DOI:
10.1039/C7FO01515A
(Review Article)
Food Funct., 2018,
9, 22-41
Enhanced delivery of lipophilic bioactives using emulsions: a review of major factors affecting vitamin, nutraceutical, and lipid bioaccessibility
Received
28th September 2017
, Accepted 4th November 2017
First published on 6th November 2017
Abstract
Many researchers are currently developing emulsion-based delivery systems to increase the bioavailability of lipophilic bioactive agents, such as oil-soluble vitamins, nutraceuticals, and lipids. Oil-in-water emulsions can be specifically designed to improve the bioavailability of these bioactives by altering their composition and structural organization. This article reviews recent progress in understanding the impact of emulsion properties on the bioaccessibility of lipophilic bioactive agents, including oil phase composition, aqueous phase composition, droplet size, emulsifier type, lipid physical state, and droplet aggregation state. This knowledge can be used to design emulsions that can enhance the bioavailability and efficacy of encapsulated hydrophobic bioactives.
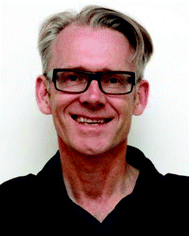 David Julian McClements | David Julian McClements is a Distinguished Professor at the Department of Food Science at the University of Massachusetts. He specializes in the areas of food biopolymers, colloids, and emulsions, with a strong emphasis on the structural design of foods to include their safety, quality, and nutritional aspects. He received his Ph.D. in Food Science (1989) at the University of Leeds (United Kingdom) in ultrasonic spectrometry, and did Post-Doctoral Research at the University of Leeds, University of California and University College Cork. He has written or edited numerous books in the food science area, and has published over 860 research articles, and is currently one of the most highly cited authors in the Agricultural Sciences. He is a fellow of the Royal Society of Chemistry, American Chemical Society (Agricultural and Food Division), and Institute of Food Technologists. |
1. Introduction
Traditionally, the research on food emulsions was primarily concerned with understanding the major factors impacting their physicochemical properties and sensory attributes.1 In particular, researchers focused on establishing relationships between droplet properties (such as concentration, composition, size, charge, and interactions) and the rheology (texture), optical properties (appearance), stability (shelf life), and/or mouthfeel of emulsions. More recently, there has been growing interest in understanding the behavior of emulsions within the human gastrointestinal tract (GIT) after ingestion.2–6 This research is mainly driven by an appreciation that the composition and structure of food emulsions (Fig. 1) can be controlled to improve their nutritional profile or to provide specific health benefits. For example, emulsions can be designed to reduce the total calorie content of foods7 or to increase the bioavailability of lipophilic bioactive agents, such as oil-soluble vitamins, nutraceuticals, and lipids.5,8 Here the term “nutraceuticals” is used to refer to components present in foods that are claimed to have beneficial health effects over and above their normal nutritional role, however, it should be noted that there is some concern about the continued use of this term, since many of these components have not been demonstrated to work in practice.9 Emulsions can also be designed to control the release of lipophilic bioactive agents at different locations within the GIT, which can be used to manipulate hormonal responses, such as hunger, satiety and satiation.10–12 The focus of the current article is on the relationship between the structure/composition of oil-in-water emulsions and their ability to increase the bioaccessibility of lipophilic bioactive agents. The majority of the studies in this area have been carried out using in vitro simulated gastrointestinal tract (GIT) systems, although some studies have been carried out using either animals or humans.
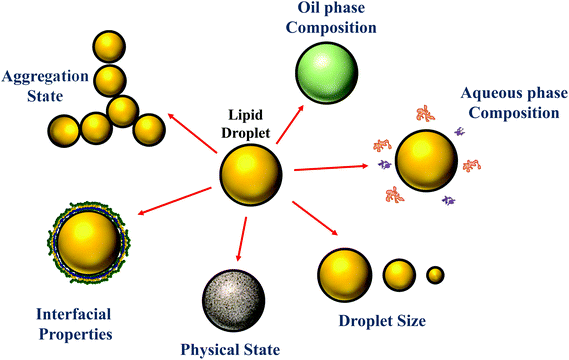 |
| Fig. 1 The properties of the droplets in oil-in-water emulsions can be tailored to alter their susceptibility to lipid digestion and bioactive bioaccessibility. | |
2. Bioaccessibility and bioavailability
The bioaccessibility (B*) can be defined as the fraction of a substance released from an ingested food (or other delivery vehicle) that is present within the gastrointestinal intestinal fluids in a form suitable for absorption.13,14 For lipophilic bioactive agents, this is usually taken to be the fraction of the substance that is solubilized within dietary mixed micelles in the small intestine: |  | (1) |
Here mM is the mass of the substance present in the dietary mixed micelle phase, and mT is the total mass of the substance present in the small intestine. The dietary mixed micelles consist of micelles and vesicles that have hydrophobic domains that can incorporate lipophilic bioactive agents and transport them to the epithelium cells (Fig. 2). The bioaccessibility of a bioactive agent may be less than 100% for a number of reasons: (i) the bioactive may not be fully released from the food matrix; (ii) there are insufficient dietary mixed micelles present to solubilize all the bioactive present; (iii) the bioactive is too large to fit inside the hydrophobic interior of the micelles or vesicles; (iv) the bioactive precipitates or crystallizes; or, (v) the bioactive forms an insoluble complex with some other component.14 In most situations, it is important to increase the bioaccessibility of a bioactive agent so as to increase its beneficial biological effects.15 However, in some cases it may be undesirable to increase the bioaccessibility of harmful bioactive agents in foods, such as toxins or pesticides.16
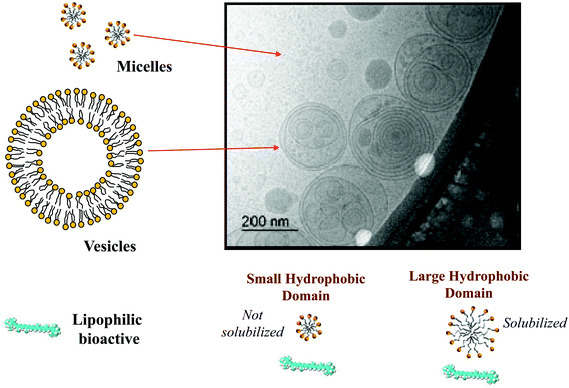 |
| Fig. 2 The TEM image is of mixed micelles formed by linoleic acid and bile salts in aqueous solution. Lipophilic bioactive molecules can be incorporated into the hydrophobic domains within the micelles and vesicles provided they have the correct molecular dimensions. | |
It should also be noted that the total amount of bioactive agent in the small intestine may be less than the amount ingested because of chemical or biochemical transformations that occur in the mouth, stomach, or small intestine.14 As a result, some of the original bioactive may be converted into other molecular forms that are more or less bioaccessible and bioactive than the parent compound. This phenomenon can be described by a transformation (T*) parameter, which describes the fraction of the parent compound remaining after any chemical or biochemical conversions have taken place. In practice, it is the fraction of the ingested bioactive agent that actually reaches the intended site of action within the human body that determines its efficacy, which is referred to as the bioavailability. The bioavailability (BA) depends primarily on three factors, the bioaccessibility, transformation, and absorption: BA = B* T*A*.14 Here, A* is the fraction of the bioaccessible bioactive agent in the gastrointestinal fluids that is actually absorbed by the epithelium cells and reaches the site of action. It is important to highlight that the bioaccessibility and bioavailability may be determined by various methods, including in vitro GIT models, cell culture models, and animal/human feeding studies.17 These models work on different physicochemical and biological principles, which should be taken into account when analyzing the impact of delivery systems on bioaccessibility and bioavailability, as well as comparing data from different studies. Moreover, animal and human feeding studies typically provide information that is much closer to reality than in vitro or cell culture models. Finally, it should be noted that it is actually the bioactivity of an ingested bioactive agent that determines its potential health benefits, which may be determined by measuring specific biomarkers or health outcomes.
In this article, the main focus will be on factors that impact the bioaccessibility of lipophilic bioactive agents, but where appropriate, factors impacting their bioavailability or bioactivity will also be discussed.
3. Emulsion design: physicochemical and structural principles
There is considerable scope for manipulating the properties of food emulsions so as to change the gastrointestinal fate and bioaccessibility of lipophilic bioactives.18,19 In this section, a general overview of some of the most important factors that can be controlled to enhance the bioaccessibility of bioactives is given, with an emphasis on describing the underlying physicochemical and structural principles involved. A review of specific empirical studies on the impact of emulsion properties on the bioaccessibility of lipophilic bioactives is provided in section 4.
3.1. Effect of oil phase composition & amount
Oil-in-water emulsions can be fabricated that have a range of lipid droplet concentrations, ranging from less than 0.1% (e.g., beverages) to more than 50% (e.g., dressings and mayonnaise).1 The concentration of lipid droplets in a food affects the quantity of mixed micelles generated in the small intestine after digestion, which impacts the solubilization capacity of the gastrointestinal fluids.20–22 Emulsions can also be formulated from a variety of different food-grade lipids, including triacylglycerols (TAGs), diacylglycerols (DAGs), monoacylglycerols (MAGs), mineral oils, flavor oils, essential oils, and fat substitutes, which vary in their polarities, solubilization capacities, viscosities, physical states, and digestibilities.1,2 The nature of the oil phase can therefore impact the maximum amount of bioactive agent that can be incorporated into an emulsion, as well as its retention, release, and solubilization within the GIT. Even within a particular class of lipid types, there may be considerable differences in oil phase properties that can alter the bioaccessibility of lipophilic bioactives. For instance, there may be variations in the chain length, degree of unsaturation, and position of the fatty acids on the glycerol backbone in MAGs, DAGs, and TAGs, which will alter the rate and extent of lipid digestion, as well as the size of the hydrophobic domains formed within the mixed micelle phase, and therefore alter their solubilization capacity for specific bioactive agents (section 4.1).
Lipophilic bioactives that are dissolved in non-digestible lipid phases (such as mineral or flavor oils) typically have a much lower bioaccessibility than those dissolved in digestible lipid phases (such as TAGs), which may occur for two reasons.23 First, the lipophilic bioactive agents may remain trapped inside non-digested lipid droplets, rather than being released into the surrounding aqueous phase.20 In this case, the bioactives may only be transferred due to simple diffusion from the droplets to any mixed micelles in the surrounding aqueous phase, which is typically a slow process due to their low water-solubility. Alternatively, the uptake of the bioactives by the GIT may rely on the absorption of the entire undigested lipid droplets, as has been shown for curcumin-loaded lipid droplets incubated on Caco-2 cells.24 Second, if there are no digestible lipids present, then no free fatty acids (FFAs) or MAGs will be generated that can form mixed micelles.25 Consequently, the bioactives can only be solubilized by simple micelles (bile salts and phospholipids), which have a much lower solubilization capacity than mixed micelles.26
The nature of the mixed micelles formed also influences the bioaccessibility of lipophilic bioactives.23 The mixed micelle phase may contain a variety of different colloidal structures, including micelles, vesicles, and liquid crystals, depending on the nature of the oil phase and other components present.27 Moreover, the hydrophobic domains within these colloidal structures may vary in dimensions depending on the chain length and unsaturation of the fatty acids in the oil phase (Fig. 2). The width of the hydrophobic domains is approximately equal to twice the length of the fatty acid chains.13 Thus, the size of the hydrophobic domains tends to increase with increasing chain length, and be larger for saturated (straight) than for unsaturated (bent) chains. If the lipophilic bioactive agent is too large to be accommodated within the hydrophobic domains in the mixed micelles, then it may not be solubilized, which leads to a lower bioaccessibility. This effect is commonly seen with carotenoids, where they are easily solubilized by mixed micelles formed from long chain fatty acids, but not from those formed from short or medium chain fatty acids.23,28 The average molecular weight, number of carbon atoms, and degree of unsaturation of a number of important digestible food lipids are shown in Table 1.
