DOI:
10.1039/C6QM00074F
(Research Article)
Mater. Chem. Front., 2017,
1, 132-145
A novel fluorophore–spacer–receptor to conjugate MWNTs and ferrite nanoparticles to design an ultra-thin shield to screen electromagnetic radiation†
Received
31st May 2016
, Accepted 12th August 2016
First published on 22nd August 2016
Abstract
A novel fluorophore–spacer–receptor has been designed with hydrazono methyl phenol as the receptor, anthracene as the fluorophore and imine (C
N) groups as the spacer. This newly designed fluorophoric system has a receptor that can bind with ferrites and a fluorophore core that can conjugate non-covalently with multiwalled carbon nanotubes (MWNTs) via π–π conjugation. The hybrid nanoparticles were thoroughly characterized using Raman, UV-vis and fluorescence spectroscopy. This unique hybrid is further explored as a novel material to screen electromagnetic (EM) radiation. By precisely localizing these hybrids in a given phase of an immiscible co-continuous blend, unique microstructures can be constructed. Herein, blends of polyvinylidene fluoride (PVDF) and polycarbonate (PC) were chosen as a model system. The hybrid nanoparticles were selectively localized in the PVDF phase owing to its higher polarity and were systematically characterized by electron microscopic and solution–dissolution techniques. The hybrid nanoparticles that were designed to shield from the incident EM radiation resulted in >99.99% attenuation, dominated mostly by absorption. This non-covalent approach of conjugating MWNTs with ferrites, aided by the fluorophoric system, was noted to be a more effective way to improve the properties (both bulk electrical conductivity and structural) than direct physical mixing/covalent conjugation approaches. In order to further enhance the shielding effectiveness (SE), a layer-by-layer architecture was constructed essentially with outer layers containing PC/PVDF blends with a MWNT–ferrite hybrid and the inner layers consisting of PC/PVDF blends with only MWNTs. An ultra-thin shield of 0.90 mm showed >99.9999% attenuation suggesting new pathways for designing lightweight, flexible EMI shielding materials.
Introduction
In recent times, the extensive use of high frequency electronic equipment in commercial, scientific and military applications has significantly improved our lives. Despite the advancement in microwave technology, inadequacies allied with electromagnetic interference and radiation pollution turn into severe issues for communication and health. Therefore, it becomes a prime requisite to develop electromagnetic shielding materials to reduce stray electromagnetic waves.1–5
Generally, shielding materials have the ability to dissipate electromagnetic waves and transform them into heat by different mechanisms. Metals are the most common EM shielding material, but an ideal EM shielding material should be lightweight, corrosion resistant and effective within a very broad band with multifunctionality. So, carbon based materials such as carbon fibres (CFs), carbon nanotubes (CNTs), and graphene oxide (GO) have been attractive in this domain due to their conducting nature and their ability to embed in a host polymeric matrix to design lightweight, flexible and easy to process shielding materials.6–16 However, their mechanism of EM shielding is generally by reflection, which limits their application. In this framework, designing microwave absorbers has received burgeoning research interest.17–20
EM radiation is a harmonized proliferation of electric and magnetic fields, which are perpendicular to each other. In this context, to circumvent the interference, the shielding material should contain both electric and magnetic dipoles which can interact with electric and magnetic vector components of the incident EM wave. Though some materials possess very high dielectric constant or very high magnetic permeability, the use of such materials is restricted by their high loading concentration. In recent studies, it is observed that conducting nanoparticles (like CNTs, GO) decorated with ferromagnetic particles (like Fe, Co, Ni, Fe3O4) are considered as promising alternatives which allow rather relatively lower filler loading.21–27 The inter-tube van der Waals force of attraction can play a significant role in efficient dispersion of these conducting nanofillers in a given matrix. In this context, altering the surface chemistry can assist in de-bundling the CNTs. However, altering it by covalent modification greatly affects the π-conjugation of CNTs, resulting in significant deterioration of the overall electronic properties of CNTs. In contrast, non-covalent functionalization retains the structural integrity of the nanotubes.28–32
A crucial step in developing high performance, lightweight EM shielding materials is to understand the parameters that govern the shielding, such as conductivity, permittivity and permeability. In light of existing challenges in designing efficient lightweight EM shielding materials, we employed a unique approach by conjugating multiwalled carbon nanotubes and ferrites using a novel fluorophoric system containing a metal receptor, a spacer and a fluorophore core. A co-continuous PC/PVDF blend was chosen as a model system to disperse the hybrid particles and design EM shielding materials. The selective filling of MWNTs in one of the phases of an immiscible blend increases the electrical conductivity significantly. However, after the incorporation of ferromagnetic nanoparticles, often, the overall properties of the blends are altered dramatically. Hence, we employed three different techniques to disperse the hybrid nanomaterials. Firstly, by physical mixing, where we directly added both the nanoparticles (ferromagnetic and conducting) during blending. It was observed that this uneven distribution of hybrid nanoparticles deteriorated the overall electrical conductivity as well as the shielding efficiency. Secondly, we covalently conjugated the conductive and the ferromagnetic nanoparticles by introducing appropriate functional groups on its surface. Although this resulted in slightly better properties in terms of bulk electrical conductivity of the blends, it was far below that by just adding an equivalent amount of conductive particles. This manifests two things: the disruption of the charge transport through the MWNT network due to defect sites created in functionalizing them and secondly, the ferromagnetic nanoparticles may have aggregated due to van der Waals force of attraction. Thirdly, we adopted a non-covalent strategy to conjugate different nanoparticles. The non-covalent attachment was successfully done here by synthesizing a novel fluorophoric system (AHB) with two receptor sites that can bind co-ordinately with Fe3O4 nanoparticles and the fluorophore core can conjugate with MWNTs via π–π stacking. The mechanical, rheological, electrical and EMI shielding properties were assessed here for all of the blends for a clear understanding of the structure–property correlation.
Finally, we developed a facile technique of layer-by-layer (LbL) assembly by compression molding a stack of different nanocomposites to create a thin (0.90 mm) multilayer structure for highly efficient EM shielding application. The rationale behind selecting different films containing either the conductive particles or the hybrid was to optimize the shielding performance. Our system comprises 3 thin layers with a potential reflective layer (conducting blends with modified MWNTs) sandwiched between two potential absorbing layers (blends containing hybrid particles). It is envisaged that most of the incident radiation will be absorbed by the top and the bottom layers and that which penetrated would reflect back from the inner conducting layers and finally be scavenged by the outer absorbing layers. This engineered multilayered sandwich structure resulted in an exceptional shielding effectiveness of −60 dB for such a thin shield of 0.9 mm.
Experimental section
Materials
Pristine MWNTs (length 1.5 μm and diameter 9.5 nm) were procured from Nanocyl SA (Belgium). Polycarbonate (Lexan-143R) was obtained from Sabic (MFI 11 g/10 min). Polyvinylidine fluoride (KYNAR-761) was procured from Arkema (MW 440
000 g mol−1). FeCl3, FeSO4·7H2O, N,N′-dicyclohexylcarbodiimide (DCC), 4-dimethylaminopyridine (DMAP), 9-anthracenecarboxaldehyde, 3-hydroxybenzaldehyde, (3-aminopropyl)triethoxysilane, and hydrazine hydrate were procured from Sigma Aldrich. Analytical grades of chloroform, 28% ammonia solution, ethanol, N,N-dimethylformamide and tetrahydrofuran were obtained from commercial sources.
Synthesis of Fe3O4 nanoparticles
Fe3O4 nanoparticles were synthesized via co-precipitation of Fe2+ and Fe3+ by a base.33 Initially, FeSO4·7H2O (1 mmol) and FeCl3 (2 mmol) were dissolved in 40 ml of DI water at 80 °C under vigorous stirring for 10 min under a nitrogen atmosphere. Later, 4 ml of 25% ammonia solution was added into the reaction mixture and stirring was continued for another 20 min. The mixture was then cooled to room temperature and the resulting particles were collected by magnetic decantation followed by repeated washing with DI water and finally dried at 80 °C (see Scheme 1).
