DOI:
10.1039/C7AN01101F
(Paper)
Analyst, 2017,
142, 3674-3679
Titanium nanoparticles (TiO2)/graphene oxide nanosheets (GO): an electrochemical sensing platform for the sensitive and simultaneous determination of benzocaine in the presence of antipyrine†
Received
1st July 2017
, Accepted 28th July 2017
First published on 10th August 2017
Abstract
An effective electrochemical sensing platform for the simultaneous determination of benzocaine (BEN) and antipyrine (ANT) based upon titanium dioxide nanoparticle (TiO2)/graphene oxide nanosheet (GO) bulk modified carbon paste electrodes (TiO2–GO/CPE) is reported. The TiO2–GO/CPE electrochemical sensing platform is found to exhibit linear ranges from 1.0 × 10−6 to 1.0 × 10−4 M and 1.2 × 10−8 to 8.0 × 10−5 M for BEN and ANT, respectively. The TiO2–GO/CPE sensor is explored towards the analysis of BEN and ANT in oral fluid (saliva) and pharmaceutical products. The synergy between the graphene oxide nanosheets and titanium dioxide nanoparticles results in a dramatic enhancement in the sensitivity of the sensor through a combination of increased surface area and improved electron transfer kinetics compared to other electrode alternatives. The fabricated TiO2–GO/CPE exhibits high sensitivity and good stability towards the sensing of BEN and ANT and has the potential to be utilised as a clinical assay and QA in pharmaceutical products.
1. Introduction
The Food and Drug Administration (FDA) issued a Public Health Advisory warning about the risk of methemoglobinemia (MHb) related to topical benzocaine used during medical procedures1 and the FDA has received over 70 cases of serious adverse events, with a number of deaths associated with MHb after the use of topical benzocaine, bringing the total reported cases to 319.2 Benzocaine (4-aminobenzoic ethyl ester) is a local anaesthetic and as such is commonly found in medicines for mild pain relief such as sore throat tablets and for reducing the pain in mucous membranes and teguments.
Various pharmaceutical products contain benzocaine, such as losenges, syrups, solutions, etc. used for the treatment of oral or tongue ulcerations, gastric ulcers, as well as ointments and suppositories used for treating hemorrhoidal disorders.3 Numerous experiments have shown that excessive doses of anaesthetic sprays containing BEN can result in cyanosis and life-threatening complications.4 Research findings have reported that the administration of BEN containing sprays in endoscopy, intubation, bronchoscopy, and similar invasive procedures may cause MHb, with potentially serious consequences.4 In addition, other research has revealed that a topical BEN 20% spray used in endoscopies can be absorbed and cause MHb.5 There is therefore a need to detect BEN in formulations for QA purposes as well as in the analysis of raw materials, drugs and pharmaceutical preparations in addition to developing bioassays/biomonitoring. Several quantitative analytical studies for quantifying BEN have been reported, involving high-performance liquid chromatography (HPLC),6–8 liquid chromatography-mass spectrometry (LC-MS),9,10 amperometric detection using a modified carbon-paste electrode8,11 and chemiluminescence.12,13
Antipyrine (ANT) and BEN are co-formulated in ear drops for pain relief and reduction of inflammation in the ear in otitis externa and acute otitis media.14 Antipyrine (1,5-dimethyl-2-phenyl-4-pyrazolin-3-one) is a non-steroidal anti-inflammatory drug (NSAID) that can relieve mild to moderate pain. ANT is an official drug as reported in the United States Pharmacopeia (USP)15 and British Pharmacopoeia (BP).16 Numerous analytical methods have been reported for the determination of ANT either alone or co-formulated with other drugs, such techniques can include spectrophotometric,17–20 chromatographic,21,22 thin-layer chromatography (TLC),23,24 gas chromatography (GC),25,26 capillary zone electrophoresis27 and non-aqueous titration28 methods. Due to the harmful side effects of BEN towards humans, there is a need for selective and fast highly efficient analytical protocols for the determination of trace BEN and its co-formulated drug antipyrine (ANT) in human fluids and pharmaceutical preparations.
Graphene oxide (GO) is being widely combined into an ever diversifying range of applications across several fields in the search for greatly improved device performance.29 One area that receives significant interest is electrochemistry where GO has been reported to be beneficial for various applications ranging from sensing through to energy storage/generation and carbon-based molecular electronics as well as water splitting.30–33 Additionally, GO can work as a building block for the construction of three dimensional (3D) graphene with various structures that can combine with other functional materials to yield new 3D-based hierarchical materials.30 Graphene oxide (GO) has a high adsorption capacity, large surface area and good biocompatibility. In particular, GO contains a large number of hydrophilic functional groups, such as –OH, –COOH and epoxides on the basal plane and the sheet edge,34–36 resulting in good hydrophilicity that makes it easily disperse in solvents with long-term stability. Due to these advantages, GO-based electrochemical sensors have been developed for the sensitive determination of various biological molecules.35 The existence of oxygen functionalities at the surface of GO is very interesting as they provide reactive sites for chemical modification using well-known carbon surface chemistry.37 One possible way to utilise the outstanding properties of GO in applications would be to integrate GO sheets in a composite material which is then applied as the basis for an electrochemical sensor.
