen, S.-X. Cheng and R.-X. Zhuo, Macromol. Biosci., 2011, 11, 576–589 CrossRef CAS PubMed
.
- H. Lee, Y. S. Lee, K. D. Lee and S. Y. Park, Macromol. Biosci., 2011, 11, 1264–1271 CrossRef PubMed
.
- M. M. Yallapu, M. Jaggi and S. C. Chauhan, Macromol. Biosci., 2010, 10, 1141–1151 CrossRef CAS PubMed
.
- R. T. Chacko, J. Ventura, J. Zhuang and S. Thayumanavan, Adv. Drug Delivery Rev., 2012, 64, 836–851 CrossRef CAS PubMed
.
- J. Zhang, S. You, S. Yan, K. Müllen, W. Yang and M. Yin, Chem. Commun., 2014, 50, 7511–7513 RSC
.
- Z. Huang, X. Zhang, X. Zhang, C. Fu, K. Wang, J. Yuan, L. Tao and Y. Wei, Polym. Chem., 2015, 6, 607–612 RSC
.
- W. Qin, D. Ding, J. Liu, W. Z. Yuan, Y. Hu, B. Liu and B. Z. Tang, Adv. Funct. Mater., 2012, 22, 771–779 CrossRef CAS
.
- Q. Wan, J. Tian, M. Liu, G. Zeng, Q. Huang, K. Wang, Q. Zhang, F. Deng, X. Zhang and Y. Wei, Appl. Surf. Sci., 2015, 346, 335–341 CrossRef CAS
.
- N. Zhang, S. R. Samanta, B. M. Rosen and V. Percec, Chem. Rev., 2014, 114, 5848–5958 CrossRef CAS PubMed
.
- A. Bajpai, S. K. Shukla, S. Bhanu and S. Kankane, Prog. Polym. Sci., 2008, 33, 1088–1118 CrossRef CAS
.
- J. K. Oh, R. Drumright, D. J. Siegwart and K. Matyjaszewski, Prog. Polym. Sci., 2008, 33, 448–477 CrossRef CAS
.
- E.-M. Rosenbauer, M. Wagner, A. Musyanovych and K. Landfester, Macromolecules, 2010, 43, 5083–5093 CrossRef CAS
.
- D. Klinger and K. Landfester, Polymer, 2012, 53, 5209–5231 CrossRef CAS
.
- J. Cui, Y. Yan, G. K. Such, K. Liang, C. J. Ochs, A. Postma and F. Caruso, Biomacromolecules, 2012, 13, 2225–2228 CrossRef CAS PubMed
.
- G. K. Such, E. Tjipto, A. Postma, A. P. Johnston and F. Caruso, Nano Lett., 2007, 7, 1706–1710 CrossRef CAS PubMed
.
- D. Roy, J. N. Cambre and B. S. Sumerlin, Prog. Polym. Sci., 2010, 35, 278–301 CrossRef CAS
.
- P. De, M. Li, S. R. Gondi and B. S. Sumerlin, J. Am. Chem. Soc., 2008, 130, 11288–11289 CrossRef CAS PubMed
.
- J.-H. Ryu, R. T. Chacko, S. Jiwpanich, S. Bickerton, R. P. Babu and S. Thayumanavan, J. Am. Chem. Soc., 2010, 132, 17227–17235 CrossRef CAS PubMed
.
- R. Tong and J. Cheng, Macromolecules, 2012, 45, 2225–2232 CrossRef CAS PubMed
.
- M. A. C. Stuart, W. T. Huck, J. Genzer, M. Müller, C. Ober, M. Stamm, G. B. Sukhorukov, I. Szleifer, V. V. Tsukruk and M. Urban, Nat. Mater., 2010, 9, 101–113 CrossRef PubMed
.
- H. Y. Cho, S. E. Averick, E. Paredes, K. Wegner, A. Averick, S. Jurga, S. R. Das and K. Matyjaszewski, Biomacromolecules, 2013, 14, 1262–1267 CrossRef CAS PubMed
.
- E. Wagner, Acc. Chem. Res., 2012, 45, 1005–1013 CrossRef CAS PubMed
.
- H. Yu, Y. Nie, C. Dohmen, Y. Li and E. Wagner, Biomacromolecules, 2011, 12, 2039–2047 CrossRef CAS PubMed
.