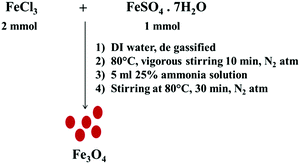 |
| Scheme 1 Co-precipitation synthetic pathway to design Fe3O4 nanoparticles. | |
Synthesis of acid functionalized MWNTs
Carboxylic acid functionalized MWNTs (MWNT–COOH) were obtained by concentrated nitric acid treatment. Initially MWNTs (100 mg) were sonicated in a mixture of 90 ml conc. HNO3 and 10 ml DI water for 1 h. Subsequently, the mixture was kept under reflux with vigorous stirring at 80 °C for 24 h. The mixture was then cooled and poured into 2 l of DI water, collected by filtration and finally dried at 80 °C (see Scheme 2).
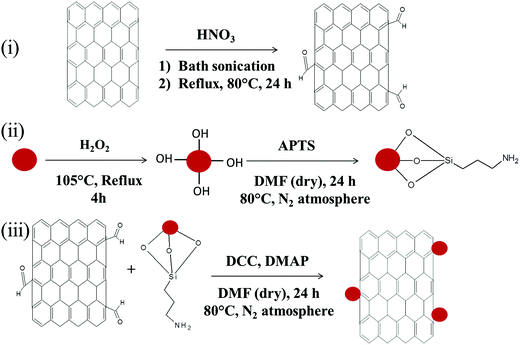 |
| Scheme 2 (i) Synthesis of acid functionalization of MWNTs, (ii) synthesis of amine terminated Fe3O4 nanoparticles, and (iii) covalent conjugation of acid functionalised MWNTs (MWNT–COOH) and amine terminated Fe3O4 nanoparticles (Fe3O4–NH2). | |
Synthesis of amine terminated Fe3O4 nanoparticles
Amine terminated Fe3O4 (Fe3O4–NH2) nanoparticles were synthesized via APTS treatment. Initially Fe3O4 nanoparticles were hydrolysed by dispersing in H2O2via bath sonication for 2 h. Then the dispersed solution was refluxed for 4 h at 105 °C. Later these hydroxylated Fe3O4 nanoparticles (100 mg) were re-dispersed in dry DMF (100 ml) by sonication. Subsequently, APTS was added into the mixture and vigorously stirred at 105 °C under a nitrogen atmosphere for 24 h. The mixture was cooled at room temperature and the resulting particles were collected by centrifugation followed by repeated washing with DMF and ethanol. Finally the resultant particles were dried at 80 °C in a vacuum oven (see Scheme 2).
Synthesis of MWNT–Fe3O4 hybrid nanoparticles
Carboxylic acid functionalized MWNTs (MWNT–COOH) and amine terminated Fe3O4 nanoparticles (Fe3O4–NH2) were covalently conjugated by a condensation reaction. Initially, acid functionalized MWNTs and amine terminated Fe3O4 nanoparticles (1
:
1) were sonicated together for 30 min at room temperature. Later, DCC and DMAP were added into the mixture and vigorously stirred for 24 h at 80 °C temperature in a nitrogen atmosphere to yield MWNT–Fe3O4 (see Scheme 2). The final product contains 46% MWNTs and 44% Fe3O4 nanoparticles (see Fig. S1, ESI,† for TGA analysis).
Synthesis of anthracene-9-(methylene)hydrazono(methyl)phenol (AHB)
In this study AHB was synthesized by a two step condensation reaction. To a 100 ml flask, 9-anthracenecarboxaldehyde (2.5 mmol) was dissolved in 30 ml ethanol. The mixture was then stirred at 45 °C for 1 h and subsequently, 12.5 ml of hydrazine hydrate was added into the mixture dropwise. Then the total mixture was heated to reflux in an air bath for 6 h. Finally the mixture was cooled and poured into 200 ml ice cold DI water and stirred vigorously, and a whitish yellow precipitate was formed. The resulting precipitate (AH) was filtered and dried under an IR lamp (see Scheme 3).
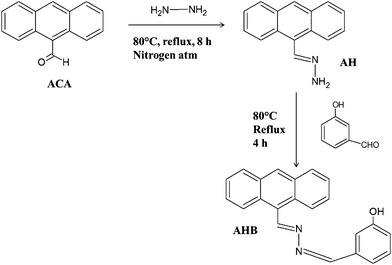 |
| Scheme 3 Synthetic procedure of AHB molecules. | |
In the second step AH (1 mmol) was dissolved in 20 ml ethanol and stirred vigorously at 45 °C for 1 h. An excess of 3-hydroxybenzaldehyde was then added dropwise into the solution and the mixture was kept in an air bath to reflux for 6 h. After that the reaction mixture was cooled and poured into 200 ml ice cold DI water and stirred vigorously, and a deep yellow precipitate was formed. The resulting precipitate (AHB) was filtered and dried under an IR lamp (see Scheme 3).
Preparation of AHB modified MWNTs
The synthesized anthracene based metal receptor has strong interaction with carbon based materials due to its extended π-conjugation of the antharacene moiety. So, here AHB molecules were non-covalently attached on the surface of MWNTs. Typically a 2
:
1 weight ratio of MWNTs and AHB was mixed in ethanol and sonicated for 2 h at room temperature. After vigorous stirring overnight the total mixture was kept for another 24 h for ageing. Finally the mixture was centrifuged and washed several times with ethanol to remove unbound AHB. The complete removal of unbound AHB was guided by the UV-Vis spectroscopic technique. The final product contains 25% (wt%) AHB molecules (see Fig. S1, ESI,† for TGA analysis) (Scheme 4).
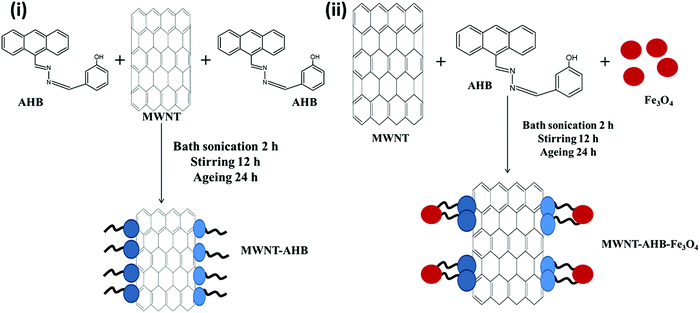 |
| Scheme 4 Synthetic pathway of a non-covalent technique: (i) fabrication of MWNT–AHB and (ii) fabrication of MNT–AHB–Fe3O4 hybrid nanomaterials. | |
Preparation of MWNT–AHB–Fe3O4 hybrid nanomaterials
Fe3O4 nanoparticles are non-covalently attached with MWNTs by this approach. Typically MWNTs, AHB and Fe3O4 are mixed in ethanol (2
:
1
:
2) and sonicated for 3 h at room temperature. After vigorous stirring overnight the mixture was kept for another 24 h for ageing. It is expected that the receptor of AHB binds with the Fe3O4 nanoparticles and the fluorophore (anthracene) conjugates with MWNTs via π–π stacking. Finally, the mixture was centrifuged and washed several times with ethanol to remove unbound AHB. The complete removal of unbound AHB was monitored by UV-Vis spectroscopy. The final product contains 22% AHB, 38% Fe3O4 and 40% MWNTs (see Fig. S1, ESI,† for TGA analysis).
Blend preparation
A neat 50/50 (wt/wt) PC/PVDF blend was prepared by mixing the constituent polymers in a Haake Minilab-II extruder at 260 °C temperature and 60 rpm rotational speed for 20 min under a nitrogen atmosphere. For the filled blends, specific nanoparticles were added directly along with the other constituents in the extruder. Few control samples were also prepared under the same extrusion conditions. All the conjugated nanoparticles were in 50/50 (wt/wt) ratios and the filler percentages were mainly adjusted so as to fix the concentration of MWNTs (2 wt%) in the blend.