In this paper, we have utilised the reported benefits of GO, to design and develop a GO bulk modified carbon-paste electrode. To provide further enhancements, we have additionally incorporated nanomaterials, in this case, titanium dioxide (TiO2) nanoparticles, due to their reported physicochemical properties such as good biocompatibility, strong adsorptive ability, high surface area, thermal stability, non-toxicity and electrical/electrochemical properties.38 To the best of our knowledge, and at the time of writing this, there are no studies reported to date that utilise TiO2–GO for the electrochemical determination of BEN and ANT. We consequently report the first ever example of using TiO2–GO/CPE electrodes for the bio-monitoring of BEN and ANT in oral fluid (saliva) and pharmaceutical products. The TiO2–GO/CPE based electrochemical sensing platform provides a simple, selective and reliable sensor for the simultaneous determination of BEN and ANT.
2. Experimental
2.1. Instrumentation
A Bio-logic SP 150 electrochemical workstation was used for all electrochemical measurements. A one compartment cell with a three electrode set-up was connected to the electrochemical workstation through a C3-stand from BAS (USA). A platinum wire from BAS (USA) was employed as the auxiliary electrode. All the cell potentials were measured with respect to an Ag/AgCl (3.0 M NaCl) reference electrode from BAS (USA). A Cyberscan 500 digital (EUTECH Instruments, USA) pH-meter with a glass combination electrode served to carry out pH measurements. All the electrochemical experiments were performed at an ambient temperature of 25 °C. Impedance spectra were recorded over a frequency range from 100 mHz to 100 kHz. Scanning electron microscopy (SEM) measurements were carried out using a JSM-6700F scanning electron microscope (Japan Electro Company). FT-IR spectra were recorded on an IR-Affinity-1 Fourier transform infrared spectrophotometer (Shimadzu, Japan). The crystalline phases were detected and identified using an X-ray diffractometer (XRD) on an X'Pert PRO MRD with a copper source at a scan rate (2θ) of 1° s−1. Sigma Plot 10.0 was used for all statistical data.
2.2. Chemicals and reagents
BEN was kindly supplied by Sabaa International Company (Egypt), purity: 99.98%. ANT was kindly supplied by Pharma Swede Egypt, purity: 99.88%. Graphite powder was obtained from Aldrich. The dosage form, Otosept ear drops (labelled to contain 300 mg of antipyrine and 100 mg of benzocaine) are manufactured by Amriya for pharmaceutical industries, Egypt. Zora-C lozenges (labelled to contain benzalkonium chloride 0.001 mL, benzocaine 1.0 mg and vitamin C 50.0 mg) is manufactured by CID Company. Titanium dioxide nanoparticles were obtained from Nano-Lab (Waltham, MA, USA). Paraffin oil from Merck was used as the pasting liquid for the preparation of the paste electrodes. Britton–Robinson buffer (BRB) was prepared by mixing different volumes of 0.04 M in H3PO4, 0.04 M acetic acid and 0.04 M boric acid with an appropriate amount of 0.2 M NaOH to obtain the desired pH 2.0–10.0. All solutions were prepared from analytical grade chemicals and sterilized Milli-Q deionized water was used.
2.3. Preparation of the GO–TiO2–CPE
Carbon paste unmodified electrodes (CPEs) were prepared by mixing graphite powder (0.5 g) with paraffin oil (0.3 mL) in a pestle and mortar. The carbon paste was packed into the hole of an electrode body and smoothed on filter paper until a shiny appearance was achieved. Graphene oxide (GO) was prepared by using Hummers’ method.39 TiO2 was dispersed with GO in water by adding 100.0 mg TiO2 and 100.0 mg GO in a 25 mL volumetric flask (1
:
1, wt%
:
wt%) and sonicated for 45 min to produce a homogeneous dispersion. The TiO2–GO nanocomposite was then left to dry at 80 °C in an oven for 4 hours. Next, 30.0 mg of the TiO2–GO nanocomposite (1
:
1) was mixed with 970.0 mg graphite. The paste was extensively mixed with an appropriate amount of paraffin oil (0.35 mL) for 40 min until a uniform and homogeneous wetted paste was obtained. This paste was packed into the hole of the electrode body and smoothed on filter paper until a shiny appearance occured. A new surface was obtained by pushing excess of the paste out of the syringe and polished with weighing paper. Other modified CPEs were prepared for comparative studies. Accordingly, TiO2/CPE and GO/CPE were made using 15.0 mg of TiO2 and GO separately with 985.0 mg of graphite. The same amount of paraffin oil was used for the preparation of partially bulk modified CPEs.