- X. Jiang, L. Li, J. Liu, W. E. Hennink and R. Zhuo, Macromol. Biosci., 2012, 12, 703–711 CrossRef CAS PubMed
.
- M. Prabaharan and J. F. Mano, Macromol. Biosci., 2006, 6, 991–1008 CrossRef CAS PubMed
.
- B. He, J. Zeng, Y. Nie, L. Ji, R. Wang, Y. Li, Y. Wu, L. Li, G. Wang and X. Luo, Macromol. Biosci., 2009, 9, 1169–1175 CrossRef CAS PubMed
.
- R. Ravichandran, S. Sundarrajan, J. R. Venugopal, S. Mukherjee and S. Ramakrishna, Macromol. Biosci., 2012, 12, 286–311 CrossRef CAS PubMed
.
- J. K. Oh, D. I. Lee and J. M. Park, Prog. Polym. Sci., 2009, 34, 1261–1282 CrossRef CAS
.
- W. Wu, M. Chen, J. Wang, Q. Zhang, S. Li, Z. Lin and J. Li, RSC Adv., 2014, 4, 30780–30783 RSC
.
- L. Huang, J. Hu, L. Lang, X. Wang, P. Zhang, X. Jing, X. Wang, X. Chen, P. I. Lelkes and A. G. MacDiarmid, Biomaterials, 2007, 28, 1741–1751 CrossRef CAS PubMed
.
- R. Liu, P. Liao, J. Liu and P. Feng, Langmuir, 2011, 27, 3095–3099 CrossRef CAS PubMed
.
- F. Zhan, W. Chen, Z. Wang, W. Lu, R. Cheng, C. Deng, F. Meng, H. Liu and Z. Zhong, Biomacromolecules, 2011, 12, 3612–3620 CrossRef CAS PubMed
.
- N. Rapoport, Prog. Polym. Sci., 2007, 32, 962–990 CrossRef CAS
.
- C. He, S. W. Kim and D. S. Lee, J. Controlled Release, 2008, 127, 189–207 CrossRef CAS PubMed
.
- J.-P. Xu, J. Ji, W.-D. Chen and J.-C. Shen, J. Controlled Release, 2005, 107, 502–512 CrossRef CAS PubMed
.
- F. Meng, Z. Zhong and J. Feijen, Biomacromolecules, 2009, 10, 197–209 CrossRef CAS PubMed
.
- R. Cheng, F. Meng, C. Deng, H.-A. Klok and Z. Zhong, Biomaterials, 2013, 34, 3647–3657 CrossRef CAS PubMed
.
- C. Deng, Y. Jiang, R. Cheng, F. Meng and Z. Zhong, Nano Today, 2012, 7, 467–480 CrossRef CAS
.
- Y.-L. Li, L. Zhu, Z. Liu, R. Cheng, F. Meng, J.-H. Cui, S.-J. Ji and Z. Zhong, Angew. Chem., Int. Ed., 2009, 48, 9914–9918 CrossRef CAS PubMed
.
- H. Sun, B. Guo, R. Cheng, F. Meng, H. Liu and Z. Zhong, Biomaterials, 2009, 30, 6358–6366 CrossRef CAS PubMed
.
- Y. Du, W. Chen, M. Zheng, F. Meng and Z. Zhong, Biomaterials, 2012, 33, 7291–7299 CrossRef CAS PubMed
.
- Y. Xu, F. Meng, R. Cheng and Z. Zhong, Macromol. Biosci., 2009, 9, 1254–1261 CrossRef CAS PubMed
.
- H. Wei, R.-X. Zhuo and X.-Z. Zhang, Prog. Polym. Sci., 2013, 38, 503–535 CrossRef CAS
.
- H. Wei, X.-Z. Zhang, H. Cheng, W.-Q. Chen, S.-X. Cheng and R.-X. Zhuo, J. Controlled Release, 2006, 116, 266–274 CrossRef CAS PubMed
.
- C. Cheng, H. Wei, B.-X. Shi, H. Cheng, C. Li, Z.-W. Gu, S.-X. Cheng, X.-Z. Zhang and R.-X. Zhuo, Biomaterials, 2008, 29, 497–505 CrossRef CAS PubMed
.
- H. Wei, S.-X. Cheng, X.-Z. Zhang and R.-X. Zhuo, Prog. Polym. Sci., 2009, 34, 893–910 CrossRef CAS
.