Characterization
Transmission electron microscopy (FEI Technai F30) operating at 300 kV equipped with an EDS detector was used to evaluate the microstructure and map the elements. A Sirion XL30 FEG SEM with an accelerating voltage of 10 kV was used to assess the morphology of the PC/PVDF blends. A PerkinElmer GX FT-IR instrument was used to acquire FT-IR spectra. A Shimadzu UV-1800 spectrophotometer was used to study the UV-Vis absorption spectra and a Hitachi F-2500 spectrofluorimeter with a 1 cm quartz cell was used for fluorescence measurements. For fluorescence measurements, the excitation and emission slits with a band pass of 2.5 nm were used for all the samples. The PMT (photomultiplier tube) voltage was kept at 700 V and the scan speed was 5 nm s−1. The magnetic properties of the synthesized nanoparticles were assessed using a Lakeshore Vibratory Sample Magnetometer (VSM) with an applied force of −8000 to 8000 Oe at room temperature. X-ray diffraction was recorded using an XPERT Pro from PANalytical. A Cu Kα radiation source (λ = 1.5406 Å, 40 kV and 30 mA) was used to determine the XRD profile of various nanoparticles. Room temperature electrical conductivity of the blends was studied using an Alpha-N Analyser, Novocontrol (Germany) in a frequency range from 0.1 Hz to 10 MHz. A calibrated differential scanning calorimeter (TA Instruments) was used for DSC experiments, where the sample heating rate was 10 K min−1. A room temperature UTM was used for evaluating the mechanical properties of various blend structures. A 5 mm min−1 cross head speed was used for all the experiments. Prior to the measurement dumbbell-shaped samples were prepared by compression moulding. A Discovery hybrid rheometer (DHR 3, TA Instruments) was used to study the melt rheology of various blends. A 25 mm diameter parallel plate geometry was used for these measurements. EM shielding interference was studied using an Anritsu MS4642A VNA. A Damaskos MT-07 co-axial adapter was used and a KEYCOM waveguide was used to measure the scattering parameters of stacked samples.
Results and discussion
Characterization of the novel fluorophore–spacer–receptor system and conjugation with MWNTs and ferrites
The XRD data confirm the successful synthesis of Fe3O4 nanoparticles (JCPDS Card No. 00-001-1111) (Fig. 1a). Furthermore, the TEM image (see the inset of Fig. 1e) shows that the average size of the ferrites is ca. 15–20 nm. The conjugation of MWNTs with ferrite by both covalent and non-covalent strategies is assessed here using XRD and TEM images (Fig. 1b–h). From the TEM images it is evident that a non-covalent approach is better suited for the decoration of Fe3O4 nanoparticles on MWNTs compared to covalent attachment (see Fig. S2, ESI†).
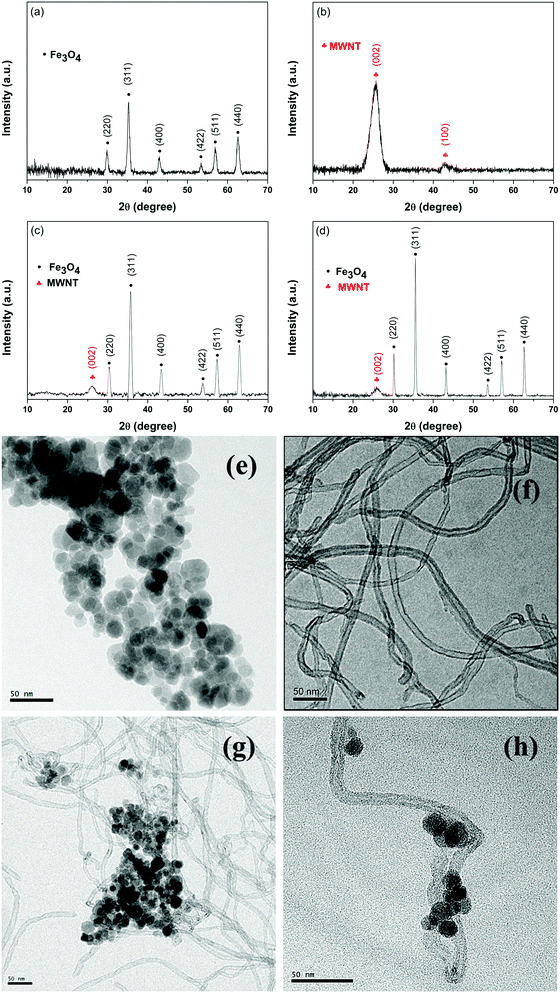 |
| Fig. 1 Characterization of various nanoparticles. XRD patterns of (a) Fe3O4 nanoparticles, (b) MWNTs, (c) MWNT–Fe3O4 and (d) MWNT–AHB–Fe3O4. TEM micrographs of (e) Fe3O4 nanoparticles, (f) MWNTs, (g) MWNT–Fe3O4 and (h) MWNT–AHB–Fe3O4. | |
For non-covalent attachment a novel anthracene hydrazide derivative was synthesized first, the steps of the synthesis were confirmed by FT-IR (Fig. 2a). As we discussed in Scheme 3, the synthetic procedure of AHB is a two-step process; initially we start from 9-anthracenecarboxaldehyde (ACA), where stretching at 1665 cm−1 corresponds to the C
O stretching frequency of aldehyde groups. Interestingly, the diminishing of the C
O stretching peak clearly confirmed the complete reduction of 9-anthracenecarboxaldehyde by hydrazine hydride. Besides, strong peaks appear at 3368 cm−1 corresponding to the N–H stretching frequency and 1550 cm−1 corresponding to the N–H bending frequency which originate from the free amine end groups of hydrazine hydride. The peak at 3368 cm−1 diminishes and a broad peak at 3380–3530 cm−1 corresponding to the O–H stretching frequency appears which confirm the successful reaction with 3-hydroxybezaldehyde (Scheme 3).
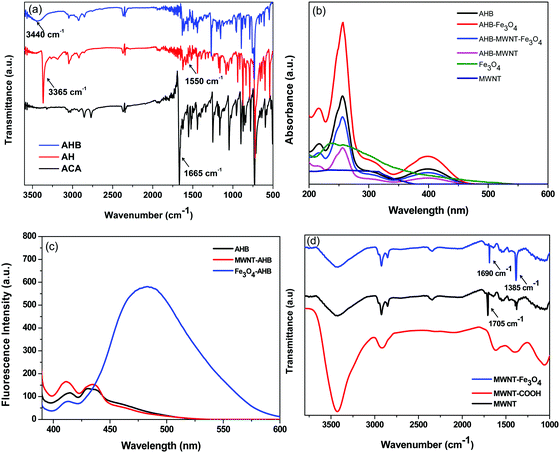 |
| Fig. 2 (a) FT-IR spectra of various intermediate compounds during synthesis of AHB, (b) UV absorption spectra of non-covalently modified nanoparticles, (c) fluorescence absorption of non-covalently modified nanoparticles, and (d) FT-IR spectra of covalently modified MWNTs. | |
The synthesized AHB has an extended π-electron cloud associated with the anthracene moiety which can be used to conjugate with the π-electron cloud of MWNTs. The attachment of AHB was further confirmed by UV-vis and fluorescence emission spectroscopy. In general the MWNTs do not show any absorption by UV or fluorescence emission spectroscopy but after non-covalent attachment of AHB, the absorption due to AHB is clearly evident. In general in the UV-Vis spectrum, a peak at 255 nm corresponds to the π–π* transition and a peak at 398 nm corresponds to the n–π* transition. The unbound AHB molecules were thoroughly washed and the hybrid nanoparticles were monitored using UV-vis spectroscopy (Fig. S3 in the ESI†). The absorption in the hybrid nanomaterials is from the AHB molecules that are non-covalently attached to the surface of MWNTs (Fig. 2b). On the other hand, the receptors of AHB molecules have the tendency to co-ordinately bind with Fe3O4 nanoparticles and exhibit a strong fluorescence emission (Fig. 2c). Here the binding of Fe3O4 occurs by forming a static excimer of AHB molecules as manifested from the perturbation of the emission spectrum which even scaled with increasing concentration of Fe3O4. During the covalent attachment, the MWNTs were given a harsh treatment to introduce appropriate surface functional groups in order to be able to conjugate with the Fe3O4 nanoparticles. The covalent surface functionalization of the nanoparticles was done in successive steps and confirmed by FT-IR (Fig. 2d). As we discussed earlier in Scheme 2, the covalent attachment of Fe3O4 on MWNTs is a two-step procedure, initially we started from acid functionalization of MWNTs and the peak at 1705 cm−1 corresponds to the C
O stretching of the carboxylic acid groups. Furthermore, in the next step, amine terminated Fe3O4 nanoparticles were reacted with a carboxylic acid group and the formation of an amide bond was confirmed by a peak at 1690 cm−1.