Table 1 Average molecular properties (number of carbon atoms and double bonds) of the fatty acid chains and molecular weights of the triglycerides (TAGS) in different kinds of common food oils
Oil type |
Average carbon atoms |
Average double bonds |
TAG MW |
Palm oil |
17.1 |
0.7 |
849.7 |
Peanut oil |
17.9 |
1.1 |
878.1 |
Sunflower oil |
17.9 |
1.5 |
875.2 |
Soybean oil |
17.8 |
1.5 |
871.6 |
Olive oil |
17.8 |
0.9 |
874.9 |
Corn oil |
17.7 |
1.3 |
868.8 |
MCT |
8.9 |
0.0 |
509.2 |
Fish oil |
17.8 |
2.2 |
868.2 |
An estimate of the dimensions of the hydrophobic domains in micelles and vesicles formed from saturated fatty acids can be obtained from experimental measurements of the increase in the size of the hydrophobic domains in lipid bilayers with increasing fatty acid chain length.29–31 Experiments have shown that the hydrophobic domain in phospholipid bilayers increases by about 0.19 nm per additional carbon atom on the fatty acid chains.31 Thus, to a first approximation, the dimensions of the hydrophobic domains (D) in mixed micelles can be given by:
Here, D is approximately equal to the length of two fatty acid chains and n is the number of carbon atoms per fatty acid chain. The dimensions of the hydrophobic domains in mixed micelles should therefore increase from about 1.5 to 4.6 nm as the fatty acid chain length increases from 8 to 24. For the sake of comparison, the length of β-carotene, a well-studied lipophilic food-grade nutraceutical is around 2.5 nm based on its unit cell crystal structure.32 Consequently, it would be expected to be solubilized in mixed micelles formed from relatively long-chain fatty acids (C > 14), but not in those with shorter chains, which is consistent with published data.23,28 For unsaturated fatty acids, this relationship would be expected to be somewhat different because the chains are kinked, and therefore pack differently in micelles and vesicles. Indeed, experimental measurements have shown that the dimensions of hydrophobic domains in phospholipid bilayers are slightly smaller for monounsaturated fatty acids (C18:1) than for saturated fatty acids with the same chain length (C18:0).33 For the same number of carbon atoms, unsaturated fatty acids have a greater cross-sectional area but shorter end-to-end length than saturated fatty acids. However, an increase in the dimensions of the hydrophobic domains with increasing chain length is still observed for unsaturated lipids.31
3.2. Effect of aqueous phase composition
The aqueous phase used to fabricate oil-in-water emulsions may contain a variety of components that could potential impact the bioaccessibility of encapsulated lipophilic bioactive agents. The aqueous phase may contain minerals, polyols, sugars, polysaccharides, peptides, proteins, and surfactants.1 These components may alter the bioaccessibility by altering droplet properties in the GIT (such as interfacial properties or aggregation state), by interfering with digestive enzymes, by interacting with other components involved in digestion (such as bile salts or calcium ions), or by changing the physicochemical properties of the gastrointestinal fluids (such as viscosity or gelation). Recent studies on the impact of various aqueous phase components on lipid digestion and bioaccessibility are reviewed in section 4.2.
3.3. Effect of droplet size
Oil-in-in-water emulsions can be prepared that have a range of different droplet sizes.1 Mean droplet diameters may range from less than 50 nm to greater than 500 μm depending on the ingredients and homogenization method used to prepare an emulsion.1 Emulsions may also be formed from ingested bulk lipids within the mouth during oral processing, which may contain relatively large oil droplets. In addition, the particle size distribution may be narrow or broad, as well as monomodal or multimodal. The size of the droplets in an emulsion may alter the bioaccessibility of lipophilic bioactives through numerous mechanisms.
The rate of release of a bioactive component from an oil droplet due to simple diffusion increases with decreasing droplet size, since the bioactive then has a shorter distance to travel. To a first approximation, the time required for the concentration of a bioactive agent originally present within an oil droplet to decrease by 50% can be predicted using the following expression:1
| 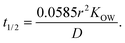 | (3) |
Here, r is the lipid droplet radius, KOW is the oil–water partition coefficient, and D is the translational diffusion coefficient of the bioactive molecules through the oil phase. This equation assumes that the bioactive-loaded lipid droplets are placed into a well-stirred aqueous phase that initially contains no bioactive agent. Predictions made using this equation indicate that bioactives may be released from oil droplets into the surrounding aqueous phase relatively quickly when they have a low hydrophobicity (log
P = 2) but relatively slowly when they have a high hydrophobicity (log
P = 8) (Table 2).
Table 2 Predicted release times of hydrophobic bioactive molecules from oil droplets: impact of droplet size and partition coefficient (log
P)
|
Release time (t1/2) |
Droplet radius |
Log P = 2 |
Log P = 4 |
Log P = 6 |
Log P = 8 |
0.1 μm |
<0.01 s |
0.015 s |
1.5 s |
2.4 min |
1 μm |
0.015 s |
1.5 s |
2.4 min |
4.1 hours |
10 μm |
1.5 s |
2.4 min |
4.1 hours |
17 days |
100 μm |
150 min |
4.1 hours |
17 days |
4.6 years |
More importantly, the rate of lipid digestion typically increases as the droplet size decreases because then there are more sites available at the oil–water interface where lipase molecules can adsorb and catalyze hydrolysis.34–37 The specific surface area (AS) of a lipid droplet increases as the droplet size decreases according to the following expression:
|  | (4) |
Here ϕ is the disperse phase volume fraction of the lipid phase in an emulsion, and r32 is the surface-weighted mean droplet radius. The rate and extent of lipid digestion is important because a lipophilic bioactive agent may not be released from the oil droplets until the lipid phase is digested.20 In addition, the lipid digestion products produced interact with bile salts and phospholipids from the GIT to form mixed micelles that solubilize and transport the released bioactive agents.38 Consequently, the rate and extent of lipid digestion may play an important role in determining bioaccessibility. If the lipid phase is not fully digested, then the bioactive agent may remain trapped inside the droplets, rather than being released into the mixed micelles. It is therefore possible to alter lipid digestion and bioaccessibility by altering the size of the droplets in emulsions (section 4.3). It should be noted that bioactives loaded into undigested lipid droplets may be absorbed if the entire droplets can pass through the mucus layer and be absorbed by the epithelium cells. This process is likely to be slower than that of the solubilization-mediated process,39 but it is also likely to depend on droplet size since the absorption of particles by epithelium cells is known to depend on their dimensions.
A mathematical model was proposed to describe the impact of droplet size on the rate and extent of lipid digestion in the small intestine, which was based on the assumption that the individual lipid droplets shrank during digestion because some of the triacylglycerols were hydrolyzed and lost.35 There was a mistake in the original derivation of this model, which was corrected by Gaucel and co-workers,37 leading to the following expression:
| 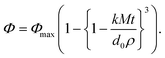 | (5) |
Here, Φ is the fraction of the lipid that has been digested by time t, Φmax is the fraction of lipid that has been digested by the end of the digestion process, k is a rate constant, M is the molar mass of the digestible lipids, d0 is the initial lipid droplet diameter, and ρ is the density of the lipid phase. In practice, this equation has to be modified slightly to ensure that the term in the curly brackets does not become less than zero so as to avoid obtaining non-physically realistic results at longer digestion times. This equation can be fit to experimental FFA versus time data to obtain values of Φmax and k for the system studied. As expected, the equation predicts that the rate of lipid digestion increases as the initial diameter of the lipid droplets (in the small intestine) decreases because of the increase in surface area exposed.
3.4. Effect of droplet physical state
The droplets in oil-in-water emulsions can be made from lipid phases that are liquid, semi-solid, or solid at body temperature.1 Studies have shown that solid or semi-solid lipid droplets are digested more slowly than liquid lipid droplets under simulated small intestine conditions.40,41 This effect may occur because the highly ordered nature of the crystalline structure makes it more difficult for lipase to access the ester bonds in the TAGs at the surfaces of the oil droplets (Fig. 3).40 Alternatively, it may occur because partially crystalline lipid droplets are prone to a phenomenon called partial coalescence, which leads to the formation of large clusters of aggregated lipid droplets in the gastrointestinal tract.41 As a result, it is more difficult for lipase molecules in the gastrointestinal fluids to access the surfaces of the lipid droplets in the interior of the aggregates. It may therefore be possible to control lipid digestion and bioaccessibility by altering the physical state of the lipid droplets in an emulsion (section 4.4).
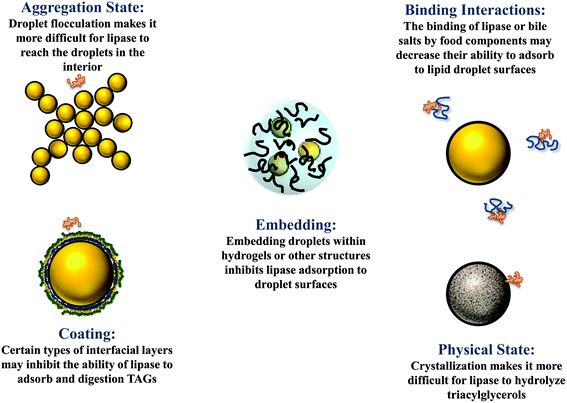 |
| Fig. 3 Some possible physicochemical mechanisms leading to retarded lipid digestion and bioaccessibility. | |
3.5. Effect of interfacial properties
The lipid droplets in oil-in-water emulsions can be coated by different kinds of emulsifiers and other substances that alter their interfacial properties, such as thickness, rheology, surface activity, and charge.1 The nature of the interfacial region surrounding the lipid droplets may have a major impact on their susceptibility to lipid digestion through numerous mechanisms (Fig. 3). If the droplets are coated by a highly surface-active emulsifier, then it may be difficult for the bile salts and lipase to displace the original emulsifier and access the lipid droplets.42 Similarly, if the droplets are coated by an interfacial layer that is strongly cross-linked, it may also be difficult for it to be displaced by bile salts and lipase.43 The properties of the interfacial layer, such as charge, thickness, and hydrophobicity, will also influence the aggregation state of the droplets, which can also impact lipid digestion. It is therefore possible to control lipid digestion and bioaccessibility by manipulating the properties of the interfacial layer around the oil droplets (section 4.5).
It is often difficult to quantify the potential effects of the interfacial layer on lipid digestion because of a lack of information about interfacial properties in different regions of the GIT, and because there are numerous types of surface-active molecules present in the gastrointestinal fluids. However, some insights can be gained into the potential for highly surface-active emulsifiers to inhibit lipid digestion using simple mathematical models. Consider an oil-in-water emulsion that contains two types of surface-active substances, the original emulsifier and bile salts from the surrounding aqueous phase. At equilibrium, the interfacial concentrations of these two types of surface-active substances depend on their surface activities and concentrations, and can roughly be described by the following expression:44
| 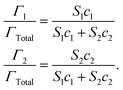 | (6) |
Here, Γ, c and S are the excess interfacial concentration, bulk concentration, and surface-activity of the surface-active substances, and the subscripts 1 and 2 refer to the original emulsifier and bile salts, respectively. The surface-activity can be taken as the concentration of the surface-active substance where the interfacial pressure is half of its saturation value (S = 1/c½) in a single-species system. This equation is based on a number of assumptions that are not typically true in practice, such as that the surface-active substances bind reversibly, form an ideal mixture, and have similar molecular dimensions.45,46 Nevertheless, these equations do provide some useful insights into the potential ability of certain types of emulsifiers to inhibit the adsorption of bile salts. For instance, the change in the relative proportions of emulsifier and bile salts at lipid droplet surfaces as a function of bile salt concentration is shown in Fig. 4. As the bile salt concentration increases, an increasing amount of the original emulsifier is displaced from the droplet surfaces. However, the greater the surface activity of the original emulsifier, the more difficult it is to replace. More comprehensive mathematical theories have been developed that account for surface-active substances with different dimensions, interactions, and that can undergo structural changes.45–48 Application of these theories may provide some useful insights into designing emulsions that can inhibit or promote lipid digestion and bioaccessibility.
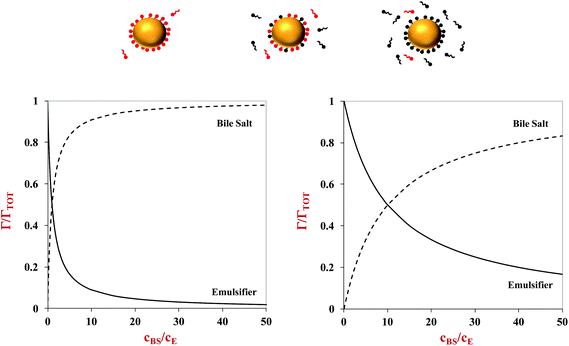 |
| Fig. 4 Predictions of the displacement of the original emulsifier (E) from lipid droplet surfaces by bile salts (BS) in the small intestine. It is assumed that the emulsifier and bile salts have similar surface-activities (SE = 1; SBS = 1) in the left hand figure, and that the emulsifier is much more surface-active than the bile salts (SE = 0.1; SBS = 1) in the right hand figure. The amount of emulsifier displaced from the droplet surfaces increases as its surface activity increases. | |
3.6. Effect of droplet aggregation state
The droplets in oil-in-water emulsions may be evenly distributed throughout the system or they may form clusters (flocs) depending on the nature of the colloidal interactions acting between them.1 When the attractive interactions (such as van der Waals, depletion, bridging, and hydrophobic) dominate the repulsive interactions (such as steric and electrostatic), then the droplets tend to flocculate. Conversely, when the repulsive interactions outweigh the attractive interactions, they tend to exist as individual droplets. The aggregation state of droplets may have a major impact on the rate and extent of lipid digestion, which can affect the bioaccessibility of bioactive agents.41,49 It is more difficult for lipase and bile salts to access the surfaces of lipid droplets when they are trapped within the interior of flocs, which may inhibit lipid digestion (Fig. 3). In addition, it is more difficult for the free fatty acids and monoacylglycerols generated at the lipid droplet surfaces to move into the aqueous phase, which may also inhibit further lipid digestion. At present, there is a relatively poor quantitative understanding of how the structure and properties of flocs impact the rate and extent of lipid digestion.