- Y. Wang, V. Bansal, A. N. Zelikin and F. Caruso, Nano Lett., 2008, 8, 1741–1745 CrossRef CAS PubMed
.
- S. Ganta, H. Devalapally, A. Shahiwala and M. Amiji, J. Controlled Release, 2008, 126, 187–204 CrossRef CAS PubMed
.
- I. Cobo, M. Li, B. S. Sumerlin and S. Perrier, Nat. Mater., 2014, 14, 143–159 CrossRef PubMed
.
- T. Traitel, R. Goldbart and J. Kost, J. Biomater. Sci., Polym. Ed., 2008, 19, 755–767 CrossRef CAS PubMed
.
- R. Liu, Y. Zhang, X. Zhao, A. Agarwal, L. J. Mueller and P. Feng, J. Am. Chem. Soc., 2010, 132, 1500–1501 CrossRef CAS PubMed
.
- R. Liu, Y. Zhang and P. Feng, J. Am. Chem. Soc., 2009, 131, 15128–15129 CrossRef CAS PubMed
.
- M. A. Azagarsamy, P. Sokkalingam and S. Thayumanavan, J. Am. Chem. Soc., 2009, 131, 14184–14185 CrossRef CAS PubMed
.
- A. Klaikherd, C. Nagamani and S. Thayumanavan, J. Am. Chem. Soc., 2009, 131, 4830–4838 CrossRef CAS PubMed
.
- J. Cheng, B. A. Teply, S. Y. Jeong, C. H. Yim, D. Ho, I. Sherifi, S. Jon, O. C. Farokhzad, A. Khademhosseini and R. S. Langer, Pharm. Res., 2006, 23, 557–564 CrossRef CAS PubMed
.
- M. Meyer, C. Dohmen, A. Philipp, D. Kiener, G. Maiwald, C. Scheu, M. Ogris and E. Wagner, Mol. Pharmaceutics, 2009, 6, 752–762 CrossRef CAS PubMed
.
- P. M. Klein and E. Wagner, Antioxid. Redox Signaling, 2014, 21, 804–817 CrossRef CAS PubMed
.
- J. Shi, L. Liu, X. Sun, S. Cao and J. F. Mano, Macromol. Biosci., 2008, 8, 260–267 CrossRef CAS PubMed
.
- B. Liu, H. Chen, X. Li, C. Zhao, Y. Liu, L. Zhu, H. Deng, J. Li, G. Li and F. Guo, RSC Adv., 2014, 4, 48943–48951 RSC
.
- D. Li, Z. Liang, J. Chen, J. Yu and R. Xu, Dalton Trans., 2013, 42, 9877–9883 RSC
.
- X. Zhang, K. Wang, M. Liu, X. Zhang, L. Tao, Y. Chen and Y. Wei, Nanoscale, 2015, 7, 11486–11508 RSC
.
- M. Liu, G. Zeng, K. Wang, Q. Wan, L. Tao, X. Zhang and Y. Wei, Nanoscale, 2016, 8, 16819–16840 RSC
.
- Q. Wan, M. Liu, J. Tian, F. Deng, G. Zeng, Z. Li, K. Wang, Q. Zhang, X. Zhang and Y. Wei, Polym. Chem., 2015, 6, 1786–1792 RSC
.
- Q. Wan, L. Mao, M. Liu, K. Wang, G. Zeng, D. Xu, H. Huang, X. Zhang and Y. Wei, Polym. Chem., 2015, 6, 7211–7218 RSC
.
- Q. Wan, K. Wang, C. He, M. Liu, G. Zeng, H. Huang, F. Deng, X. Zhang and Y. Wei, Polym. Chem., 2015, 6, 8214–8221 RSC
.
- J. Tian, H. Zhang, M. Liu, F. Deng, H. Huang, Q. Wan, Z. Li, K. Wang, X. He and X. Zhang, Appl. Surf. Sci., 2015, 357, 1996–2003 CrossRef CAS
.
- X. Zhang, Q. Huang, M. Liu, J. Tian, G. Zeng, Z. Li, K. Wang, Q. Zhang, Q. Wan and F. Deng, Appl. Surf. Sci., 2015, 343, 19–27 CrossRef CAS
.