Fig. 3a depicts the Raman spectra of MWNTs after different types of modifications. The spectrum clearly shows two signature bands of a carbon system; a G-band which corresponds to the stretching of sp2 hybridized carbon atoms and a D-band which corresponds to the disordered carbon atoms and defects. The intensity ratio of the bands (ID/IG) indicates the amount of disorder and defects in the structure. It is observed that the acid functionalization of MWNTs introduced a greater number of defect sites when compared with the non-covalent attachment of Fe3O4 nanoparticles to the MWNTs.
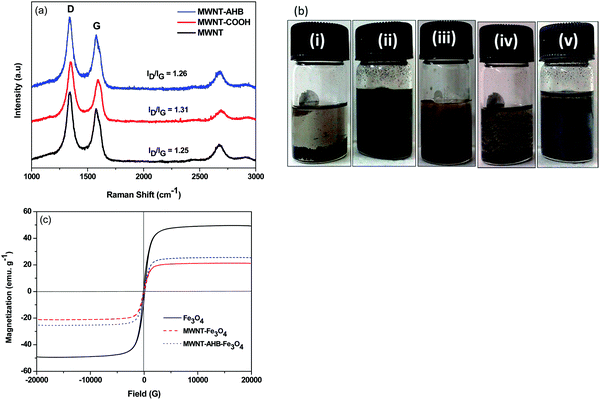 |
| Fig. 3 (a) Raman spectra of different modified MWNTs, (b) dispersion test of various nanomaterials in ethanol where (i) MWNTs, (ii) MWNT–AHB, (iii) MWNT + Fe3O4, (iv) MWNT–Fe3O4 and (v) MWNT–AHB–Fe3O4. (c) Room temperature VSM plot of various nanoparticles. | |
The state of dispersion was assessed here by solution dissolution experiments. Fig. 3b depicts the visual pictures of various dispersions in ethanol, 1 h after the initial bath sonication process. It is observed that the non-covalent surface modification approach showed much better dispersion quality as compared to the covalent approach. The magnetic properties of various synthesized particles were analysed by the VSM study (Fig. 3c). A drastic decrease in saturation magnetization of Fe3O4 was observed after conjugating it with MWNTs (Table 1).
Table 1 Room temperature magnetic hysteresis parameters of different particles
Particle |
Saturation magnetization (MS) (emu g−1) |
Remnant magnetization (MR) (emu g−1) |
Coercivity (HC) (Oe) |
MWNT39 |
0.344 |
0.10 |
234 |
Fe3O4 |
49 |
19 |
380 |
MWNT–Fe3O4 |
22 |
16 |
240 |
MWNT–AHB–Fe3O4 |
25 |
16 |
260 |
Selective localization of hybrid nanoparticles in PC/PVDF blends
Tailor-made properties with precise control of nanoparticles can be achieved by blending two polymers.34 However, the lack of specific interactions or chemical incompatibility between the constituents often leads to heterogeneous morphology. So, monitoring the phase morphology after blending is the determining factor for the resulting properties. For the SEM analysis, the PC phase was etched out by chloroform to enhance the contrast. It is evident from the micrographs that a co-continuous morphology is obtained here by blending PC/PVDF in a 50/50 wt% ratio (Fig. 4a). With the addition of MWNTs or MWNT–Fe3O4 the co-continuity of the phases is retained as seen from Fig. 4b and c. Interestingly, although the PC phase is thermodynamically more favoured, the nanoparticles preferentially localize in the PVDF phase due to its higher polarity compared to PC.35 High resolution SEM micrographs confirm the presence of nanoparticles in the PVDF phase (Fig. 4d). The selective dissolution experiments further support this observation, where the MWNTs containing blend was initially suspended in CHCl3 (to dissolve the PC phase) and later suspended in DMF to remove the PVDF phase. The obtained solution after complete removal of the PC phase remains colorless; whereas a black colored solution was obtained immediately after suspending the sample in DMF. This reveals the preferential localization of MWNTs in the PVDF phase (Fig. 4e). Selective localization of Fe3O4 nanoparticles in PVDF was further confirmed by EDAX mapping (Fig. 4f). Further crystallization behaviour (discussed in the ESI†) is the key factor especially for semicrystalline polymers like PVDF for dictating their mechanical performance. In general, crystallization and melting temperatures are greatly affected by the presence of various rigid nanoparticles and their distribution inside the matrix. So the preferential localization of various nanoparticles in the PVDF phase was further assessed by DSC experiments; changes in the crystallization and melting temperatures of PVDF (Table S1 in the ESI†) clearly indicate the presence of a heteronucleating effect of these nanoparticles in the PVDF phase (Fig. S4 in the ESI†). In addition the mechanical properties (Fig. S5 in the ESI†) and rheological properties (Fig. S6 in the ESI†) of these blends are also assessed here. It is evident that the mechanical properties deteriorate upon chemically conjugating the hybrid particles, whereas they are either retained or slightly enhanced in the presence of hybrid nanoparticles conjugated by non-covalent treatment.
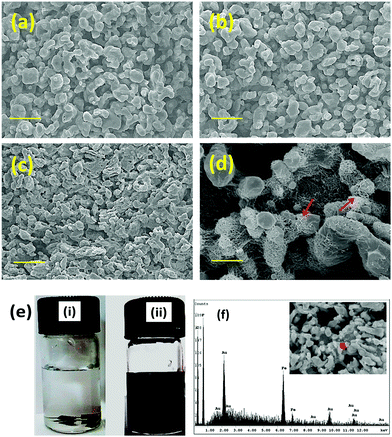 |
| Fig. 4 SEM morphology of (a) neat 50/50 PC/PVDF, (b) with 2 wt% MWNTs and (c) with 2 wt% MWNTs + Fe3O4. (d) High resolution SEM images, arrows indicate the localization of MWNTs. (e) Solution dissolution experiment of a PC/PVDF blend with 2 wt% MWNTs + Fe3O4 where (i) a sample dissolved in chloroform for etching out the PC phase; colorless solution confirms no particle distribution in the PC phase and (ii) a sample dissolved in DMF for etching out the PVDF phase; black colored solution confirms the selective particle localization at PVDF. (f) EDAX spectra of a 2 wt% MWNTs + Fe3O4 containing blend after etching out the PVDF phase. | |
Heterogeneous dispersion of nanoparticles in immiscible blends: effect on bulk electrical conductivity
An interconnected network of conducting nanoparticles is the prime requisite for charge transportation through insulting polymer composites.36,37 The preferential localization of such nanoparticles in an energetically favoured phase of an immiscible polymer blend readily enhances the blend conductivity due to significant enhancement in the effective filler concentration in a given phase. In our previous study we showed that the percolation threshold of MWNTs in the 50/50 PC/PVDF blend is in the range of 0.5–1 wt% MWNTs. Fig. 5a depicts the AC electrical conductivity of various blends as a function of frequency. Interestingly, AHB modified MWNTs showed better electrical conductivity than unmodified MWNTs. As discussed earlier the effective aspect ratio of a conducting filler is a decisive parameter in controlling the final blend conductivity. However, due to agglomeration, led by van der Waals forces of attraction, the effective aspect ratio reduces to a greater extent. In this context, surface functionalization by non-covalent attachment of AHB molecules on the MWNTs facilitates better exfoliation resulting in higher electrical conductivity. After addition of Fe3O4 nanoparticles the overall AC electrical conductivity of the blends decreased significantly. As Fe3O4 nanoparticles are selectively localized in the PVDF phase, it impedes the three-dimensional conducting pathways of MWNTs. This phenomenon is more prominent when Fe3O4 nanoparticles were directly added to the matrix without any surface modification, which readily agglomerates and reduces the bulk conductivity of the blends. By non-covalent attachment of Fe3O4 nanoparticles onto MWNTs, a significant improvement in AC electrical conductivity was observed. This can be appreciated by comparing the electrical conductivity of the blends containing MWNT–Fe3O4 conjugated by covalent means. It is envisaged that the structural integrity of MWNTs is affected due to harsh acid treatment resulting in defects. These become scattering sites and impede the charge transport in MWNTs.