The fraction of droplets that are flocculated, the size and structure of the flocs formed, and the strength of the bonds holding the droplets together within the flocs, are all likely to play an important role in determining the impact of droplet aggregation on lipid digestion. Again, it is important to point out that it is the nature of the flocs formed in the region of the GIT where lipid digestion and bioactive release occurs that will be important, rather than the original aggregation state.
3.7. Effect of droplet encapsulation in matrices
In some types of structured emulsions, the oil droplets are trapped inside larger structures such as microscopic or macroscopic hydrogels (Fig. 3).50–52 In this case, the oil droplets may have to be released from the hydrogels before they can be digested. Alternatively, gastrointestinal components (such as bile salts, proteases, amylases and lipases) may have to diffuse through the hydrogel matrix before they can access the lipid droplets.53 The rate of lipid digestion may then depend on changes in the properties of the hydrogels within the GIT, such as changes in their pore size, interactions, or disintegration.54
Some attempts have been made to quantify the potential effects of hydrogel matrix properties on the digestion of lipids, which would be expected to impact the bioaccessibility of encapsulated bioactive components. For instance, the following expression can be used to describe the release of small particles (or molecules) from spherical hydrogel beads:54,55
|  | (7) |
Here, Φ is the fraction of the small particles diffusing out of the hydrogel beads after a certain time t, M(t) and M(∞) are the concentrations of the small particles in the hydrogel beads at time t and when equilibrium has been reached, n is an integer, a is the radius of the hydrogel beads, and Dgel is the diffusion coefficient of the small particles through the hydrogel beads. The diffusion of small particles through polymer networks can be described using the following equation:56,57
| 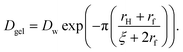 | (8) |
Here, Dw is the translational diffusion coefficient of the small particles through pure water, rH is the hydrodynamic radius of the small particles, rf is the cross-sectional radius of the polymer chains that make up the interior of the hydrogel beads, and ζ is the diameter of the pores in the hydrogel network. The translational diffusion coefficient of the small particles through pure water can be approximated using the following equation:
Here, kB is Boltzmann's constant, T is absolute temperature, and η is the viscosity of the water.
The same equations can simply be adopted to predict the fraction of small particles that diffuse into a hydrogel bead. The small particles considered by these equations could be lipid droplets, digestive enzymes (such as lipase or protease), bile salts, or mixed micelles. These equations can therefore be used to predict the impact of hydrogel bead properties and small particle properties on lipid digestion and bioaccessibility. For instance, they could be used to examine the release of lipid droplets encapsulated in hydrogel beads, or to examine the penetration of lipase into hydrogel beads. These equations predict that the release rate of small particles from hydrogel beads decreases as the bead dimensions increase, the small particle dimensions increase, and the hydrogel pore size decreases.54 This information can then be used to design hydrogel beads for specific applications. Typically, transport of small particles is relatively fast when they have dimensions less than the pore size of the hydrogel network, but is relatively slow otherwise. These equations have been used to interpret the impact of pore size on the release of curcumin-loaded lipid droplets from alginate hydrogel beads under simulated GIT conditions.54,58 The experiments showed that a slower rate of droplet release occurred when the beads contained higher levels of alginate, which was attributed to the decrease in small pore size relative to the droplet size.
4. Review of recent research
In this section, an overview of recent research on the design of emulsions to enhance the bioaccessibility of lipophilic bioactives is given.
4.1. Effect of oil phase composition & amount
One of the earliest studies in this area, showed that the bioaccessibility of carotenoids loaded in phospholipid-stabilized emulsions increased with increasing fat content, and depended on the fatty acid composition of the lipid phase,59 which was attributed to changes in the solubilization capacity of the dietary mixed micelle phase. Since this early work, there have been numerous other studies on the impact of oil phase type and amount on the bioaccessibility of various lipophilic bioactive agents. A comprehensive in vitro study of the influence of oil phase composition on the bioaccessibility of carotenoids was recently carried out using a simulated GIT model.60 In this study, the carotenoids were originally present within different kinds of fruits or vegetables (e.g., carrot, papaya, or spinach), and oil-in-water emulsions were formed in the gastric phase by adding an oil phase and blending (no particle size control). The authors showed that the bioaccessibility of the carotenoids increased as the oil content increased up to about 2.5% (Fig. 5), which was attributed to a greater level of dietary mixed micelles being available to solubilize them. The authors also reported that the type of oil phase used impacted carotenoid bioaccessibility. For example, the bioaccessibility of β-carotene in carrots was 22.6, 24.0, 22.6, 16.0, 15.0 and 7.8% for olive, soybean, sunflower, peanut, palm and coconut oils at a lipid phase level of 2.5%, respectively. Similar trends were seen for the other types of fruits and vegetables and carotenoids studied. The coconut oil (which mainly contains MCT) consistently gave a lower bioaccessibility than the other oils, which can be attributed to the relatively short hydrocarbon chain length of the fatty acids in this type of oil (Table 1). Consequently, the dietary mixed micelles formed after lipid digestion have relatively small hydrophobic domains that are unable to incorporate the long carotenoid molecules (Fig. 2). The palm and peanut oils gave a lower bioaccessibility than other oils containing long chain triglycerides, however, the origin of this effect is currently unknown.
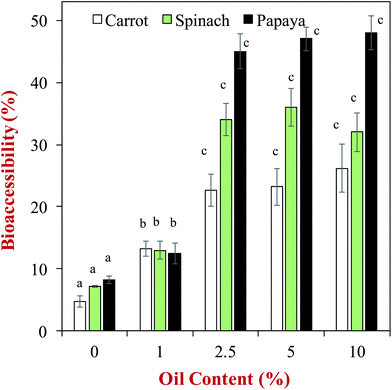 |
| Fig. 5 Impact of olive oil content on the bioaccessibility of carotenoids from different fruits and vegetables. Emulsions were formed by blending the olive oil with the produce (no particle size control). The letters above the data show significant differences between oil contents on the same produce (p < 0.05). Data adopted from Table 3 of Mashurabad et al. (2017).60 | |
Table 3 Examples of studies of the impact of emulsion properties on the bioaccessibility (B*) and/or bioavailability (BA) of selected nutraceuticals and vitamins. Key: soybean soluble polysaccharides = SSP; decaglycreol monolaurate = DM
Bioactive |
Emulsion system |
Main findings |
Analytical methods |
Ref. |
Oil level
|
α- and β-carotene |
Oil: corn oil |
The bioaccessibility of carotenoids in carrots increased when fat content was increased from 0 to 8% |
In vitro GIT model |
23
|
Surfactant: whey protein |
Diameter: 140 nm |
Carotenoids |
Oil: olive oil |
The bioaccessibility of carotenoids in tomatoes increased when fat content was increased from 0 to 4% |
In vitro GIT model |
57
|
Surfactant: whey protein |
Diameter: 146 nm |
Carotenoids |
Oil: corn oil |
The bioaccessibility of carotenoids in mangoes increased when fat content was increased from 0 to 4% |
In vitro GIT model |
58
|
Surfactant: Tween 20 |
Diameter: 145–188 nm |
Carotenoids |
Oil: corn oil |
The bioaccessibility of carotenoids in yellow peppers increased when fat content was increased from 0 to 4% |
In vitro GIT model |
59
|
Surfactant: Tween 20 |
Diameter: 140–180 nm |
Oil type
|
β-Carotene |
Oil: corn oil, MCT, & orange oil |
Carotenoid bioaccessibility decreased: corn oil ≫ MCT > Orange oil |
In vitro GIT model |
21
|
Surfactant: Tween 20 |
Diameter: 140–170 nm |
Fucoxanthin |
Oil: corn oil, MCT, & orange oil |
Carotenoid bioaccessibility decreased: corn oil > MCT > Orange oil |
In vitro GIT model |
61
|
Surfactant: Tween 20 |
BA: corn oil > MCT ≈ Orange oil |
In vivo (rat gavage) |
Diameter: 200–250 nm |
|
|
Carotenoids |
Oil: corn oil |
Carotenoid bioaccessibility decreased: corn oil > MCT > Orange oil |
In vitro GIT model |
59
|
Surfactant: Tween 20 |
Diameter: 140–180 nm |
Vitamin E |
Oil: corn oil & MCT |
Vitamin E bioaccessibility was higher for corn oil than MCT, but Caco-2 cell absorption was similar for corn oil and MCT |
In vitro GIT model |
63
|
Surfactant: Quillaja saponin |
Caco-2 Cells |
Diameter: 140–180 nm |
|
Droplet size
|
β-Carotene |
Oil: corn oil |
The bioaccessibility increased with decreasing droplet size |
In vitro GIT model |
107
|
Surfactant: Tween 20 |
Diameter: 200, 400 & 23 000 nm |
β-Carotene |
Oil: corn oil |
The bioaccessibility increased with decreasing droplet size |
In vitro GIT model |
108
|
Surfactant: caseinate |
Diameter: 124–368 nm |
Vitamin E |
Oil: sunflower oil |
The smaller droplets gave a higher maximum in vitamin E plasma level, and shorter time to reach maximum |
In vivo (rat gavage) |
115
|
Surfactant: Quillaja saponin |
Diameter: 270 & 1285 nm |
Vitamin D2 |
Oil: corn oil |
The smaller droplets gave a higher in vitro bioaccessibility, but larger droplets gave higher in vitro serum levels |
In vitro GIT model |
114
|
Surfactant: Tween 80 |
In vivo (rat gavage) |
Diameter: 112, 530 & 14 500 nm |
|
Emulsifier type
|
β-Carotene |
Oil: MCT |
The release of β-carotene decreased in the following order whey protein > DM > SSPS |
In vitro GIT model |
123
|
Surfactant: whey protein, soybean soluble polysaccharides, decaglycreol monolaurate |
Diameter: 200–700 nm |
β-Carotene |
Oil: MCT |
The bioaccessibility of β-carotene was higher for the conjugate that the protein alone |
In vitro GIT model |
126
|
Surfactant: protein versus protein–polyphenol–carbohydrate conjugate |
Diameter: 200–300 nm |
β-Carotene |
Oil: sunflower oil |
The bioaccessibility of β-carotene was fairly similar for the three systems |
In vitro GIT model |
148
|
Surfactant: soy glycinin nanoparticles (SGN), sodium caseinate or whey protein |
Diameter: 1800 nm for SGN system |
Lutein |
Oil: corn oil |
The bioaccessibility of lutein was similar for the conjugates and protein alone |
In vitro GIT model |
127
|
Surfactant: protein versus protein–carbohydrate conjugate |
Diameter: 180 nm |
Curcumin |
Oil: vegetable oil |
The bioaccessibility of curcumin was fairly similar for the KPN and Tween 80 stabilized systems |
In vitro GIT model |
149
|
Surfactant: kafirin protein nanoparticles (KPN) and Tween 80 |
Diameter: 49 000 and 7000 nm |
The bioaccessibility of carotenoids (α- and β-carotene) from raw and cooked carrots has also been shown to increase as the oil content in emulsions mixed with them prior to exposure to the GIT was increased.26 Similar results have been obtained for emulsions mixed with other types of fruits and vegetables, including tomatoes,61 mangoes,62 and peppers.63 Again, these effects can be attributed to the greater number of dietary mixed micelles formed after digestion of the lipid phase in the emulsions.64
Numerous other studies have shown that the bioaccessibility of carotenoids depends on the nature of the oil phase used to prepare emulsion-based delivery systems. The bioaccessibility of β-carotene was negligible (≈0%) for orange oil, very low for MCT (≈2%), and relatively high for LCT (≈66%) when loaded into O/W nanoemulsions (Fig. 6).23In vitro and in vivo studies have shown that the bioaccessibility of another carotenoid (fucoxanthin) decreased in the same order when delivered in nanoemulsions prepared from different oils: orange oil < MCT < LCT.65 The bioaccessibility of β-carotene has been shown to increase when the ratio of LCT-to-MCT in the oil phase of emulsions and nanoemulsions was increased.28,66
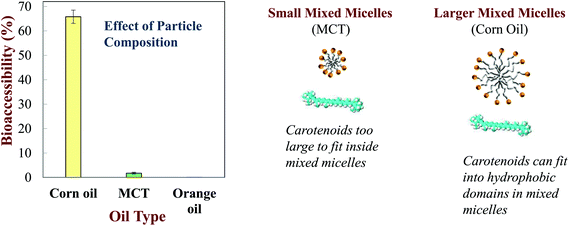 |
| Fig. 6 Impact of oil phase type on the bioaccessibility of b-carotene nanoemulsions made from LCT (corn oil), MCT (medium chain triglycerides) or indigestible (orange oil) oils (Qian et al. 2012).161 | |
The nature of the oil phase (MCT vs. LCT) may also impact the chemical transformation and absorption of lipophilic bioactive agents encapsulated within emulsions. For instance, a recent study examined the impact of lipid phase type on the bioaccessibility, absorption, and transformation of vitamin E and vitamin E tocopherol.67 This study showed that the bioaccessibility of vitamin E was higher for emulsions formulated from LCT (46%) than from MCT (19%), but that the intestinal epithelial permeability of vitamin E determined using Caco-2 cells was similar for both oils (28–30%). It also showed that the transformation of vitamin E tocopherol to vitamin E was higher when delivered in emulsions fabricated from LCT than from those fabricated from MCT.