- X. Zhang, G. Zeng, J. Tian, Q. Wan, Q. Huang, K. Wang, Q. Zhang, M. Liu, F. Deng and Y. Wei, Appl. Surf. Sci., 2015, 351, 425–432 CrossRef CAS
.
- C. Heng, M. Liu, P. Wang, K. Wang, X. Zheng, D. Fan, J. Hui, X. Zhang and Y. Wei, Chem. Eng. J., 2016, 296, 268–276 CrossRef CAS
.
- H. Huang, M. Liu, S. Luo, K. Wang, Q. Wan, F. Deng, D. Xu, X. Zhang and Y. Wei, Chem. Eng. J., 2016, 304, 149–155 CrossRef CAS
.
- C. Muniesa, V. Vicente, M. Quesada, S. Sáez-Atiénzar, J. R. Blesa, I. Abasolo, Y. Fernández and P. Botella, RSC Adv., 2013, 3, 15121–15131 RSC
.
- F. Meng, W. E. Hennink and Z. Zhong, Biomaterials, 2009, 30, 2180–2198 CrossRef CAS PubMed
.
- R. Cheng, F. Feng, F. Meng, C. Deng, J. Feijen and Z. Zhong, J. Controlled Release, 2011, 152, 2–12 CrossRef CAS PubMed
.
- H. Sun, B. Guo, X. Li, R. Cheng, F. Meng, H. Liu and Z. Zhong, Biomacromolecules, 2010, 11, 848–854 CrossRef CAS PubMed
.
- C. Lin, Z. Zhong, M. C. Lok, X. Jiang, W. E. Hennink, J. Feijen and J. F. Engbersen, J. Controlled Release, 2006, 116, 130–137 CrossRef CAS PubMed
.
- Y. Wu, W. Chen, F. Meng, Z. Wang, R. Cheng, C. Deng, H. Liu and Z. Zhong, J. Controlled Release, 2012, 164, 338–345 CrossRef CAS PubMed
.
- A. N. Zelikin, J. F. Quinn and F. Caruso, Biomacromolecules, 2006, 7, 27–30 CrossRef CAS PubMed
.
- A. P. Bapat, J. G. Ray, D. A. Savin and B. S. Sumerlin, Macromolecules, 2013, 46, 2188–2198 CrossRef CAS
.
- B. S. Tucker, S. G. Getchell, M. R. Hill and B. S. Sumerlin, Polym. Chem., 2015, 6, 4258–4263 RSC
.
- R. Liu, X. Zhao, T. Wu and P. Feng, J. Am. Chem. Soc., 2008, 130, 14418–14419 CrossRef CAS PubMed
.
- Z. Zhang, L. Yin, C. Tu, Z. Song, Y. Zhang, Y. Xu, R. Tong, Q. Zhou, J. Ren and J. Cheng, ACS Macro Lett., 2013, 2, 40–44 CrossRef CAS PubMed
.
- H. Wang, L. Tang, C. Tu, Z. Song, Q. Yin, L. Yin, Z. Zhang and J. Cheng, Biomacromolecules, 2013, 14, 3706–3712 CrossRef CAS PubMed
.
- N. Zheng, Z. Song, Y. Liu, R. Zhang, R. Zhang, C. Yao, F. M. Uckun, L. Yin and J. Cheng, J. Controlled Release, 2015, 205, 231–239 CrossRef CAS PubMed
.
- K. Cai, J. Yen, Q. Yin, Y. Liu, Z. Song, S. Lezmi, Y. Zhang, X. Yang, W. G. Helferich and J. Cheng, Biomater. Sci., 2015, 3, 1061–1065 RSC
.
- J. S. Lee, X. Deng, P. Han and J. Cheng, Macromol. Biosci., 2015, 15, 1314–1322 CrossRef CAS PubMed
.
- J. Kamada, K. Koynov, C. Corten, A. Juhari, J. A. Yoon, M. W. Urban, A. C. Balazs and K. Matyjaszewski, Macromolecules, 2010, 43, 4133–4139 CrossRef CAS
.
- X. Song, L. Lin, M. Rong, Y. Wang, Z. Xie and X. Chen, Carbon, 2014, 80, 174–182 CrossRef CAS
.
- Y. X. Gao, J. R. Lu, J. Wu, J. Hu and Y. Ju, RSC Adv., 2014, 4, 63539–63543 RSC
.