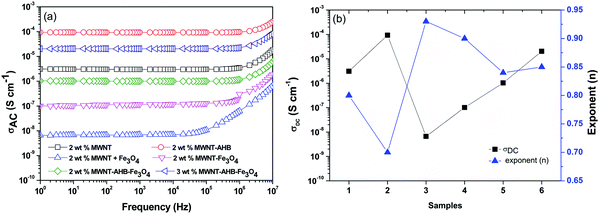 |
| Fig. 5 Conductivity: (a) AC electrical conductivity of various blends with frequency variation and (b) DC electrical conductivity and the value of the exponent with respect to various blends: (1) 2 wt% MWNTs, (2) 2 wt% MWNT–AHB, (3) 2 wt% MWNT + Fe3O4, (4) 2 wt% MWNT–Fe3O4, (5) 2 wt% MWNT–AHB–Fe3O4 and (6) 3 wt% MWNT–AHB–Fe3O4 along the X-axis. | |
The charge transport phenomenon of the conducting network inside the various blends was further analysed by the power law equation:
| σ′(ω) = σ(0) + σAC(ω) = σDC + Aωn | (1) |
where
ω is the angular frequency,
σDC is the dielectric constant,
A is the temperature dependent constant and
n is the exponent. The exponent is the measure of the three dimensional network of a capacitor or resistor and depends on both frequency and temperature and the value is in the range of 0–1. The charge transport mechanism is by hopping when
n < 0.7. By fitting the AC electrical conductivity with
eqn (1), different parameters are obtained and the exponent is plotted in
Fig. 5b. It is observed that
σDC increases after AHB modification, whereas the exponent decreased to 0.70 indicating charge transport by electron hopping. After the addition of Fe
3O
4 nanoparticles the ‘
n’ has increased indicating resistive behaviour. But interestingly applying the non-covalent attachment routes, the value of ‘
n’ suggests more capacitance behavior indicating better distribution of nanoparticles in the matrix.
EMI shielding properties: synergetic effect from dielectric and magnetic dipoles
The amount of attenuation of incident EM waves is analysed by assessing the total shielding effectiveness (SET) and expressed in dB. It is mainly the quantitative measure of dissipated EM waves. Generally, when the value of SET reaches 20 dB, the material is capable of attenuating 99% of the incident EM waves.6 It is also well known that SET is the combination of three components i.e. shielding by reflection (SER), shielding by absorption (SEA) and shielding by multiple reflection (SEMR). | SET = SEA + SER + SEMR | (2) |
But SEMR can be neglected when SEA is greater than 15 dB or the shield thickness is higher than the skin depth due to increasing absorption from the internal surface.6,38 So SET can be expressed as,from the VNA, the SET can be estimated from scattering parameters (S11 is a forward reflection coefficient, S12 is a reverse transmission coefficient and S21 is a forward transmission coefficient) and is expressed as, |  | (4) |
by knowing the scattering parameters we can also assess the total reflection and absorption by the following equations, | 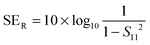 | (5) |
| 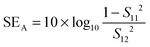 | (6) |
Fig. 6a depicts the total shielding efficiency of various blends as a function of frequency. While neat blends are transparent to EM radiation, SET increases with the addition of MWNTs in the blend. It is well understood that the material should have adequate electrical conductivity for EM attenuation. In addition, the dielectric loss should also be high for effective shielding. So, the connecting network of the conducting phase, which can carry the mobile charge, is important for EM attenuation rather than very high electrical conductivity. The latter only results in surface reflection. Here in the case of immiscible blends, the selective localization of conducting nanoparticles in a given phase facilitates the formation of a connecting network at comparatively lower filler content. Hence, in this case multiple scattering within the network of CNTs (of very high specific surface area) attenuates EM waves mostly by absorption rather than reflection. The PC/PVDF (50/50) blends with 2 wt% MWNTs exhibited considerably higher shielding efficiency than individual composites in a similar weight fraction of MWNTs (Table 2) signifying the potential of conducting polymer blends over nanocomposites in designing efficient shielding materials. After the addition of AHB modified MWNTs, the SET was enhanced due to better exfoliation of conducting nanoparticles thereby forming a finer mesh for the conducting network that can carry mobile charges easily. However, as we discussed earlier to shield from EM radiation both electrical and magnetic dipoles are necessary as the radiation is a synchronized propagation of both electric and magnetic vector components. Therefore, after incorporation of Fe3O4 nanoparticles along with the conducting nanoparticles into the blend, SET has increased significantly by several orders. The interfacial dielectric polarization of MWNTs resulted in the absorption of the electric component whereas a magnetic hysteresis loss through incorporation of Fe3O4 nanoparticles has led to the absorption of a magnetic vector component of the incident electromagnetic wave. The improvement in SET is lower when the Fe3O4 nanoparticles are directly added into the blend without any surface modification, resulting in an uneven distribution of Fe3O4 nanoparticles which actually impedes the conducting network.