A kinetic model has recently been developed to relate the kinetics of lipid digestion and mixed micelle formation to carotenoid bioaccessibility.68 The authors measured the reduction in the levels of triacylglycerols (TAGs) in the system during digestion, as well as the increase in the levels of monoacylglycerols (MAGs) and free fatty acids (FFAs). They also measured the increase in the fraction of the MAGs, FFAs and carotenoids incorporated into the mixed micelle phase throughout lipid digestion. They then fitted the data with a mathematical model to determine rate constants for the various processes occurring (Fig. 7):
| X(t) = Xf + (Xi − Xf) exp(−kt) | (10) |
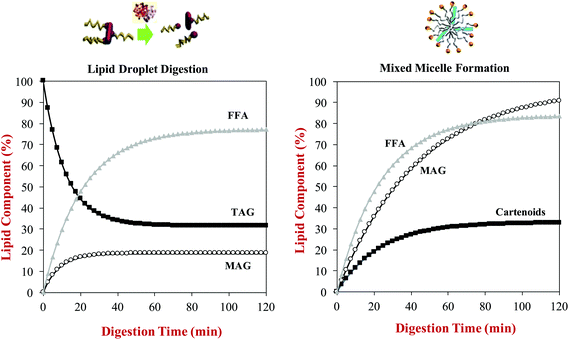 |
| Fig. 7 Predictions of: (a) The rate of triacylglycerol (TAG) hydrolysis and free fatty acid (FFA) and monoacyglycerol (MAG) generation during lipid digestion; (b) The rate of incorporation of FFA, MAG and carotenoid incorporation into mixed micelles during lipid digestion. Data predicted for β-carotene-loaded olive oil- in-water emulsions during digestion in a simulated GIT from information provided by Mutsokoti et al. (2017).68 | |
Here, X(t) is the concentration of the component (TAG, MAG, FFA or carotenoids) at time t, Xi is the initial concentration, Xf is the final concentration, and k is the rate constant. The application of this model is useful for quantifying the impact of various factors on lipid digestion and carotenoid bioaccessibility. For example, the rate of micelle incorporation was slower for more hydrophobic carotenoids, and depended on their isomeric form with the cis-form being incorporated into mixed micelles more rapidly than the trans-form. The origin of this later effect was attributed to the fact that the cis-form has smaller molecular dimensions, and is therefore easier to incorporate into the hydrophobic domains in the mixed micelles. This model also showed that there was a linear relationship between the extent of lipid digestion and carotenoid bioaccessibility, highlighting the importance of the lipid digestion step in determining bioaccessibility.
The incorporation of sufficiently high levels of oleogelators (such as ethylcellulose) into the oil phase of emulsions has been shown to decrease lipid digestion and carotenoid bioaccessibility using in vitro GIT models.69 This effect may have occurred because the oleogelators were indigestible and formed a three-dimensional network throughout the oil phase, and therefore they may have prevented the lipase from accessing the TAG molecules.
In summary, numerous studies have shown the importance of lipid phase type and concentration on the bioaccessibility of lipophilic bioactive agents, which was mainly attributed to changes in the amount and dimensions of the hydrophobic domains in the dietary mixed micelles formed, since these parameters determine their solubilization capacity. In future studies, it would be useful to further investigate the relationship between fatty acid composition of digestible lipids and the dimensions of the hydrophobic domains formed within the mixed micelles. A recent example of this approach is the utilization of synchrotron small angle X-ray scattering (SAXS) to characterize the structure of the colloidal structures formed during lipid digestion.70 This study showed that both micelles and vesicles were generated during the lipolysis of emulsified triacylglycerols (triolein), and that the size of these colloidal structures increased during digestion. In a related study, SAXS was used to monitor the properties of the colloidal structures formed during the digestion of mayonnaise.71 This study suggested that a range of colloidal structures were formed from the initial lipid droplets, including liquid crystals, vesicles, and micelles. Neutron scattering methods have also been used to provide information about the dimensions of mixed micelles before and after incorporation of lipid digestion products.72 This study showed that the mixed micelles formed had cylindrical structures that increased in length as lipid digestion proceeded due to incorporation of lipid digestion products. A combination of SAXS and neutron scattering was used to investigate the impact of lipid type (short, medium or long chain fatty acids) on the colloidal structure of model dietary mixed micelles.73 The size of the mixed micelles increased as the chain length of the fatty acids increased, which would be expected to increase their ability to solubilize lipophilic bioactive molecules. In future, it would be useful to apply these powerful scattering and microscopy techniques to help further understand the relationship between oil type, digesta structure, and bioaccessibility for different types of bioactive agents. This information could then be used to select the most appropriate lipid phase composition for formulating emulsion-based delivery systems.
4.2. Effect of aqueous phase composition
A range of different water-soluble or water-dispersible food components are often incorporated into the aqueous phases of oil-in-water emulsions to alter their physicochemical or sensory properties, including flavors, sugars, salts, alcohols, polysaccharides, proteins, and phospholipids.1 Some of these components may impact the rate and extent of lipid digestion and bioaccessibility. In this section, recent research on some common aqueous phase components on the bioaccessibility of lipophilic bioactives in emulsions is reviewed.
Dietary fibers.
Dietary fibers may be added to emulsions as functional ingredients to alter their physicochemical properties (such as thickening agents, gelling agents, or stabilizers) or their nutritional properties (such as prebiotics, laxatives, or cholesterol reducers). Many researchers have therefore focused on the impact of dietary fibers on lipid digestion and bioaccessibility in emulsions. A recent study showed that the addition of dietary fibers extracted from mandarins increased the bioaccessibility of β-carotene encapsulated in Tween 20-stabilized oil-in-water emulsions.74 In contrast, the addition of methyl cellulose was found to decrease the bioaccessibility of β-carotene encapsulated in whey protein-coated lipid droplets trapped in starch hydrogels.75 Studies have also shown that the type and amount of pectin added to oil-in-water emulsions impacts β-carotene bioaccessibility.76–78 Another study that examined the impact of pectin type and concentration on the bioaccessibility of carotenoids in emulsions focused on identifying the different physicochemical mechanisms involved, such as bile salt binding and viscosity enhancement.79 These researchers reported that the addition of pectin to the emulsions could either increase or decrease carotenoid bioaccessibility depending on the type and level of pectin used. The addition of beet pectin to whey protein-stabilized emulsions was reported to decrease the bioaccessibility of encapsulated β-carotene.80 Another study showed that addition of chitosan to soy protein-stabilized emulsions reduced lipid digestion and carotenoid bioaccessibility, whereas addition of alginate had little effect.81 Addition of κ-carrageenan to Tween 80-stabilized emulsions has also been shown to decrease carotenoid bioaccessibility.82,83
In summary, previous studies have shown that dietary fibers can either increase or decrease lipid digestion and bioaccessibility depending on the nature of the system studied. Presumably, the different effects of dietary fibers can be attributed to the fact that they can impact the gastrointestinal fate of emulsions by many different mechanisms. First, they may thicken or gel the aqueous gastrointestinal fluids, thereby slowing down molecular diffusion and inhibiting thorough mixing.84 As a consequence, it may be more difficult for GIT components (such as bile salts and digestive enzymes) to access the lipid droplet surfaces. Second, the dietary fibers may form a coating around the lipid droplets (e.g., due to electrostatic attraction) that inhibits the ability of bile salts and digestive enzymes to attach to the droplet surfaces, thereby decreasing the rate of lipid digestion. This effect has been reported in emulsions where anionic alginate molecules formed a coating around cationic lactoferrin-stabilized lipid droplets.85 Third, the dietary fibers may change the aggregation state of the lipid droplets, either promoting or inhibiting droplet flocculation depending on the molecular characteristics of the dietary fibers and emulsifiers used.49,86–90 Typically, flocculated droplets are digested more slowly than free droplets because it is harder for bile salts and digestive enzymes to reach the surfaces of the droplets within the floc interior (Fig. 3). Consequently, dietary fibers that promote flocculation (e.g., through a bridging or depletion mechanism) tend to inhibit lipid digestion.49,91 Conversely, dietary fibers that inhibit droplet flocculation in appropriate regions of the GIT (e.g., by forming a protective coating around them) may enhance lipid digestion.89,92 Fourth, dietary fibers may interfere with lipid digestion by binding to GIT components, such as bile salts, digestive enzymes, or mixed micelles.79,93 For example, if a fraction of the lipase molecules in a system binds to a dietary fiber then there may be less available to interact with the lipid droplets and hydrolyze the triacylglycerols (Fig. 3). Similarly, if a fraction of the calcium ions in the system binds to a dietary fiber, then they may not be available to form insoluble soaps with the long chain FFAs released by lipid digestion, thereby inhibiting further digestion.94
Starches.
Digestible polysaccharides (starch) have also been shown to impact the rate and extent of lipid digestion and bioaccessibility of emulsions.95–97 For example, the incorporation of protein-coated lipid droplets into starch hydrogels was shown to increase the bioaccessibility of β-carotene, which was attributed to the ability of the starch to inhibit droplet flocculation in the stomach and small intestine.96,97 Experiments with a model custard, which consisted of genistein-loaded milk fat droplets dispersed in an aqueous solution, indicated that genistein bioaccessibility was appreciably larger when starch was used as a thickening agent rather than carboxymethylcellulose.98 Researchers have also examined the impact of calcium on the bioaccessibility of β-carotene encapsulated in lipid droplets stabilized by modified starch-based emulsifiers.99 It was shown that addition of calcium ions promoted lipid digestion, but reduced β-carotene bioaccessibility, which was attributed to their ability to bind to free fatty acids and bile salts.
Proteins.
Oil-in-water emulsions often contain appreciable levels of proteins in the aqueous phase surrounding the lipid droplets, which may alter lipid digestion and bioaccessibility.100,101 Typically, proteins are fully or partially digested in the stomach and small intestine by gastric and pancreatic proteases within the gastrointestinal fluids. In addition, the proteins and peptides may be susceptible to aggregation within different regions of the GIT, or they may adsorb to the lipid droplet surfaces and alter their interfacial composition. Consequently, it is important to understand how different proteins impact lipid digestion and bioaccessibility. Recently, studies have been carried out on the impact of protein-rich food matrices (milk, cheese, and yogurt) on lipid digestion.102 This study showed that lipid digestion was slower for solid-matrices (cheese) than for fluid matrices (milk and yogurt). Studies have shown that protein-coated lipid droplets were digested more slowly than surfactant-coated ones, which was attributed to the fact that the protein-coated droplets aggregated extensively when exposed to simulated gastric conditions, thereby reducing the ability of the lipase to reach the lipid droplets in the floc interiors.89 Studies have also shown that the type of protein at the lipid droplet surfaces impacts the rate of lipid digestion, e.g., gliadin < caseinate ≈ whey protein.103 The type of protein-based emulsifier used (β-lactoglobulin, α-lactalbumin, or lactoferrin) has also been shown to impact the bioaccessibility of β-carotene, with β-lactoglobulin giving the highest bioaccessibility.104 The utilization of protein-based emulsifiers (caseinate and whey protein) rather than surfactant-based ones (Tween 80) was shown to increase the concentration of curcumin in the mixed micelle phase after lipid digestion, which was attributed to their ability to retard the chemical degradation of curcumin.105 Selection of appropriate types and amounts of adsorbed and non-adsorbed proteins may therefore be useful for modulating lipid digestion and bioaccessibility.
Surfactants.