- Y. Wang, H. Wang, G. Liu, X. Liu, Q. Jin and J. Ji, Macromol. Biosci., 2013, 13, 1084–1091 CrossRef CAS PubMed
.
- T. Gulin-Sarfraz, J. Sarfraz, D. Ş. Karaman, J. Zhang, C. Oetken-Lindholm, A. Duchanoy, J. M. Rosenholm and D. Abankwa, RSC Adv., 2014, 4, 16429–16437 RSC
.
- P. Zhang, F. Deng, Y. Peng, H. Chen, Y. Gao and H. Li, RSC Adv., 2014, 4, 47361–47367 RSC
.
- N. Ashwinkumar, S. Maya and R. Jayakumar, RSC Adv., 2014, 4, 49547–49555 RSC
.
- H. C. Kang, H.-J. Kang and Y. H. Bae, Biomaterials, 2011, 32, 1193–1203 CrossRef CAS PubMed
.
- H. S. Hwang, H. C. Kang and Y. H. Bae, Biomacromolecules, 2013, 14, 548–556 CrossRef CAS PubMed
.
- L. Tian, H. C. Kang and Y. H. Bae, Biomacromolecules, 2013, 14, 2570–2581 CrossRef CAS PubMed
.
- N. Ma, Y. Li, H. Xu, Z. Wang and X. Zhang, J. Am. Chem. Soc., 2010, 132, 442–443 CrossRef CAS PubMed
.
- H. Ren, Y. Wu, N. Ma, H. Xu and X. Zhang, Soft Matter, 2012, 8, 1460–1466 RSC
.
- H. Xu, W. Cao and X. Zhang, Acc. Chem. Res., 2013, 46, 1647–1658 CrossRef CAS PubMed
.
- W. Cao, Y. Gu, M. Meineck, T. Li and H. Xu, J. Am. Chem. Soc., 2014, 136, 5132–5137 CrossRef CAS PubMed
.
- W. Ong, Y. Yang, A. C. Cruciano and R. L. McCarley, J. Am. Chem. Soc., 2008, 130, 14739–14744 CrossRef CAS PubMed
.
- M. Hu, J. Zhu and L. Qiu, RSC Adv., 2014, 4, 64151–64161 RSC
.
- R. L. McCarley, Annu. Rev. Anal. Chem., 2012, 5, 391–411 CrossRef CAS PubMed
.
- Q. Zhang, S. Aleksanian, S. M. Noh and J. K. Oh, Polym. Chem., 2013, 4, 351–359 RSC
.
- S. Aleksanian, B. Khorsand, R. Schmidt and J. K. Oh, Polym. Chem., 2012, 3, 2138–2147 RSC
.
- B. Khorsand, G. Lapointe, C. Brett and J. K. Oh, Biomacromolecules, 2013, 14, 2103–2111 CrossRef CAS PubMed
.
- B. Khorsand Sourkohi, A. Cunningham, Q. Zhang and J. K. Oh, Biomacromolecules, 2011, 12, 3819–3825 CrossRef CAS PubMed
.
- N. R. Ko and J. K. Oh, Biomacromolecules, 2014, 15, 3180–3189 CrossRef CAS PubMed
.
- B. Mandal and B. Basu, RSC Adv., 2014, 4, 13854–13881 RSC
.
- D. Maciel, P. Figueira, S. Xiao, D. Hu, X. Shi, J. o. Rodrigues, H. Tomaás and Y. Li, Biomacromolecules, 2013, 14, 3140–3146 CrossRef CAS PubMed
.
- A. Musyanovych and K. Landfester, Macromol. Biosci., 2014, 14, 458–477 CrossRef CAS PubMed
.
- A. L. Becker, N. I. Orlotti, M. Folini, F. Cavalieri, A. N. Zelikin, A. P. Johnston, N. Zaffaroni and F. Caruso, ACS Nano, 2011, 5, 1335–1344 CrossRef CAS PubMed
.
- Q. Liu, B. Yu, W. Ye and F. Zhou, Macromol. Biosci., 2011, 11, 1227–1234 CrossRef CAS PubMed
.
- Q. Yan, J. Yuan, Z. Cai, Y. Xin, Y. Kang and Y. Yin, J. Am. Chem. Soc., 2010, 132, 9268–9270 CrossRef CAS PubMed
.
- Q. Yan, R. Zhou, C. Fu, H. Zhang, Y. Yin and J. Yuan, Angew. Chem., 2011, 123, 5025–5029 CrossRef
.