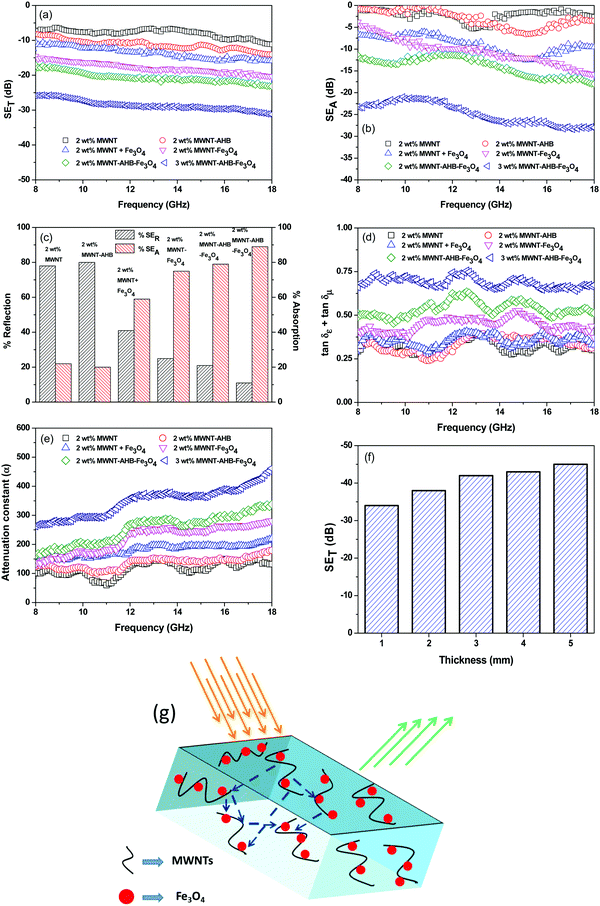 |
| Fig. 6 EMI shielding properties of various blends: (a) total shielding effectiveness in respect of frequency, (b) total absorption ability in respect of frequency, (c) % absorption and % reflection, (d) consolidated tangent loss in respect of frequency, (e) attenuation constant in respect of frequency, (f) total shielding effectiveness in respect of thickness of the highest attenuating sample and (g) a schematic representation of the shielding mechanism of the highest attenuating modulating sample. | |
Table 2 Total shielding effectiveness of various blends at 18 GHz frequency for 0.9 mm thick toroidal samples
Composition |
Total shielding effectiveness (dB) |
50/50 PC/PVDF |
0 |
50/50 PC/PVDF with 2 wt% MWNT |
−11 |
PVDF with 2 wt% MWNT |
−4 |
50/50 PC/PVDF with 2 wt% MWNT–AHB |
−14 |
50/50 PC/PVDF with 2 wt% MWNT + Fe3O4 |
−16 |
50/50 PC/PVDF with 2 wt% MWNT–Fe3O4 |
−20 |
50/50 PC/PVDF with 2 wt% MWNT–AHB–Fe3O4 |
−23 |
50/50 PC/PVDF with 3 wt% MWNT–AHB–Fe3O4 |
−31 |
The attachment of Fe3O4 nanoparticles onto MWNTs by non-covalent means retained the exfoliated interconnected conducting network of the nanotubes, an essential criterion for EM attenuation. This intact structural integrity of conducting nanoparticles facilitated enhanced bulk conductivity as well as attenuation over hybrid particles that were conjugated by covalent means. Furthermore, increasing the concentration of hybrid nanoparticles in the blend increased the total shielding efficiency. The blend with 3 wt% MWNT–AHB–Fe3O4 exhibited an SET of −45 dB due to synergistic effects of both MWNTs and Fe3O4 nanoparticles. More interestingly, the incorporation of Fe3O4 nanoparticles in the blend not only improved the shielding efficiency, but also altered the mechanism of shielding. In general, the EM attenuation is mostly dominated by reflection in conducting surfaces (like metals) whereas attenuation by absorption is facilitated by hybrid nanoparticles where both electric and magnetic dipoles are present (Fig. 6b and c). In order to understand the shielding mechanism we evaluated the complex (relative) permittivity (εr) and the complex relative permeability (μr) of the blends through a well-established line theory.39–42 In an air field coaxial line, the reflection coefficient can be expressed as,
| 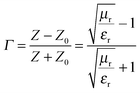 | (7) |
if thickness is expressed as
t, then transmission coefficient can be expressed as,
|  | (8) |
where complex (relative) permittivity is
μr and complex relative permeability is
εr. The characteristic impedance of the specimen mounting region is
Z and
Z0 is the characteristic impedance through a coaxial line. The different scattering parameters observed from the VNA, as we stated earlier, can be expressed as,
now, by using scattering parameters the reflection coefficient can be calculated using the following equation,
| 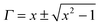 | (11) |
where

. Furthermore,
| 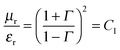 | (12) |
| 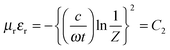 | (13) |
so, the complex permittivity can be expressed as

and complex permeability as

.
The parameters μr and εr convey the ability of materials to attenuate EM radiation, while storage ability is the main source of polarization and dissipated energy is associated with the loss. So the shielding efficiency can be altered by the inclusion of hybrid nanoparticles which consist of both electrical and magnetic dipoles. Generally, when the incident EM wave interacts with such hybrid nanoparticles the resultant local field disparity can have a strong consequence on the energy of absorption at the boundaries as absorption depends quadratically on the electric field intensity. These complex parameters are directly related to the absorption and reflection coefficients of the total shielding effectiveness expressed by these following equations:
| 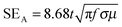 | (14) |
| 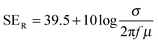 | (15) |
where
μ =
μ0μr and
σ = 2π
fε0ε′′. So it is clear that shielding by absorption is increased with increasing values of
μr and
εr where
μr =
μ′ −
jμ′′ and
εr =
ε′ −
jε′′. So the relative permittivity (
εr) and permeability (
μr) values mainly depend on the real and imaginary parts of the permittivity and permeability values. The dielectric and magnetic lossy characteristics (
ε′′ and
μ′′), the real parts of permittivity (
ε′) and permeability (
μ′), dielectric and magnetic tangent (tan
δ) were estimated to obtain an insight into the mechanism of shielding, where tan
δε =
ε′′/
ε′ and tan
δμ =
μ′′
/μ′. The total loss tangent (tan
δε + tan
δμ) can give more insight into the mechanism of shielding.
Fig. 6d depicts consolidated loss tangent values of various blends as a function of frequency. For blends with only MWNTs, the consolidated loss is because of dielectric loss and interfacial dielectric polarization as they are embedded in a dielectric matrix. The effect of Fe
3O
4 nanoparticles on the EM attenuation is clearly evident from the enhancement of loss tangent over only the conducting phase. In general, magnetic loss originates from the magnetic hysteresis losses due to natural resonance and eddy current in GHz frequency. The consolidated loss part also scales up by a different modification technique. By physical mixing the effect is lower because the permittivity values are lower due to an impeded conducting network. A similar effect is also noted in hybrid particle filled blends wherein the hybrid particles were covalently conjugated. This could be due to structural defects in MWNTs due to harsh chemical treatment that decreased the overall conductivity/permittivity values. In the case of non-covalent modification, the loss tangent is higher as compared to other blends. Further, increasing the concentration of hybrid nanoparticles increases the total loss resulting in 86% absorption of the incident EM radiation.
For more clear understanding of the shielding mechanism, the attenuation constant (α), which is a measure of electromagnetic wave absorption penetrated through the shield, was estimated using the complex microwave properties.43α can be expressed as:
|  | (16) |
where
c is the speed of light.
Fig. 6e depicts the attenuation constants of various blends as a function of frequency. It is well evident that
α scales with a consolidated loss tangent. The highest
α in the case of MWNT–AHB–Fe
3O
4 containing blend suggested maximum absorption ability.
The shield thickness is also an important parameter for effective shielding.6 To evaluate the effect of thickness on the total shielding efficiency, we evaluated the SET of a 3 wt% MWNT–AHB–Fe3O4 containing blend at different shield thicknesses. From Fig. 6f it is obvious that shielding effectiveness scales with the sample thickness. Generally, EM attenuation is governed by interconnected conducting and magnetic networks. Therefore, attenuation of incident radiation is improved by increasing the sample thickness. The skin depth (δ) of the highest attenuating sample was evaluated using the following equation,
|  | (17) |
the skin depth of the highest attenuating sample is
ca. 1.12 mm. A cartoon illustrating the possible shielding mechanism is illustrated in
Fig. 6g. As we discussed earlier, in the case of MWNT–AHB–Fe
3O
4 containing blend the observed attenuation was mostly due to the absorption of incident EM radiation. The synergetic effect of large dielectric losses arising from conducting MWNTs and magnetic losses generated from ferrimagnetic nanoparticles has resulted in excellent shielding efficiency.
Layer-by-layer assembly: a potential ultra-thin EMI shield
The outstanding shielding performance of individual materials further led us to design layer-by-layer (LbL) stacks of different polymer blends in designing lightweight, flexible and easy to design shielding materials that can be explored for myriad opportunities. But, most of the fabrication techniques for LbL assembly are not cost effective, difficult to process and require a long time to prepare. Here we prepared LbL assembly by a simple compression moulding technique which is described schematically in Fig. 7a. It is well understood from the previous section that incorporation of heterogeneous hybrid nanomaterials improved the EM attenuation property by absorbing the electric and magnetic components of incident EM waves in contrast to MWNTs which showed reflection dominated shielding. However the non-covalent attachment technique showed better EM attenuation property over the direct physical mixing/covalent attachment, restricting 3 wt% MWNT–AHB–Fe3O4 nanoparticles containing blend as the upper and bottom layers of the assembly to maximize the total absorption of the incident EM radiation. Furthermore the penetration of incident EM radiation from the upper and bottom layers is modulated by the highly conducting inner layer of 2 wt% MWNT–AHB containing blend. In general, the EM shielding originates from the multiple reflection of conducting MWNT surfaces. So the penetrated EM radiation is readily absorbed by multiple reflections within the interconnected exfoliated MWNT network in the inner layer, resulting in small heating of the sample. Besides, due to heterogeneous dielectric polarizabilities, the accumulation of the virtual charges at the interface of the two layers having different dielectric constants and conductivities can lead to interfacial polarization, which will also enhance the total EM shielding efficiency. Finally by taking advantage of this LbL technique we achieved a SET of −60 dB with just a 0.90 mm thick film (Fig. 7b) wherein the individual layers have low shielding efficiency (see Table 3). The mechanism of shielding of this layer architecture is schematically represented in Fig. 7c. Our results have been compared with the available literature on MWNTs and Fe3O4 and are listed in Table S2 (see ESI†).