Oil-in-water emulsions often contain various natural or synthetic low-molecular weight surfactants in the aqueous phase surrounding the droplets.1 These surfactants may be present as individual molecules, as micelles, or bound to other substances. The bioaccessibility of carotenoids in oil-in-water emulsions has been reported to increase when phospholipids were added to the aqueous phase, presumably because they become part of the mixed micelle phase and therefore increased its solubilization capacity.106 Conversely, the addition of high levels of surfactants (Tween 20, Tween 80, Brij 35, SDS, and DTAB) to the aqueous phase of oil-in-water emulsions has been reported to reduce the rate and extent of lipid digestion,107 which would be expected to decrease bioaccessibility (but this was not studied in this article). The surfactants may have inhibited lipid digestion through a number of mechanisms: (i) inhibition of lipase adsorption to the lipid droplet surfaces; (ii) binding to and denaturation of the lipase molecules; (iii) alteration in the aggregation state of the lipid droplets (Fig. 3 and 4).
Mineral ions.
Studies have shown that the addition of calcium ions to the aqueous phase of an emulsion may either increase or decrease the rate of lipid digestion depending on the system.94,108,109 The rate of lipid digestion may increase as the concentration of soluble calcium in the aqueous phase increases because the calcium ions precipitate long chain saturated fatty acids generated at the lipid droplet surfaces during digestion, thereby removing them and allowing the lipase to continue to function.109 A recent study showed that the addition of calcium ions to emulsions increased the lipid digestion rate, but lowered the bioaccessibility of β-carotene.99 It was proposed that the presence of the calcium ions caused the formation of insoluble complexes of bile salts and fatty acids that contained the carotenoids, thereby reducing their concentration in the mixed micelles. The addition of calcium ions to the aqueous phase could reduce lipid digestion and bioaccessibility if it promoted droplet aggregation or the formation of a gel-network around the lipid droplets, since these processes will decrease the access of lipase to the lipid droplet surfaces.
4.3. Effect of droplet size
The size of the droplets in emulsions may have a pronounced impact on lipid digestion and bioaccessibility because it alters the surface area of lipids exposed to the digestive components in the gastrointestinal fluids, such as bile salts, lipase, and protease.20 However, it is important to state that it is the size of the lipid droplets in the small intestine that is important, rather than their initial size.110 Emulsion droplets may become aggregated (coalesced or flocculated) within the mouth or stomach, which may alter the surface area of lipid exposed to lipase in the small intestine. In this situation, there may be little correlation between lipid digestion and bioaccessibility and the initial droplet size.
Studies with Tween 20-stabilized β-carotene-loaded emulsions have shown that the extent of lipid digestion and carotenoid bioaccessibility increased with decreasing droplet size (Fig. 8), which was attributed to more rapid and complete lipid digestion and mixed micelle formation occurred for smaller droplets because of their larger specific surface area.111 Studies with caseinate-stabilized oil-in-water emulsions fortified with β-carotene also showed that the rate of lipid digestion and carotenoid bioaccessibility increased with decreasing droplet size.112 A recent study confirmed that the rate of lipid digestion and carotenoid solubilization in mixed micelles increased as the lipid droplet diameter decreased.20 The relatively low bioaccessibility obtained for the large droplets was attributed to the fact that they were not fully digested, and so some of the carotenoids remained inside them and less mixed micelles were formed. A recent study with fucoxanthin-loaded oil droplets showed that the rate of lipid digestion and the carotenoid bioaccessibility increased with decreasing droplet size.113
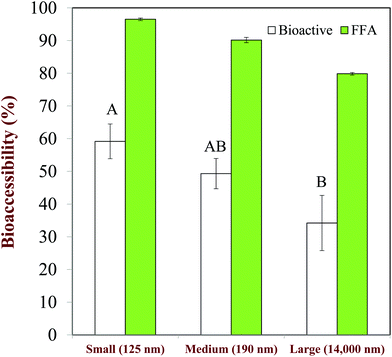 |
| Fig. 8 Impact of droplet size on the extent of lipid digestion (%FFA) and b-carotene bioaccessibility (%Bioactive) from oil-in-water emulsions. Mean droplet diameters shown in nm. The letters above the bioaccessibility data shown significant differences (p < 0.05). Adapted from Salvia-Trujillo et al. (2013).28,111 | |
Studies with excipient emulsions have shown that the bioaccessibility of carotenoids from tomatoes is increased as the lipid droplet size is decreased.114,115 The ability of emulsions to increase the bioaccessibility of lycopene from tomato extracts has also been shown to increase with decreasing droplet size,116 which may have been due to faster digestion and solubilization. Thus, reducing the droplet size in an emulsion-based delivery system may be advantageous for increasing the bioaccessibility of lipophilic bioactives. However, studies have shown that in some cases the absolute amount of a lipophilic bioactive (curcumin) in the mixed micelle phase produced after emulsion digestion may actually decrease with decreasing droplet size.117 This effect was attributed to the fact that curcumin is chemically unstable when it comes into contact with water, and therefore it degrades faster in smaller droplets because then a greater fraction of the curcumin was in close contact with the surrounding aqueous phase. Consequently, it may be important to optimize the particle size of the lipid droplets depending on the nature of the bioactive that is encapsulated.
In vivo studies have also shown that emulsions containing smaller droplets are typically digested more rapidly and give a higher bioaccessibility. In an early study, it was shown that fine emulsions (d = 0.7 μm) injected into the stomach were digested more rapidly than coarse emulsions (d = 10 μm), even though there were some changes in droplet size within the gastric phase.59 Recently published research, showed that the bioaccessibility of vitamin D encapsulated in emulsions increased with decreasing droplet size when determined using in vitro studies, but that its bioavailability actually decreased when determined using in vivo animal (rat) feeding studies.118 This poor in vivo – in vitro correlation was attributed to the fact that the simulated GIT used could not accurately reflect the complexity of the animal GIT, some droplet coalescence may have occurred in the rat GIT, and because the levels of the vitamin in the blood were not measured over time. Conversely, an in vivo rat feeding study using vitamin E encapsulated in emulsions showed that its bioavailability increased appreciably with decreasing droplet size.119 Emulsions containing very fine lipid droplets have also been shown to be effective at increasing the in vivo bioavailability of coenzyme Q10.120,121
4.4. Effect of droplet physical state
The lipid droplets in oil-in-water emulsions may be liquid, semi-solid, or solid depending on the nature of the lipid phase used and the thermal history of the sample.1 The physical state of the lipid phase can have a pronounced effect on lipid digestion and bioaccessibility. Studies have shown that oil-in-water emulsions stabilized by two kinds of non-ionic surfactants (Poloxamer 188 and Tween 20) were digested more rapidly and gave a higher carotenoid bioaccessibility when the droplets were liquid rather than solid.122 Other studies have shown that tripalmitin oil-in-water emulsions were digested more rapidly when the lipid phase was liquid rather than solid.40 Studies of lipid phases containing high melting point saturated fatty acids (such as stearic acid) and low melting point unsaturated fatty acids (oleic acid) showed that the steric acid was digested more slowly.123 These effects have been attributed to the fact that the lipase is able to access the ester bonds in the triacylglycerol molecules more readily when the lipid phase is liquid rather than when it is solid. The physical state of the lipid droplets may also alter lipid digestion and bioaccessibility of emulsions by altering the aggregation state of the droplets. Partially crystalline lipid droplets are susceptible to a phenomenon known as partial coalescence, where the crystals in one droplet penetrate into the liquid region in another droplet, leading to the formation of large clumps of aggregated droplets. This phenomenon has been shown to decrease the rate of lipid digestion because then it is more difficult for the lipase to reach the lipids in the interior of the clumps.41,124
There have been relatively few studies on the impact of the physical state of the lipid phase on the bioaccessibility of lipophilic bioactives, however, some studies have shown an effect. For instance, it was shown that the bioaccessibility of quercetin decreased in the following order nanostructure lipid carriers (NLC) > nanoemulsions (NE) > solid lipid nanoparticles (SLN).125 The NEs contained liquid droplets, the NLC contained partially crystalline lipid particles, and the SLN contained fully crystalline lipid particles. However, these effects may also have been due to differences in the size of the lipid particles in each system used in this study: SLN > NE > NLC. Another study showed that the rate of lipid digestion and β-carotene bioaccessibility was higher when it was encapsulated in emulsions containing liquid lipid droplets than in similarly-sized SLNs containing solid lipid particles.122
4.5. Effect of interfacial properties
The lipid droplets in oil-in-water emulsions may be coated by interfacial layers that vary considerable in their properties depending on the type of emulsifier and other surface-active substances present.1 For example, their composition, thickness, rheology, packing, hydrophobicity, and charge may change considerably, which can impact their gastrointestinal fate. Interfacial properties may be altered by using different kinds of emulsifiers to stabilize the lipid droplets, or by using interfacial engineering approaches, such as conjugation, layer-by-layer deposition, or particle adsorption.
Emulsifier type.
The impact of various types of food-grade non-ionic and anionic surfactants on lipid digestion and bioaccessibility have been studied using a dynamic in vitro GIT model.126 This study showed that there were appreciable differences in the rate and extent of lipid digestion depending on the nature of the surfactant used, which may be useful for controlling lipid digestion. Other studies have also examined the impact of emulsifier type on the fate of lipid droplets in the GIT. A comparison of lipid digestion in emulsions containing oil droplets coated by caseinate, lactoferrin, or Tween 80 reported that the caseinate-coated droplets had the slowest rate of lipid digestion.90 This effect was attributed to the fact that the caseinate-coated lipid droplets became highly aggregated in the stomach thereby inhibiting the ability of the lipase to access the droplets in the floc interior. A comparison of the lipid digestion rate of droplets coated by Pluronic F68 and lecithin suggested that surfactants that adsorb to the droplet surfaces more strongly (Pluronic) are more effective at inhibiting lipid digestion,42 presumably because they can inhibit the binding of bile salts and lipase (Fig. 4). Another study showed that β-carotene bioaccessibility was higher in emulsions containing lipid droplets coated by whey proteins, than in those coated by soybean soluble polysaccharides or by decaglycerol monolaurate.127 Lipid droplets coated with high methoxyl cellulose (MHC) were reported to be digested more slowly than those coated with protein.128 Differences in the gastrointestinal fate of lipid droplets coated by different kinds of emulsifiers can be attributed to various mechanisms (section 3.5), including: inhibition of bile salt or lipase adsorption; changing the initial droplet size; alteration in the droplet aggregation state; and, direct enzyme inhibition.
Surface–active conjugates.
The gastrointestinal fate of lipid droplet surfaces can also be controlled by coating the lipid droplets with surface–active conjugates. These conjugates are typically formed by covalently linking two or more molecules to form an amphiphilic molecule.129 A recent study showed that lipid droplets coated by polyphenol–protein–carbohydrate conjugates were more stable to aggregation under simulated GIT conditions than those coated by proteins alone, which led to a higher carotenoid bioaccessibility.130 In another study, it was shown that lipid droplets coated by protein–carbohydrate conjugates were more stable to droplet aggregation in the GIT than those stabilized by protein alone, but that the bioaccessibility was not impacted.131 A study using whey protein–beet pectin conjugates reported that droplets coated by the conjugates were more stable to aggregation than droplets coated by proteins alone, but that the rate of lipid digestion and β-carotene bioaccessibility were reduced by the conjugates.80 Surface-active protein–polyphenol conjugates have antioxidant properties may can also decrease the rate of degradation of encapsulated lipophilic components, such as polyunsaturated fatty acids or carotenoids, thereby increasing the amount available for absorption.129,132,133 It therefore appears that the nature of the conjugates used has a major impact on lipid digestion and bioaccessibility and should be optimized for a particular system.
Electrostatic deposition.
The interfacial properties of lipid droplets can also be altered after an emulsion has been formed using electrostatic deposition methods, where an electrically charged biopolymer is deposited onto the surface of an oppositely charged lipid droplet.134 This process can be repeated numerous times to form multiple layers of biopolymers around lipid droplets, which allows manipulation of the thickness, charge, and composition of the interfacial layer. Studies have shown that electrostatic deposition of anionic alginate molecules onto the surfaces of cationic lactoferrin-stabilized lipid droplets decreased the digestion rate, but not the final extent of digestion.85 Another recent study showed that curcumin-fortified emulsions containing alginate-lactoferrin coated lipid droplets behaved differently from lactoferrin-coated droplets in a dynamic simulated GIT.135 Nevertheless, the extent of lipid digestion and curcumin bioaccessibility were fairly similar for the two emulsions. Numerous other studies have shown that lipid droplets coated by multiple layers of biopolymers may be digested differently from those coated by a single layer, but did not examine nutraceutical bioaccessibility.85,92,136–141 For example, it has been shown that lipid droplets coated by pectin-gelatin coatings were more stable to aggregation under simulated GIT conditions than those coated only by gelatin, which led to a faster rate of lipid digestion.136
Particle adsorption (Pickering emulsions).