- E. Kim, D. Kim, H. Jung, J. Lee, S. Paul, N. Selvapalam, Y. Yang, N. Lim, C. G. Park and K. Kim, Angew. Chem., 2010, 122, 4507–4510 CrossRef
.
- Z. Hong, P. Zhang, C. He, X. Qiu, A. Liu, L. Chen, X. Chen and X. Jing, Biomaterials, 2005, 26, 6296–6304 CrossRef CAS PubMed
.
- H. Tian, Z. Tang, X. Zhuang, X. Chen and X. Jing, Prog. Polym. Sci., 2012, 37, 237–280 CrossRef CAS
.
- X. He, M. Ding, J. Li, H. Tan, Q. Fu and L. Li, RSC Adv., 2014, 4, 24736–24746 RSC
.
- X. Zhang, Y. Li, X. Chen, X. Wang, X. Xu, Q. Liang, J. Hu and X. Jing, Biomaterials, 2005, 26, 2121–2128 CrossRef CAS PubMed
.
- Z. Hong, X. Qiu, J. Sun, M. Deng, X. Chen and X. Jing, Polymer, 2004, 45, 6699–6706 CrossRef CAS
.
- H. Tian, C. Deng, H. Lin, J. Sun, M. Deng, X. Chen and X. Jing, Biomaterials, 2005, 26, 4209–4217 CrossRef CAS PubMed
.
- L. Piao, Z. Dai, M. Deng, X. Chen and X. Jing, Polymer, 2003, 44, 2025–2031 CrossRef CAS
.
- P. Zhang, Z. Hong, T. Yu, X. Chen and X. Jing, Biomaterials, 2009, 30, 58–70 CrossRef CAS PubMed
.
- L. Gao, Q. Luo, Y. Wang, H. Du, X. Li, Z. Shen and W. Zhu, RSC Adv., 2014, 4, 4177–4180 RSC
.
- A. Zhang, Z. Zhang, F. Shi, C. Xiao, J. Ding, X. Zhuang, C. He, L. Chen and X. Chen, Macromol. Biosci., 2013, 13, 1249–1258 CrossRef CAS PubMed
.
- J. Ding, C. Xiao, C. He, M. Li, D. Li, X. Zhuang and X. Chen, Nanotechnology, 2011, 22, 494012 CrossRef PubMed
.
- C. Fu, L. Lin, H. Shi, D. Zheng, W. Wang, S. Gao, Y. Zhao, H. Tian, X. Zhu and X. Chen, Biomaterials, 2012, 33, 4589–4596 CrossRef CAS PubMed
.
- H. Tian, W. Xiong, J. Wei, Y. Wang, X. Chen, X. Jing and Q. Zhu, Biomaterials, 2007, 28, 2899–2907 CrossRef CAS PubMed
.
- J. Ding, F. Shi, C. Xiao, L. Lin, L. Chen, C. He, X. Zhuang and X. Chen, Polym. Chem., 2011, 2, 2857–2864 RSC
.
- A. Lavasanifar, J. Samuel and G. S. Kwon, Adv. Drug Delivery Rev., 2002, 54, 169–190 CrossRef CAS PubMed
.
- S. Chen, X.-Z. Zhang, S.-X. Cheng, R.-X. Zhuo and Z.-W. Gu, Biomacromolecules, 2008, 9, 2578–2585 CrossRef CAS PubMed
.
- S. Li, J. Han and C. Gao, Polym. Chem., 2013, 4, 1774–1787 RSC
.
- C. Gao and D. Yan, Prog. Polym. Sci., 2004, 29, 183–275 CrossRef CAS
.
- Y. Zhou, W. Huang, J. Liu, X. Zhu and D. Yan, Adv. Mater., 2010, 22, 4567–4590 CrossRef CAS PubMed
.
- H. Jin, W. Huang, X. Zhu, Y. Zhou and D. Yan, Chem. Soc. Rev., 2012, 41, 5986–5997 RSC
.
- J. Liu, Y. Pang, W. Huang, X. Zhu, Y. Zhou and D. Yan, Biomaterials, 2010, 31, 1334–1341 CrossRef CAS PubMed
.
- D. Wang, T. Zhao, X. Zhu, D. Yan and W. Wang, Chem. Soc. Rev., 2015, 44, 4023–4071 RSC
.