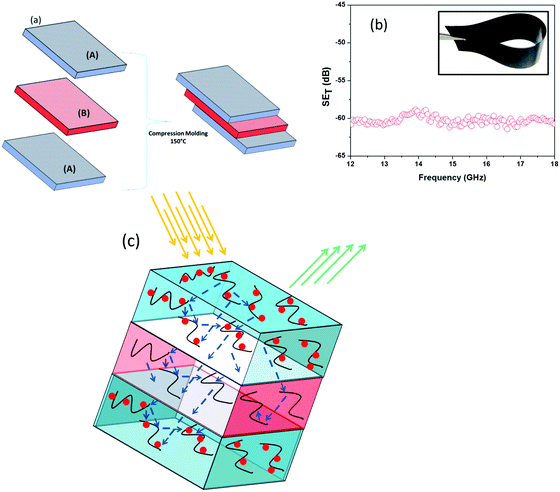 |
| Fig. 7 (a) Layer-by-layer assembly technique of an EM shield architecture where (A) corresponds to a blend with 2 wt% MWNT–AHB–Fe3O4 and (B) corresponds to a blend with 2 wt% MWNT–AHB. (b) SET of an LbL architecture and the inset shows the flexibility of the LbL structure, and (c) the shielding mechanism of a LbL structure. | |
Table 3 Total shielding effectiveness of a layer-by-layer structure with different thicknesses and compositions
Composition |
Thickness (mm) |
Total shielding effectiveness (dB) |
50/50 PC/PVDF with 3 wt% MWNT–AHB–Fe3O4 |
0.30 |
−19 |
(A) |
0.90 |
−31 |
50/50 PC/PVDF with 2 wt% MWNT–AHB |
0.30 |
−9 |
(B) |
0.90 |
−14 |
Layer-by-layer assembly (LbL) of A–B–A |
0.90 |
−60 |
Conclusions
In summary, our study clearly demonstrated that a novel fluorophore–spacer–receptor system, containing hydrazono methyl phenol as the receptor, anthracene as the fluorophore and imine (C
N) groups as the spacer, can assist in conjugating ferrites and carbon based conductive phases. These hybrid particles in co-continuous PC/PVDF blends exhibited outstanding EMI shielding in contrast to direct physical mixing/covalent conjugation approaches. The designed hybrid nanoparticles were selectively localized in the PVDF phase and thereby increased the bulk electrical conductivity and the overall losses in the blends. The hybrid particle filled blends showed >99.99% EM attenuation dominated mostly by absorption. Taking advantage of the shielding efficiency of the individual components, a multilayered stack was constructed with outer layers containing PC/PVDF blends with a MWNT–ferrite hybrid and the inner layers consisting of PC/PVDF blends with only MWNTs. An ultra-thin shield of 0.90 mm showed >99.9999% attenuation suggesting new pathways for designing lightweight, flexible EMI shielding materials.
Acknowledgements
The authors sincerely acknowledge Ms Dipanwita Chatterjee for TEM studies and Goutam P. Kar for TGA studies. The authors gratefully acknowledge the financial support from DST (India) and JATP (IISc).
References
- N. Li, Y. Huang, F. Du, X. He, X. Lin, H. Gao, Y. Ma, F. Li, Y. Chen and P. C. Eklund, Electromagnetic interference (EMI) shielding of single-walled carbon nanotube epoxy composites, Nano Lett., 2006, 6(6), 1141–1145 CrossRef CAS PubMed.
- Y. Yang, M. C. Gupta, K. L. Dudley and R. W. Lawrence, Novel carbon nanotube-polystyrene foam composites for electromagnetic interference shielding, Nano Lett., 2005, 5(11), 2131–2134 CrossRef CAS PubMed.
- H.-B. Zhang, Q. Yan, W.-G. Zheng, Z. He and Z.-Z. Yu, Tough graphene–polymer microcellular foams for electromagnetic interference shielding, ACS Appl. Mater. Interfaces, 2011, 3(3), 918–924 CAS.
- D. Chung, Electromagnetic interference shielding effectiveness of carbon materials, Carbon, 2001, 39(2), 279–285 CrossRef CAS.
- S. P. Pawar, S. Biswas, G. P. Kar and S. Bose, High frequency millimetre wave absorbers derived from polymeric nanocomposites, Polymer, 2016, 84, 398–419 CrossRef CAS.
- M. H. Al-Saleh and U. Sundararaj, Electromagnetic interference shielding mechanisms of CNT/polymer composites, Carbon, 2009, 47(7), 1738–1746 CrossRef CAS.
- M. Arjmand, M. Mahmoodi, G. A. Gelves, S. Park and U. Sundararaj, Electrical and electromagnetic interference shielding properties of flow-induced oriented carbon nanotubes in polycarbonate, Carbon, 2011, 49(11), 3430–3440 CrossRef CAS.
- X. Luo and D. Chung, Electromagnetic interference shielding using continuous carbon-fiber carbon-matrix and polymer-matrix composites, Composites, Part B, 1999, 30(3), 227–231 CrossRef.
- M.-S. Cao, W.-L. Song, Z.-L. Hou, B. Wen and J. Yuan, The effects of temperature and frequency on the dielectric properties, electromagnetic interference shielding and microwave-absorption of short carbon fiber/silica composites, Carbon, 2010, 48(3), 788–796 CrossRef CAS.
- J. Liang, Y. Wang, Y. Huang, Y. Ma, Z. Liu, J. Cai, C. Zhang, H. Gao and Y. Chen, Electromagnetic interference shielding of graphene/epoxy composites, Carbon, 2009, 47(3), 922–925 CrossRef CAS.
- V. Eswaraiah, V. Sankaranarayanan and S. Ramaprabhu, Functionalized graphene–PVDF foam composites for EMI shielding, Macromol. Mater. Eng., 2011, 296(10), 894–898 CrossRef CAS.
- A. P. Singh, P. Garg, F. Alam, K. Singh, R. Mathur, R. Tandon, A. Chandra and S. Dhawan, Phenolic resin-based composite sheets filled with mixtures of reduced graphene oxide, γ-Fe2O3 and carbon fibers for excellent electromagnetic interference shielding in the X-band, Carbon, 2012, 50(10), 3868–3875 CrossRef CAS.
- T. K. Gupta, B. P. Singh, S. R. Dhakate, V. N. Singh and R. B. Mathur, Improved nanoindentation and microwave shielding properties of modified MWCNT reinforced polyurethane composites, J. Mater. Chem. A, 2013, 1(32), 9138–9149 CAS.
- S. Biswas, G. P. Kar and S. Bose, Simultaneous Improvement in Structural Properties and Microwave Shielding of Polymer Blends with Carbon Nanotubes, ChemNanoMat, 2016, 2(2), 140–148 CrossRef CAS.
- S. K. Rath, S. Dubey, G. S. Kumar, S. Kumar, A. Patra, J. Bahadur, A. Singh, G. Harikrishnan and T. U. Patro, Multi-walled CNT-induced phase behaviour of poly(vinylidene fluoride) and its electro-mechanical properties, J. Mater. Sci., 2014, 49(1), 103–113 CrossRef CAS.
- G. P. Kar, S. Biswas and S. Bose, Tuning the microwave absorption through engineered nanostructures in co-continuous polymer blends, Mater. Res. Express, 2016, 3(6), 064002 CrossRef.
- G. P. Kar, S. Biswas, R. Rohini and S. Bose, Tailoring the dispersion of multiwall carbon nanotubes in co-continuous PVDF/ABS blends to design materials with enhanced electromagnetic interference shielding, J. Mater. Chem. A, 2015, 3(15), 7974–7985 CAS.