There has been great interest recently in modulating lipid digestion and bioaccessibility by adsorbing small colloidal particles, which may be inorganic or organic, onto the surfaces of the oil droplets in emulsions.142 These systems are usually called Pickering emulsions or colloidosomes.143–145 Coating lipid droplets with silica nanoparticles has been shown to reduce the rate of lipid digestion compared to coating them with β-lactoglobulin.146 A related study showed that lipid droplets coated with silica nanoparticles could protect curcumin from release under simulated gastric conditions, but then release it under simulated small intestine conditions.147 Much of the recent work in this area has focused on the utilization of natural colloidal particles to coat lipid droplets because of their potential for producing clean-label food products.142 Coating lipid droplets with certain types of digestible or indigestible polysaccharide particles has been shown to improve the chemical stability and bioaccessibility of curcumin under simulated gastrointestinal conditions, e.g., starch granules148 and chitosan-tripolyphosphate nanoparticles.149 Other studies have shown that coating lipid droplets with polysaccharide particles can inhibit lipid digestion, e.g., chitin nanocrystals150 or starch granules.151 Coating lipid droplets with different types of protein nanoparticles has also been shown to alter the bioaccessibility of lipophilic bioactive agents. For example, coating lipid droplets with soy glycinin nanoparticles was shown to slow down the release of β-carotene from the droplets under simulated GIT conditions.152 Coating lipid droplets with kafirin protein nanoparticles was shown to cause a slight decrease in the bioaccessibility of curcumin when compared to coating the lipid droplets with Tween 80.153 Other studies have shown that the adsorption of protein particles to the surfaces of lipid droplets can retard lipid digestion, e.g., whey protein microgels,154 kafirin protein nanoparticles,153 and pea protein nanoparticles.155 However, coating lipid droplets with lactoferrin nanoparticles was shown to have little impact on the rate of lipid digestion in the small intestine when compared to coating them with molecular lactoferrin.156 In summary, Pickering emulsions may be useful for controlling the digestion and bioavailability of lipids, but research is still needed to find economically viable sources of natural colloidal particles that can produce emulsions containing reasonably small lipid droplets.
4.6. Effect of droplet aggregation state
The droplets in emulsions may exist as freely dispersed individual entities or they may be aggregated in clumps i.e., flocculated or partially coalesced.1 The aggregation state of the lipid droplets in oil-in-water emulsions may change appreciably as they pass through the different regions of the GIT, which can have a pronounced effect on lipid digestion and bioaccessibility.2,41 Typically, droplet aggregation reduces lipid digestion, and therefore decreases bioaccessibility, because it is more difficult for the lipase molecules to reach the surfaces of the droplets within the interior of the flocs (Fig. 3). Consequently, emulsifier-coated lipid droplets that have a strong tendency to aggregate under GIT conditions may lead to slower digestion and a reduced bioaccessibility.4,41
Caseinate-coated lipid droplets have been shown to be highly aggregated under simulated gastric and small intestine conditions, which reduces lipid digestion and bioaccessibility when compared to lipid droplets coated by emulsifiers not prone to this effect, such as Tween 80.89 The bioaccessibility of β-carotene loaded in whey protein-coated lipid droplets was reported to be less than that in Tween 20-coated droplets for a similar reason.97 A higher β-carotene bioaccessibility was reported in emulsions containing lipid droplets coated by polyphenol–protein–carbohydrate conjugates than in those coated by proteins alone, which was attributed to the fact that the adsorbed conjugates inhibited extensive droplet flocculation under GIT conditions.130 Similarly, a higher lutein bioaccessibility was reported in emulsions containing lipid droplets coated by casein–dextrin conjugates than in those containing droplets coated by casein alone, which was again attributed to the ability of the conjugates to inhibit flocculation.131 Moreover, a higher β-carotene bioaccessibility was reported in emulsions containing lipid droplets coated by a whey protein–pectin conjugate than by whey protein alone for a similar reason.80 Including additives in emulsions that promote droplet flocculation has also been shown to inhibit lipid digestion and bioaccessibility, e.g., adding cationic chitosan to emulsions containing protein-coated droplets.81 Conversely, including additives that inhibit lipid droplet flocculation has been shown to promote lipid digestion and improve bioaccessibility, e.g., adding anionic fucoidan to emulsions containing caseinate-coated lipid droplets.89 Encapsulation of β-carotene-loaded lipid droplets in starch hydrogels was also reported to improve carotenoid bioaccessibility, which was again attributed to the ability of the hydrogels to inhibit droplet flocculation in the GIT.97 Overall, these results indicate that the bioaccessibility of bioactive agents can be controlled by altering the flocculation state of the lipid droplets in emulsions, which can be achieved by altering emulsifier type, interfacial properties, or incorporating additives.
4.7. Effect of droplet encapsulation in matrices
The lipid droplets in emulsion-based delivery systems may be encapsulated within microscopic or macroscopic hydrogels so as to alter their physicochemical properties and functional attributes.54 Encapsulation of β-carotene-loaded oil droplets inside alginate microgels has been shown to increase their chemical stability, but decrease their bioaccessibility under simulated GIT conditions.157 This latter effect is attributed to the fact that the digestive enzymes (lipase) have to penetrate through the hydrogel matrix before they can reach surfaces of the lipid droplets, and so triacylglycerol digestion, mixed micelle formation, and carotenoid release all occur more slowly (section 3.7). Similar results have been reported for another hydrophobic bioactive agent (nobilitin) encapsulated in alginate microgels.158 The encapsulation of curcumin in biopolymer microgels was also reported to decrease its bioaccessibility by an amount depending on polysaccharide type: free lipid droplets (73%) > carrageenan microgels (33%) > alginate microgels (16%).159 It was proposed that alginate microgels were more effective at decreasing curcumin bioaccessibility than carrageenan microgels because they had smaller pore sizes and were more resistant to disintegration under GIT conditions. Microgels may be useful for improving the chemical stability of lipophilic nutraceuticals in food products, but they should be carefully designed to ensure that the bioactive agents are actually released and absorbed after ingestion.
The encapsulation of β-carotene-loaded oil droplets within starch hydrogels has been shown to enhance carotenoid bioaccessibility using a simulated GIT.96,97 This effect was attributed to the ability of the starch hydrogels to inhibit extensive flocculation of the oil droplets in the gastric phase, which would normally reduce lipid digestion by making it more difficult for the lipase to access the lipid droplets in the floc interiors (section 4.7).
4.8. Effect of other additives
Various other additives can be added to emulsions to increase the amount of the bioactive agent that is available for absorption. For example, antioxidants can be added to the oil, water, or interfacial phases to retard the chemical degradation of bioactive components, and therefore increase the amount present in an active form.160–162 Similarly, chelating agents (such as EDTA) can be added to the aqueous phase of emulsions to chelate transition metals, and therefore improve the stability of encapsulated lipophilic agents to chemical degradation.163 Consequently, there is considerable scope for increasing the total amount of a bioactive component that is solubilized within the mixed micelle phase in an active form by selecting appropriate additives.
5. Conclusions
There has been a large increase in the number of studies investigating the impact of emulsion composition and structure on the bioaccessibility of lipophilic bioactive agents over the past decade or so. Researchers have examined the impact of lipid phase type, concentration, and physical state: droplet dimensions and surface area; emulsifier type and interfacial properties; physical state; and additives such as thickeners, gelling agents, mineral ions, antioxidants, and chelating agents. These studies have provided valuable insights into the major factors impacting the bioaccessibility of lipophilic bioactives. The knowledge gained from these studies can therefore be used to design emulsion-based delivery systems for increasing the bioaccessibility of specific kinds of bioactive agents. For example, experiments with carotenoids suggest that their bioaccessibility can be enhanced by encapsulating them in oil-in-water emulsions with the following properties: (i) a sufficiently high quantity of lipid phase (>4%); (ii) a lipid phase comprised of digestible long chain triacylglycerols (>16 carbons); (iii) a lipid phase that is liquid (rather than crystalline) at body temperature; (iv) lipid droplets that are relatively small (<200 nm); and (v) lipid droplets that are coated with an emulsifier that inhibits droplet aggregation in the GIT, but enables easy absorption of bile salts and lipids to the droplet surfaces. Moreover, the addition of antioxidants to the lipid phase may retard degradation of carotenoids during storage and in the GIT, thereby increasing the amount available for absorption. Emulsion-based delivery systems for other types of lipophilic bioactive agents may need to be tailored for each specific application depending on the properties of the bioactive and food matrix. Research is continuing to screen different kinds of emulsion components and designs on bioaccessibility, and examining the functionality of next-generation emulsion-based systems, such as those created using interfacial engineering, embedding, and combination approaches. Future studies should focus on establishing the physicochemical mechanisms of the observed effects, and in carrying out animal and human feeding studies to demonstrate the efficacy of the delivery systems in practice.
Conflicts of interest
There are no conflicts to declare.
Acknowledgements
This material was partly based upon work supported by the National Institute of Food and Agriculture, USDA, Massachusetts Agricultural Experiment Station (MAS00491) and USDA, AFRI Grants (2014-67021, 2016-25147, 2016-08782).
References
-
D. J. McClements, Food Emulsions: Principles, Practices, and Techniques, CRC Press, Boca Raton, FL, 3rd edn, 2015 Search PubMed.
- D. J. McClements and H. Xiao, Food Funct., 2012, 3, 202–220 CAS.
- A. Steingoetter, S. Buetikofer, J. Curcic, D. Menne, J. F. Rehfeld, M. Fried, W. Schwizer and T. J. Wooster, J. Nutr., 2017, 147, 706–714 CrossRef CAS PubMed.
- T. J. Wooster, S. C. Moore, W. Chen, H. Andrews, R. Addepalli, R. B. Seymour and S. A. Osborne, RSC Adv., 2017, 7, 40053–40066 RSC.
- B. Ozturk, Eur. J. Lipid Sci. Technol., 2017, 119, 1–18 CrossRef.
- P. Joyce, C. P. Whitby and C. A. Prestidge, Adv. Colloid Interface Sci., 2016, 237, 52–75 CrossRef CAS PubMed.
- F. Jimenez-Colmenero, Food Res. Int., 2013, 52, 64–74 CrossRef CAS.
- L. Salvia-Trujillo, O. Martin-Belloso and D. J. McClements, Nanomaterials, 2016, 6, 1–16 CrossRef PubMed.
- J. K. Aronson, Br. J. Clin. Pharmacol., 2017, 83, 8–19 CrossRef PubMed.
- M. N. Corstens, C. C. Berton-Carabin, R. de Vries, F. J. Troost, A. A. M. Masclee and K. Schroen, Crit. Rev. Food Sci. Nutr., 2017, 57, 2218–2244 CrossRef CAS PubMed.
- A. M. Lett, J. E. Norton and M. R. Yeomans, Appetite, 2016, 96, 18–24 CrossRef PubMed.
- T. J. Wooster, L. Day, M. Xu, M. Golding, S. Oiseth, J. Keogh and P. Clifton, Food Hydrocolloids, 2014, 36, 102–114 CrossRef CAS.
-
S. Marze, in Annual Review of Food Science and Technology, ed. M. P. Doyle and T. R. Klaenhammer, 2017, vol. 8, pp. 35–55 Search PubMed.
- D. J. McClements, F. Li and H. Xiao, Annu. Rev. Food Sci. Technol., 2015, 6, 299–327 CrossRef CAS PubMed.
- A. M. B. Priyadarshani, Crit. Rev. Food Sci. Nutr., 2017, 57, 1710–1717 CrossRef CAS PubMed.
- R. Zhang, W. Wu, Z. Zhang, Y. Park, L. He, B. Xing and D. J. McClements, J. Agric. Food Chem., 2017, 65, 9128–9138 CrossRef CAS PubMed.
- E. Fernandez-Garcia, I. Carvajal-Lerida and A. Perez-Galvez, Nutr. Res., 2009, 29, 751–760 CrossRef CAS PubMed.
- V. Raikos and V. Ranawana, Int. J. Food Sci. Technol., 2017, 52, 68–80 CrossRef CAS.
- D. J. McClements, L. Saliva-Trujillo, R. J. Zhang, Z. P. Zhang, L. Q. Zou, M. F. Yao and H. Xiao, Food Res. Int., 2016, 88, 140–152 CrossRef CAS PubMed.
- L. Salvia-Trujillo, S. H. E. Verkempinck, L. Sun, A. M. Van Loey, T. Grauwet and M. E. Hendrickx, Food Chem., 2017, 229, 653–662 CrossRef CAS PubMed.
- S. R. Goltz, W. W. Campbell, C. Chitchumroonchokchai, M. L. Failla and M. G. Ferruzzi, Mol. Nutr. Food Res., 2012, 56, 866–877 CAS.
- T. Huo, M. G. Ferruzzi, S. J. Schwartz and M. L. Failla, J. Agric. Food Chem., 2007, 55, 8950–8957 CrossRef CAS PubMed.
- C. Qian, E. A. Decker, H. Xiao and D. J. McClements, Food Chem., 2012, 135, 1440–1447 CrossRef CAS PubMed.