- J. Liu, H. Duong, M. R. Whittaker, T. P. Davis and C. Boyer, Macromol. Rapid Commun., 2012, 33, 760–766 CrossRef CAS PubMed
.
- W. Godbey, K. K. Wu and A. G. Mikos, J. Controlled Release, 1999, 60, 149–160 CrossRef CAS PubMed
.
- W. Godbey, K. K. Wu and A. G. Mikos, J. Controlled Release, 1999, 60, 149–160 CrossRef CAS PubMed
.
- C. Liu, P. Zhang, X. Zhai, F. Tian, W. Li, J. Yang, Y. Liu, H. Wang, W. Wang and W. Liu, Biomaterials, 2012, 33, 3604–3613 CrossRef CAS PubMed
.
- E. R. Gillies and J. M. Frechet, Drug Discovery Today, 2005, 10, 35–43 CrossRef CAS PubMed
.
- D. A. Tomalia and J. M. Fréchet, J. Polym. Sci., Part A: Polym. Chem., 2002, 40, 2719–2728 CrossRef CAS
.
- S. Stiriba, H. Frey and R. Haag, Angew. Chem., Int. Ed., 2002, 41, 1329–1334 CrossRef CAS PubMed
.
- C.-M. Dong and G. Liu, Polym. Chem., 2013, 4, 46–52 RSC
.
- R. Esfand and D. A. Tomalia, Drug Discovery Today, 2001, 6, 427–436 CrossRef CAS PubMed
.
- E. Arce, P. M. Nieto, V. Díaz, R. G. Castro, A. Bernad and J. Rojo, Bioconjugate Chem., 2003, 14, 817–823 CrossRef CAS PubMed
.
- J. Liu, Y. Pang, W. Huang, X. Huang, L. Meng, X. Zhu, Y. Zhou and D. Yan, Biomacromolecules, 2011, 12, 1567–1577 CrossRef CAS PubMed
.
- H. Mok and T. G. Park, Macromol. Biosci., 2012, 12, 40–48 CrossRef CAS
.
- J. Liu, W. Yang, L. Tao, D. Li, C. Boyer and T. P. Davis, J. Polym. Sci., Part A: Polym. Chem., 2010, 48, 425–433 CrossRef CAS
.
- S. H. Lee, D. R. Dreyer, J. An, A. Velamakanni, R. D. Piner, S. Park, Y. Zhu, S. O. Kim, C. W. Bielawski and R. S. Ruoff, Macromol. Rapid Commun., 2010, 31, 281–288 CrossRef CAS PubMed
.
- K. Hu, D. D. Kulkarni, I. Choi and V. V. Tsukruk, Prog. Polym. Sci., 2014, 39, 1934–1972 CrossRef CAS
.
- T. Kuilla, S. Bhadra, D. Yao, N. H. Kim, S. Bose and J. H. Lee, Prog. Polym. Sci., 2010, 35, 1350–1375 CrossRef CAS
.
- S. Stankovich, D. A. Dikin, G. H. Dommett, K. M. Kohlhaas, E. J. Zimney, E. A. Stach, R. D. Piner, S. T. Nguyen and R. S. Ruoff, Nature, 2006, 442, 282–286 CrossRef CAS PubMed
.
- W. S. Hummers Jr and R. E. Offeman, J. Am. Chem. Soc., 1958, 80, 1339 CrossRef
.
- S. Stankovich, D. A. Dikin, R. D. Piner, K. A. Kohlhaas, A. Kleinhammes, Y. Jia, Y. Wu, S. T. Nguyen and R. S. Ruoff, Carbon, 2007, 45, 1558–1565 CrossRef CAS
.
- F. Bonaccorso, L. Colombo, G. Yu, M. Stoller, V. Tozzini, A. C. Ferrari, R. S. Ruoff and V. Pellegrini, Science, 2015, 347, 1246501 CrossRef PubMed
.
- Z. Li, J. C. Barnes, A. Bosoy, J. F. Stoddart and J. I. Zink, Chem. Soc. Rev., 2012, 41, 2590–2605 RSC
.
- L. Tan, H.-X. Wu, M.-Y. Yang, C.-J. Liu and R.-X. Zhuo, RSC Adv., 2015, 5, 10393–10399 RSC
.
- Z. Tao, RSC Adv., 2014, 4, 18961–18980 RSC
.