- F. Ren, H. Yu, L. Wang, M. Saleem, Z. Tian and P. Ren, Current progress on the modification of carbon nanotubes and their application in electromagnetic wave absorption, RSC Adv., 2014, 4(28), 14419–14431 RSC.
- M.-S. Cao, J. Yang, W.-L. Song, D.-Q. Zhang, B. Wen, H.-B. Jin, Z.-L. Hou and J. Yuan, Ferroferric oxide/multiwalled carbon nanotube vs polyaniline/ferroferric oxide/multiwalled carbon nanotube multiheterostructures for highly effective microwave absorption, ACS Appl. Mater. Interfaces, 2012, 4(12), 6949–6956 CAS.
- P. Saini, V. Choudhary, B. Singh, R. Mathur and S. Dhawan, Polyaniline–MWCNT nanocomposites for microwave absorption and EMI shielding, Mater. Chem. Phys., 2009, 113(2), 919–926 CrossRef CAS.
- H. Kim, K. Kim, C. Lee, J. Joo, S. Cho, H. Yoon, D. Pejaković, J.-W. Yoo and A. Epstein, Electrical conductivity and electromagnetic interference shielding of multiwalled carbon nanotube composites containing Fe catalyst, Appl. Phys. Lett., 2004, 84(4), 589–591 CrossRef CAS.
- Y. Liu, D. Song, C. Wu and J. Leng, EMI shielding performance of nanocomposites with MWCNTs, nanosized Fe3O4 and Fe, Composites, Part B, 2014, 63, 34–40 CrossRef CAS.
- P. Saini, V. Choudhary, N. Vijayan and R. Kotnala, Improved electromagnetic interference shielding response of poly(aniline)-coated fabrics containing dielectric and magnetic nanoparticles, J. Phys. Chem. C, 2012, 116(24), 13403–13412 CAS.
- B. Shen, W. Zhai, M. Tao, J. Ling and W. Zheng, Lightweight, multifunctional polyetherimide/graphene@Fe3O4 composite foams for shielding of electromagnetic pollution, ACS Appl. Mater. Interfaces, 2013, 5(21), 11383–11391 CAS.
- K. Singh, A. Ohlan, V. H. Pham, R. Balasubramaniyan, S. Varshney, J. Jang, S. H. Hur, W. M. Choi, M. Kumar and S. Dhawan, Nanostructured graphene/Fe3O4 incorporated polyaniline as a high performance shield against electromagnetic pollution, Nanoscale, 2013, 5(6), 2411–2420 RSC.
- Y.-L. Ren, H.-Y. Wu, M.-M. Lu, Y.-J. Chen, C.-L. Zhu, P. Gao, M.-S. Cao, C.-Y. Li and Q.-Y. Ouyang, Quaternary nanocomposites consisting of graphene, Fe3O4@Fe core@ shell, and ZnO nanoparticles: synthesis and excellent electromagnetic absorption properties, ACS Appl. Mater. Interfaces, 2012, 4(12), 6436–6442 CAS.
- S. Biswas, G. P. Kar and S. Bose, Attenuating microwave radiation by absorption through controlled nanoparticle localization in PC/PVDF blends, Phys. Chem. Chem. Phys., 2015, 17(41), 27698–27712 RSC.
- G. P. Kar, S. Biswas and S. Bose, Simultaneous enhancement in mechanical strength, electrical conductivity, and electromagnetic shielding properties in PVDF–ABS blends containing PMMA wrapped multiwall carbon nanotubes, Phys. Chem. Chem. Phys., 2015, 17(22), 14856–14865 RSC.
- J.-M. Thomassin, C. Jérôme, T. Pardoen, C. Bailly, I. Huynen and C. Detrembleur, Polymer/carbon based composites as electromagnetic interference (EMI) shielding materials, Mater. Sci. Eng., R, 2013, 74(7), 211–232 CrossRef.
- S.-H. Park, P. T. Theilmann, P. M. Asbeck and P. R. Bandaru, Enhanced electromagnetic interference shielding through the use of functionalized carbon-nanotube-reactive polymer composites, IEEE Trans. Nanotechnol., 2010, 9(4), 464–469 CrossRef.
- E. Vázquez and M. Prato, Carbon nanotubes and microwaves: interactions, responses, and applications, ACS Nano, 2009, 3(12), 3819–3824 CrossRef PubMed.
- H. Wang, G. Wang, W. Li, Q. Wang, W. Wei, Z. Jiang and S. Zhang, A material with high electromagnetic radiation shielding effectiveness fabricated using multi-walled carbon nanotubes wrapped with poly(ether sulfone) in a poly(ether ether ketone) matrix, J. Mater. Chem., 2012, 22(39), 21232–21237 RSC.
- S. Mohapatra, S. Sahu, S. Nayak and S. K. Ghosh, Design of Fe3O4@SiO2@Carbon Quantum Dot Based Nanostructure for Fluorescence Sensing, Magnetic Separation, and Live Cell Imaging of Fluoride Ion, Langmuir, 2015, 31(29), 8111–8120 CrossRef CAS PubMed.
-
C. Harrats, S. Thomas and G. Groeninckx, Micro-and nanostructured multiphase polymer blend systems: phase morphology and interfaces, CRC Press, 2005 Search PubMed.
- S. Biswas, G. P. Kar and S. Bose, Engineering nanostructured polymer blends with controlled nanoparticle location for excellent microwave absorption: a compartmentalized approach, Nanoscale, 2015, 7(26), 11334–11351 RSC.
- S. Maiti, S. Suin, N. K. Shrivastava and B. Khatua, A strategy to achieve high electromagnetic interference shielding and ultra low percolation in multiwall carbon nanotube–polycarbonate composites through selective localization of carbon nanotubes, RSC Adv., 2014, 4(16), 7979–7990 RSC.
- P. Dey, K. Naskar, B. Dash, S. Nair, G. Unnikrishnan and G. B. Nando, Selective dispersion of carbon fillers into dynamically vulcanized rubber/plastic blends: a thermodynamic approach to evaluate polymer reinforcement and conductivity enhancement, RSC Adv., 2015, 5(40), 31886–31900 RSC.
- M. Mahmoodi, M. Arjmand, U. Sundararaj and S. Park, The electrical conductivity and electromagnetic interference shielding of injection molded multi-walled carbon nanotube/polystyrene composites, Carbon, 2012, 50(4), 1455–1464 CrossRef CAS.
- S. Biswas, G. P. Kar and S. Bose, Tailor-Made Distribution of Nanoparticles in Blend Structure toward Outstanding Electromagnetic Interference Shielding, ACS Appl. Mater. Interfaces, 2015, 7(45), 25448–25463 CAS.
- S. Abbas, A. Dixit, R. Chatterjee and T. Goel, Complex permittivity and microwave absorption properties of BaTiO3–polyaniline composite, Mater. Sci. Eng., B, 2005, 123(2), 167–171 CrossRef.
- Y. Hong, C. Lee, C. Jeong, D. Lee, K. Kim and J. Joo, Method and apparatus to measure electromagnetic interference shielding efficiency and its shielding characteristics in broadband frequency ranges, Rev. Sci. Instrum., 2003, 74(2), 1098–1102 CrossRef CAS.
- S. Biswas, G. P. Kar and S. Bose, Microwave absorbers designed from PVDF/SAN blends containing multiwall carbon nanotubes anchored cobalt ferrite via a pyrene derivative, J. Mater. Chem. A, 2015, 3(23), 12413–12426 CAS.
- W. Li, H. Qi, X. Niu, F. Guo, X. Chen, L. Wang and B. Lv, Fe–Fe3 C/C microspheres as a lightweight microwave absorbent, RSC Adv., 2016, 6(30), 24820–24826 RSC.
Footnote |
† Electronic supplementary information (ESI) available. See DOI: 10.1039/c6qm00074f |
|
This journal is © the Partner Organisations 2017 |