- M. Sessa, M. L. Balestrieri, G. Ferrari, L. Servillo, D. Castaldo, N. D'Onofrio, F. Donsi and R. Tsao, Food Chem., 2014, 147, 42–50 CrossRef CAS PubMed.
- H. T. Cho, L. Salvia-Trujillo, J. Kim, Y. Park, H. Xiao and D. McClements, Food Chem., 2014, 156, 117–122 CrossRef CAS PubMed.
- R. J. Zhang, Z. P. Zhang, L. Q. Zou, H. Xiao, G. D. Zhang, E. A. Decker and D. J. McClements, Food Biophys., 2016, 11, 71–80 CrossRef.
- H. D. Williams, N. L. Trevaskis, Y. Y. Yeap, M. U. Anby, C. W. Pouton and C. J. H. Porter, Pharm. Res., 2013, 30, 2976–2992 CrossRef CAS PubMed.
- L. Salvia-Trujillo, C. Qian, O. Martin-Belloso and D. J. McClements, Food Chem., 2013, 139, 878–884 CrossRef CAS PubMed.
- M. Lopez, S. E. Evangelista, M. Morales and S. Lee, Langmuir, 2017, 33, 900–912 CrossRef CAS PubMed.
- I. Vodyanoy and J. E. Hall, Biophys. J., 1984, 46, 187–194 CrossRef CAS PubMed.
- N. Kucerka, F. A. Heberle, J. J. Pan and J. Katsaras, Membranes, 2015, 5, 454–472 CrossRef CAS PubMed.
-
M. Helliwell, in Carotenoids, Volume 4: Natural Functions, ed. G. Britton, Birkhäuser Verlag, Basel, 2008, ch. 4, pp. 37–52 Search PubMed.
- N. Kucerka, J. F. Nagle, J. N. Sachs, S. E. Feller, J. Pencer, A. Jackson and J. Katsaras, Biophys. J., 2008, 95, 2356–2367 CrossRef CAS PubMed.
- Y. Li, M. Hu and D. J. McClements, Food Chem., 2011, 126, 498–505 CrossRef CAS.
- Y. Li and D. J. McClements, J. Agric. Food Chem., 2010, 58, 8085–8092 CrossRef CAS PubMed.
- T. M. Giang, S. Gaucel, P. Brestaz, M. Anton, A. Meynier, I. C. Trelea and S. Le Feunteun, Food Chem., 2016, 194, 1180–1188 CrossRef CAS PubMed.
- S. Gaucel, I. C. Trelea and S. Le Feunteun, J. Agric. Food Chem., 2015, 63, 10352–10353 CrossRef CAS PubMed.
- H. D. Williams, P. Sassene, K. Kleberg, J. C. Bakala-N'Goma, M. Calderone, V. Jannin, A. Igonin, A. Partheil, D. Marchaud, E. Jule, J. Vertommen, M. Maio, R. Blundell, H. Benameur, F. Carriere, A. Mullertz, C. J. H. Porter and C. W. Pouton, J. Pharm. Sci., 2012, 101, 3360–3380 CrossRef CAS PubMed.
- H. L. Yu and Q. R. Huang, J. Agric. Food Chem., 2012, 60, 5373–5379 CrossRef CAS PubMed.
- L. Bonnaire, S. Sandra, T. Helgason, E. A. Decker, J. Weiss and D. J. McClements, J. Agric. Food Chem., 2008, 56, 3791–3797 CrossRef CAS PubMed.
- M. Golding, T. J. Wooster, L. Day, M. Xu, L. Lundin, J. Keogh and P. Clifton, Soft Matter, 2011, 7, 3513–3523 RSC.
- A. Torcello-Gomez, J. Maldonado-Valderrama, A. Martin-Rodriguez and D. J. McClements, Soft Matter, 2011, 7, 6167–6177 RSC.
- B. Hu, L. Y. Zhang, R. Liang, F. Z. Chen, L. P. He, B. Hu and X. M. Zeng, J. Agric. Food Chem., 2015, 63, 2033–2040 CrossRef CAS PubMed.
- L. Razumovsky and S. Damodaran, J. Agric. Food Chem., 2001, 49, 3080–3086 CrossRef CAS PubMed.
- V. B. Fainerman, E. Lucassen-Reynders and R. Miller, Colloids Surf., A, 1998, 143, 141–165 CrossRef CAS.
- C. Kotsmar, V. Pradines, V. S. Alahverdjieva, E. V. Aksenenko, V. B. Fainerman, V. I. Kovalchuk, J. Kragel, M. E. Leser, B. A. Noskov and R. Miller, Adv. Colloid Interface Sci., 2009, 150, 41–54 CrossRef CAS PubMed.
- E. Guzman, S. Llamas, A. Maestro, L. Fernandez-Pena, A. Akanno, R. Miller, F. Ortega and R. G. Rubio, Adv. Colloid Interface Sci., 2016, 233, 38–64 CrossRef CAS PubMed.
- V. B. Fainerman and E. H. Lucassen-Reynders, Adv. Colloid Interface Sci., 2002, 96, 295–323 CrossRef CAS PubMed.
- D. K. Qin, X. J. Yang, S. R. Gao, J. H. Yao and D. J. McClements, J. Food Sci., 2016, 81, C1636–C1645 CrossRef CAS PubMed.
- O. Torres, B. Murray and A. Sarkar, Trends Food Sci. Technol., 2016, 55, 98–108 CrossRef CAS.
- Z. P. Zhang, R. J. Zhang, L. Chen, Q. Y. Tong and D. J. McClements, Eur. Polym. J., 2015, 72, 698–716 CrossRef CAS.
-
E. Dickinson, in Annual Review of Food Science and Technology, ed. M. P. Doyle and T. R. Klaenhammer, 2015, vol. 6, pp. 211–233 Search PubMed.
- Y. Li, M. Hu, Y. Du, H. Xiao and D. J. McClements, Food Hydrocolloids, 2011, 25, 122–130 CrossRef CAS.
- D. J. McClements, Adv. Colloid Interface Sci., 2017, 240, 31–59 CrossRef CAS PubMed.
-
J. Crank, The Mathematics of Diffusion, Oxford Science Publications, Oxford, U.K., 2nd edn, 1975 Search PubMed.
- A. W. Chan and R. J. Neufeld, Biomaterials, 2009, 30, 6119–6129 CrossRef CAS PubMed.
- Y. Zhang and B. G. Amsden, Macromolecules, 2006, 39, 1073–1078 CrossRef CAS.
- B. Zeeb, A. H. Saberi, J. Weiss and D. J. McClements, Food Hydrocolloids, 2015, 50, 27–36 CrossRef CAS.
- P. Borel, P. Grolier, M. Armand, A. Partier, H. Lafont, D. Lairon and V. AzaisBraesco, J. Lipid Res., 1996, 37, 250–261 CAS.
- P. C. Mashurabad, R. Palika, Y. W. Jyrwa, K. Bhaskarachary and R. Pullakhandam, J. Food Sci. Technol., 2017, 54, 333–341 CrossRef CAS PubMed.
- Q. Li, T. Li, C. M. Liu, J. Chen, R. J. Zhang, Z. P. Zhang, T. T. Dai and D. J. McClements, Food Res. Int., 2016, 89, 320–329 CrossRef CAS PubMed.
- X. Liu, J. F. Bi, H. Xiao and D. J. McClements, J. Food Sci., 2016, 81, N754–N761 CrossRef CAS PubMed.
- X. Liu, J. F. Bi, H. Xiao and D. J. McClements, J. Agric. Food Chem., 2015, 63, 8534–8543 CrossRef CAS PubMed.
- D. J. McClements, L. Q. Zou, R. J. Zhang, L. Salvia-Trujillo, T. Kumosani and H. Xiao, Compr. Rev. Food Sci. Food Saf., 2015, 14, 824–847 CrossRef CAS.
- L. Salvia-Trujillo, Q. Sun, B. H. Urn, Y. Park and D. J. McClements, J. Funct. Foods, 2015, 17, 293–304 CrossRef CAS.
- R. J. Zhang, Z. P. Zhang, T. Kumosani, S. Khoja, K. O. Abualnaja and D. J. McClements, Food Biophys., 2016, 11, 154–164 CrossRef.
- Y. Yang, H. Xiao and D. J. McClements, J. Agric. Food Chem., 2017, 65, 3946–3955 CrossRef CAS PubMed.
- L. Mutsokoti, A. Panozzo, A. P. Pallares, S. Jaiswal, A. Van Loey, T. Grauwet and M. Hendrickx, Food Chem., 2017, 232, 124–134 CrossRef CAS PubMed.
- C. M. O'Sullivan, M. Davidovich-Pinhas, A. J. Wright, S. Barbut and A. G. Marangoni, Food Funct., 2017, 8, 1438–1451 Search PubMed.
- S. Marze, C. Gaillard and P. Roblin, Soft Matter, 2015, 11, 5365–5373 RSC.
- S. Salentinig, H. Amenitsch and A. Yaghmur, ACS Omega, 2017, 2, 1441–1446 CrossRef CAS.
- O. Rezhdo, S. Di Maio, P. Le, K. C. Littrell, R. L. Carrier and S. H. Chen, J. Colloid Interface Sci., 2017, 499, 189–201 CrossRef CAS PubMed.
- S. Phan, S. Salentinig, E. Gilbert, T. A. Darwish, A. Hawley, R. Nixon-Luke, G. Bryant and B. J. Boyd, J. Colloid Interface Sci., 2015, 449, 160–166 CrossRef CAS PubMed.
- A. Gasa-Falcon, I. Odriozola-Serrano, G. Oms-Oliu and O. Martin-Belloso, LWT–Food Sci. Technol., 2017, 84, 331–337 CrossRef CAS.
- S. Mun, S. Park, Y. R. Kim and D. J. McClements, Food Res. Int., 2016, 87, 18–24 CrossRef CAS.
- T. A. J. Verrijssen, L. G. Balduyck, S. Christiaens, A. M. Van Loey, S. Van Buggenhout and M. E. Hendrickx, Food Res. Int., 2014, 57, 71–78 CrossRef CAS.
- T. A. J. Verrijssen, S. Christiaens, S. H. E. Verkempinck, J. Boeve, T. Grauwet, A. M. Van Loey, L. Salvia-Trujillo and M. E. Hendrickx, J. Food Sci., 2016, 81, C2327–C2336 CrossRef CAS PubMed.
- T. A. J. Verrijssen, S. H. E. Verkempinck, S. Christiaens, A. M. Van Loey and M. E. Hendrickx, Food Hydrocolloids, 2015, 49, 73–81 CrossRef CAS.
- B. Cervantes-Paz, J. D. Ornelas-Paz, J. D. Perez-Martinez, J. Reyes-Hernandez, P. B. Zamudio-Flores, C. Rios-Velasco, V. Ibarra-Junquera and S. Ruiz-Cruz, Food Hydrocolloids, 2016, 60, 580–588 CrossRef CAS.
- D. X. Xu, F. Yuan, Y. X. Gao, A. Panya, D. J. McClements and E. A. Decker, Food Chem., 2014, 156, 374–379 CrossRef CAS PubMed.
- C. L. Zhang, W. Xu, W. P. Jin, B. R. Shah, Y. Li and B. Li, Food Res. Int., 2015, 77, 419–425 CrossRef CAS.
- C. Soukoulis, M. Tsevdou, L. Yonekura, S. Cambier, P. S. Taoukis and L. Hoffmann, Carbohydr. Polym., 2017, 167, 259–269 CrossRef CAS PubMed.
- C. Soukoulis, M. Tsevdou, C. M. Andre, S. Cambier, L. Yonekura, P. S. Taoukis and L. Hoffmann, Food Chem., 2017, 220, 208–218 CrossRef CAS PubMed.
- Z. Wang, M. A. Neves, I. Kobayashi, K. Uemura and M. Nakajima, Biosci., Biotechnol., Biochem., 2013, 77, 467–474 CrossRef CAS PubMed.
- T. Tokle, U. Lesmes, E. A. Decker and D. J. McClements, Food Funct., 2012, 3, 58–66 CAS.
- D. K. Qin, X. J. Yang, S. R. Gao, J. H. Yao and D. J. McClements, Food Hydrocolloids, 2017, 69, 382–392 CrossRef CAS.
- M. Espinal-Ruiz, F. Parada-Alfonso, L. P. Restrepo-Sanchez, C. E. Narvaez-Cuenca and D. J. McClements, Food Funct., 2014, 5, 3083–3095 CAS.
- M. Espinal-Ruiz, L. P. Restrepo-Sanchez, C. E. Narvaez-Cuenca and D. J. McClements, Food Hydrocolloids, 2016, 52, 329–342 CrossRef CAS.
- Y. G. Chang and D. J. McClements, Food Hydrocolloids, 2016, 61, 92–101 CrossRef CAS.