- J. L. Vivero-Escoto and D. L. Vega, RSC Adv., 2014, 4, 14400–14407 RSC
.
- B. Chang, D. Chen, Y. Wang, Y. Chen, Y. Jiao, X. Sha and W. Yang, Chem. Mater., 2013, 25, 574–585 CrossRef CAS
.
- Y. Wen and J. K. Oh, RSC Adv., 2014, 4, 229–237 RSC
.
- B. Gyarmati, Á. Némethy and A. Szilágyi, RSC Adv., 2014, 4, 8764–8771 RSC
.
- B. Gyarmati, B. Vajna, Á. Némethy, K. Lászlá and A. Szilágyi, Macromol. Biosci., 2013, 13, 633–640 CrossRef CAS PubMed
.
- J. Shi, N. M. Alves and J. F. Mano, Macromol. Biosci., 2006, 6, 358–363 CrossRef CAS PubMed
.
- W. Cheng, J. N. Kumar, Y. Zhang and Y. Liu, Macromol. Biosci., 2014, 14, 347–358 CrossRef CAS PubMed
.
- L. Ren, L. He, T. Sun, X. Dong, Y. Chen, J. Huang and C. Wang, Macromol. Biosci., 2009, 9, 902–910 CrossRef CAS PubMed
.
- N. Chan, B. Khorsand, S. Aleksanian and J. K. Oh, Chem. Commun., 2013, 49, 7534–7536 RSC
.
- N. Chan, S. Y. An and J. K. Oh, Polym. Chem., 2014, 5, 1637–1649 RSC
.
- H. Tan, L. Zhao, W. Liu, L. Ren, S. Xu, L. Chen and W. Li, RSC Adv., 2014, 4, 60413–60420 RSC
.
- W. Yuan, H. Zou, W. Guo, T. Shen and J. Ren, Polym. Chem., 2013, 4, 2658–2661 RSC
.
- S. Jun, F. Zeng, H. Jian and S. Wu, Biomacromolecules, 2013, 14, 728–736 CrossRef PubMed
.
- X. Hu, H. Li, S. Luo, T. Liu, Y. Jiang and S. Liu, Polym. Chem., 2013, 4, 695–706 RSC
.
- L.-A. Tziveleka, P. Bilalis, A. Chatzipavlidis, N. Boukos and G. Kordas, Macromol. Biosci., 2014, 14, 131–141 CrossRef CAS PubMed
.
- J. A. Nam, A. Al-Nahain, S. Hong, K. D. Lee, H. Lee and S. Y. Park, Macromol. Biosci., 2011, 11, 1594–1602 CAS
.
- Y. Guan, H.-B. Zhao, L.-X. Yu, S.-C. Chen and Y.-Z. Wang, RSC Adv., 2014, 4, 4955–4959 RSC
.
- X. Huang, X. Jiang, Q. Yang, Y. Chu, G. Zhang, B. Yang and R. Zhuo, J. Mater. Chem. B, 2013, 1, 1860–1868 RSC
.
- Y. Ding, Y. Yi, H. Xu, Z. Wang and X. Zhang, Chem. Commun., 2014, 50, 2585–2588 RSC
.
- L. Zeng, Y. Li, T. Li, W. Cao, Y. Yi, W. Geng, Z. Sun and H. Xu, Chem. – Asian J., 2014, 9, 2295–2302 CrossRef CAS PubMed
.
- P. Han, N. Ma, H. Ren, H. Xu, Z. Li, Z. Wang and X. Zhang, Langmuir, 2010, 26, 14414–14418 CrossRef CAS PubMed
.
- N. Ma, Y. Li, H. Ren, H. Xu, Z. Li and X. Zhang, Polym. Chem., 2010, 1, 1609–1614 RSC
.
- N. Ma, H. Xu, L. An, J. Li, Z. Sun and X. Zhang, Langmuir, 2011, 27, 5874–5878 CrossRef CAS PubMed
.
- P. Han, S. Li, W. Cao, Y. Li, Z. Sun, Z. Wang and H. Xu, J. Mater. Chem. B, 2013, 1, 740–743 RSC
.
- L. Wang, W. Cao, Y. Yi and H. Xu, Langmuir, 2014, 30, 5628–5636 CrossRef CAS PubMed
.
|
This journal is © the Partner Organisations 2017 |