- R. J. Zhang, Z. P. Zhang, H. Zhang, E. A. Decker and D. J. McClements, Food Hydrocolloids, 2015, 45, 175–185 CrossRef CAS.
- M. Beysseriat, E. A. Decker and D. J. McClements, Food Hydrocolloids, 2006, 20, 800–809 CrossRef CAS.
- B. Zeeb, J. Weiss and D. J. McClements, Food Chem., 2015, 180, 257–264 CrossRef CAS PubMed.
- M. Espina-Ruiz, F. Parada-Alfonso, L. P. Restrepo-Sanchez, C. E. Narvaez-Cuenca and D. J. McClements, J. Agric. Food Chem., 2014, 62, 12620–12630 CrossRef PubMed.
- M. Hu, Y. Li, E. A. Decker and D. J. McClements, Food Hydrocolloids, 2010, 24, 719–725 CrossRef CAS.
- N. Tangsrianugul, M. Suphantharika and D. J. McClements, Food Res. Int., 2015, 78, 79–87 CrossRef CAS PubMed.
- S. Mun, Y. R. Kim and D. J. McClements, Food Chem., 2015, 173, 454–461 CrossRef CAS PubMed.
- S. Mun, Y. R. Kim, M. Shin and D. J. McClements, Food Hydrocolloids, 2015, 44, 380–389 CrossRef CAS.
- T. Sanz and H. Luyten, Food Hydrocolloids, 2007, 21, 203–211 CrossRef CAS.
- Q. Q. Lin, R. Liang, A. Q. Ye, H. Singh and F. Zhong, Food Hydrocolloids, 2017, 73, 184–193 CrossRef CAS.
- C. S. Levi, N. Goldstein, R. Portmann and U. Lesmes, Food Hydrocolloids, 2017, 69, 393–401 CrossRef.
- A. I. Mulet-Cabero, N. M. Rigby, A. Brodkorb and A. R. Mackie, Food Hydrocolloids, 2017, 67, 63–73 CrossRef CAS.
- S. Lamothe, N. Remillard, J. Tremblay and M. Britten, Food Res. Int., 2017, 92, 138–146 CrossRef CAS PubMed.
- C. Y. Qiu, M. M. Zhao, E. A. Decker and D. J. McClements, Food Chem., 2015, 175, 249–257 CrossRef CAS PubMed.
- Y. W. Liu, F. Lei, F. Yuan and Y. X. Gao, Food Funct., 2014, 5, 2940–2947 CAS.
- L. Q. Zou, W. Liu, C. M. Liu, H. Xiao and D. J. McClements, Food Funct., 2015, 6, 2475–2486 CAS.
- T. A. J. Verrijssen, K. H. G. Smeets, S. Christiaens, S. Palmers, A. M. Van Loey and M. E. Hendrickx, Food Res. Int., 2015, 67, 60–66 CrossRef CAS.
- Y. Li and D. J. McClements, Eur. J. Pharm. Biopharm., 2011, 79, 423–431 CrossRef CAS PubMed.
- A. Q. Ye, J. Cui, X. Q. Zhu and H. Singh, Food Chem., 2013, 139, 681–688 CrossRef CAS PubMed.
- R. Devraj, H. D. Williams, D. B. Warren, A. Mullertz, C. J. H. Porter and C. W. Pouton, Int. J. Pharm., 2013, 441, 323–333 CrossRef CAS PubMed.
- P. Borel, M. Armand, B. Pasquier, M. Senft, G. Dutot, C. Melin, H. Lafont and D. Lairon, JPEN, J. Parenter. Enteral Nutr., 1994, 18, 534–543 CrossRef CAS PubMed.
- L. Salvia-Trujillo, C. Qian, O. Martin-Belloso and D. J. McClements, Food Chem., 2013, 141, 1472–1480 CrossRef CAS PubMed.
- J. Yi, Y. Li, F. Zhong and W. Yokoyama, Food Hydrocolloids, 2014, 35, 19–27 CrossRef CAS.
- Z. H. Huang, L. Q. Xu, X. M. Zhu, J. N. Hu, H. L. Peng, Z. L. Zeng and H. Xiong, Int. J. Food Eng., 2017, 13, 1–12 CAS.
- Q. Li, T. Li, C. M. Liu, T. T. Dai, R. J. Zhang, Z. P. Zhang and D. J. McClemnets, Food Biophys., 2017, 12, 172–185 CrossRef.
- L. Salvia-Trujillo and D. J. McClements, Food Chem., 2016, 210, 295–304 CrossRef CAS PubMed.
- T. V. A. Ha, S. Kim, Y. Choi, H. S. Kwak, S. J. Lee, J. Y. Wen, I. Oey and S. Ko, Food Chem., 2015, 178, 115–121 CrossRef CAS PubMed.
- L. Q. Zou, B. J. Zheng, W. Liu, C. M. Liu, H. Xiao and D. J. McClements, J. Funct. Foods, 2015, 15, 72–83 CrossRef CAS.
- L. Salvia-Trujillo, B. Fumiaki, Y. Park and D. J. McClements, Food Funct., 2017, 8, 767–777 CAS.
- S. Parthasarathi, S. P. Muthukumar and C. Anandharamakrishnan, Food Funct., 2016, 7, 2294–2302 CAS.
- J. Hatanaka, Y. Kimura, Z. Lai-Fu, S. Onoue and S. Yamada, Int. J. Pharm., 2008, 363, 112–117 CrossRef CAS PubMed.
- H. M. Ok, S. M. Kim, J. W. Park, K. S. Kim, H. K. Nam, J. Y. Kim and O. Kwon, J. Korean Soc. Appl. Biol. Chem., 2012, 55, 619–623 CrossRef CAS.
- A. M. Nik, S. Langmaid and A. J. Wright, Food Funct., 2012, 3, 234–245 CAS.
- S. H. Thilakarathna, M. Rogers, Y. Lan, S. Huynh, A. G. Marangoni, L. E. Robinson and A. J. Wright, Food Funct., 2016, 7, 1932–1940 CAS.
- A. Steingoetter, T. Radovic, S. Buetikofer, J. Curcic, D. Menne, M. Fried, W. Schwizer and T. J. Wooster, Am. J. Clin. Nutr., 2015, 101, 714–724 CrossRef CAS PubMed.
- N. P. Aditya, A. S. Macedo, S. Doktorovov, E. B. Souto, S. Kim, P. S. Chang and S. Ko, LWT–Food Sci. Technol., 2014, 59, 115–121 CrossRef CAS.
- A. Speranza, M.
G. Corradini, T. G. Hartman, D. Ribnicky, A. Oren and M. A. Rogers, J. Agric. Food Chem., 2013, 61, 6505–6515 CrossRef CAS PubMed.
- Y. W. Liu, Z. Q. Hou, F. Lei, Y. Y. Chang and Y. X. Gao, Innovative Food Sci. Emerging Technol., 2012, 15, 86–95 CrossRef.
- J. Borreani, M. Espert, A. Salvador, T. Sanz, A. Quiles and I. Hernando, Food Funct., 2017, 8, 1547–1557 CAS.
- F. G. Liu, C. C. Ma, D. J. McClements and Y. X. Gao, Food Hydrocolloids, 2016, 61, 578–588 CrossRef CAS.
- F. G. Liu, C. C. Ma, R. J. Zhang, Y. X. Gao and D. J. McClements, Food Chem., 2017, 221, 395–403 CrossRef CAS PubMed.
- C. E. Gumus, G. Davidov-Pardo and D. J. McClements, Food Hydrocolloids, 2016, 60, 38–49 CrossRef CAS.
- L. P. Gu, N. Peng, C. H. Chang, D. J. McClements, Y. J. Su and Y. J. Yang, Food Biophys., 2017, 12, 198–210 CrossRef.
- L. P. Gu, Y. J. Su, M. Q. Zhang, C. H. Chang, J. H. Li, D. J. McClements and Y. J. Yang, Food Res. Int., 2017, 96, 84–93 CrossRef CAS PubMed.
- D. J. McClements, J. Food Sci., 2010, 75, R30–R42 CrossRef CAS PubMed.
- A. C. Pinheiro, M. A. Coimbra and A. A. Vicente, Food Hydrocolloids, 2016, 52, 460–467 CrossRef CAS.
- B. Zeeb, C. L. Lopez-Pena, J. Weiss and D. J. McClements, Food Hydrocolloids, 2015, 46, 125–133 CrossRef CAS.
- V. Gudipati, S. Sandra, D. J. McClements and E. A. Decker, J. Agric. Food Chem., 2010, 58, 8093–8099 CrossRef CAS PubMed.
- M. Hu, Y. Li, E. A. Decker, H. Xiao and D. J. McClements, Food Biophys., 2011, 6, 37–48 CrossRef.
- U. Klinkesorn and D. J. McClements, Food Biophys., 2010, 5, 73–81 CrossRef.
- Y. Li, M. Hu, H. Xiao, Y. M. Du, E. A. Decker and D. J. McClements, Eur. J. Pharm. Biopharm., 2010, 76, 38–47 CrossRef CAS PubMed.
- Y. Li and D. J. McClements, Food Hydrocolloids, 2014, 35, 367–374 CrossRef.
- J. Xiao, Y. Q. Li and Q. R. Huang, Trends Food Sci. Technol., 2016, 55, 48–60 CrossRef CAS.
-
C. C. Berton-Carabin and K. Schroen, in Annual Review of Food Science and Technology, ed. M. P. Doyle and T. R. Klaenhammer, 2015, vol. 6, pp. 263–297 Search PubMed.
- E. Dickinson, Trends Food Sci. Technol., 2012, 24, 4–12 CrossRef CAS.
- I. Tavernier, W. Wijaya, P. Van der Meeren, K. Dewettinck and A. R. Patel, Trends Food Sci. Technol., 2016, 50, 159–174 CrossRef CAS.
- P. E. Ruiz-Rodriguez, D. Meshulam and U. Lesmes, Food Biophys., 2014, 9, 406–415 CrossRef.
- R. V. Tikekar, Y. J. Pan and N. Nitin, Food Res. Int., 2013, 51, 370–377 CrossRef CAS.
- A. Marefati, M. Bertrand, M. Sjoo, P. Dejmek and M. Rayner, Food Hydrocolloids, 2017, 63, 309–320 CrossRef CAS.
- B. R. Shah, C. L. Zhang, Y. Li and B. Li, Food Res. Int., 2016, 89, 399–407 CrossRef CAS PubMed.
- M. V. Tzoumaki, T. Moschakis, E. Scholten and C. G. Biliaderis, Food Funct., 2013, 4, 121–129 CAS.
- M. Sjoo, S. C. Emek, T. Hall, M. Rayner and M. Wahlgren, J. Colloid Interface Sci., 2015, 450, 182–188 CrossRef PubMed.
- F. Liu and C. H. Tang, Food Hydrocolloids, 2016, 60, 631–640 CrossRef CAS.
- J. Xiao, C. Lo and Q. R. Huang, J. Agric. Food Chem., 2015, 63, 10263–10270 CrossRef CAS PubMed.
- A. Sarkar, B. Murray, M. Holmes, R. Ettelaie, A. Abdalla and X. Yang, Soft Matter, 2016, 12, 3558–3569 RSC.
- Y. Shao and C. H. Tang, Food Res. Int., 2016, 79, 64–72 CrossRef CAS.
- D. Meshulam and U. Lesmes, Food Funct., 2014, 5, 65–73 CAS.
- Z. P. Zhang, R. J. Zhang and D. J. McClements, Food Hydrocolloids, 2016, 61, 1–10 CrossRef CAS.
- L. L. Lei, Y. Z. Zhang, L. L. He, S. Wu, B. Li and Y. Li, LWT–Food Sci. Technol., 2017, 82, 260–267 CrossRef CAS.
- Z. P. Zhang, R. J. Zhang, L. Q. Zou, L. Chen, Y. Ahmed, W. Al Bishri, K. Balamash and D. J. McClements, Food Hydrocolloids, 2016, 58, 160–170 CrossRef CAS.
- D. Sotomayor-Gerding, B. D. Oomah, F. Acevedo, E. Morales, M. Bustamante, C. Shene and M. Rubilar, Food Chem., 2016, 199, 463–470 CrossRef CAS PubMed.
- C. Qian, E. A. Decker, H. Xiao and D. J. McClements, Food Chem., 2012, 135, 1036–1043 CrossRef CAS PubMed.
- H. R. Sharif, H. D. Goff, H. Majeed, F. Liu, J. Nsor-Atindana, J. Haider, R. Liang and F. Zhong, Colloids Surf., A, 2017, 529, 550–559 CrossRef CAS.
- P. Shao, Q. Qiu, J. Xiao, Y. G. Zhu and P. L. Sun, Int. J. Biol. Macromol., 2017, 102, 225–231 CrossRef CAS PubMed.
|
This journal is © The Royal Society of Chemistry 2018 |