Prior to any voltammetric measurements, the modified TiO2–GO/CPE electrode was cycled between 0.0 and −1400 mV at a scan rate of 100 mV s−1 in a pH 2 BRB several times until a reproducible response was achieved. Following this, the modified TiO2–GO/CPE electrode was transferred into another cell containing pH 2 BRB.
2.4. Analysis of real samples
Otosept ear drops.
A volume of ear drops equivalent to 1.0 × 10−3 M BEN and ANT was transferred into a volumetric flask (10 mL) and made up to the mark with double distilled water. An appropriate dilution was made using double distilled water to obtain solutions of the final concentrations of BEN and ANT.
Five tablets were weighed and transferred to a clean, dry mortar. An accurately weighed amount equivalent to two tablets was dissolved in 10 mL of distilled water to give a solution containing 1.2 × 10−3 M BEN; this was then diluted to give 1.0 × 10−3 M BEN, which was then filtered through a Nylon membrane filter.
Oral fluid (saliva).
Collection of saliva samples was achieved using a salivette from a healthy volunteer who received repeated doses of Zora-C lozenges (once daily) within a pharmacokinetic study.40 The saliva samples were centrifuged for 5 min at 4000 rpm, then diluted to 5.0 mL with pH 2 BRB and were analysed using the standard addition method. The solution was transferred into the voltammetric cell to be analysed without any further pretreatment. All experiments were performed in compliance with the relevant laws and institutional guidelines, and the institutional ethics committees have approved these experiments.
3. Results and discussion
3.1 Electrochemical characterisation of the TiO2–GO/CPE
The GO–TiO2 used in the fabrication of the TiO2–GO/CPE electrochemical sensors is fully characterised and presented in the ESI (Fig. S1 and S2†) confirming the presence of nanometer-sized TiO2 supported on graphene oxide (GO) nanosheets where the GO serves to electrically wire and support the TiO2 electroactive centres. To demonstrate the benefits of using the TiO2–GO/CPE as the basis for a sensor, the electrochemical response was explored using not only the TiO2–GO/CPE, but also a bare CPE, TiO2/CPE and GO/CPE. The cyclic voltammetric responses are shown in Fig. 1A where in all cases, two voltammetric peaks are observed corresponding to the electrochemical oxidation of BEN and ANT. It is evident that the largest voltammetric response is observed with slight improvements in the voltammetric peak potentials using the TiO2–GO/CPE, which is likely due to a combination of increased surface area and favourable electrochemical activity largely from the TiO2 and GO. The electroactive area of each of the electrodes was calculated (see ESI Fig. S3†). The calculated areas were found to correspond to 0.055, 0.074, 0.095 and 0.144 cm2 for the CPE, TiO2/CPE, and GO/CPE, and TiO2–GO/CPE, respectively. This confirms that the TiO2–GO/CPE exhibits the largest electrode surface area.
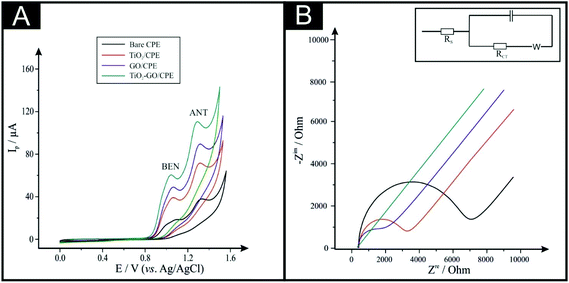 |
| Fig. 1 Cyclic voltammograms (A) of 1.0 mM BEN and ANT in BRB (pH 2.0) at a scan rate of 0.1 V s−1 utilising a CPE (black line), TiO2/CPE (red line), GO/CPE (purple line) and TiO2–GO/CPE (green line). Additionally shown (B) are the respective electrochemical impedance spectra in a 5.0 mM K3Fe(CN)6 (1 : 1) solution with a 0.1 M KCl supporting electrolyte. | |
Next, electrochemical impedance spectroscopy (EIS) was used to study the electrochemical response of the CPE, TiO2/CPE, and GO/CPE, and TiO2–GO/CPE; the results are depicted in Fig. 1B. In order to obtain detailed data of the impedance spectroscopy, a simple equivalent circuit model was used to fit the results. In this circuit, Rs, C and RCT represent solution resistance, a capacitance for the double-layer and electron transfer resistance, respectively. By fitting the data, the values of RCT were estimated to correspond to 4207.0 Ω at the bare CPE, which decreases to 2509.0 and 1877.0 Ω for the TiO2/CPE and GO/CPE, respectively, which is then observed to further decrease to 160.0 Ω for the TiO2–GO/CPE, indicating the significantly lower electron-transfer resistance of the TiO2–GO/CPE compared with other electrodes. The results are consistent with the CV results presented above, demonstrating that the TiO2–GO/CPE composite likely provides higher electron conduction pathways due to the synergistic effects of its constituents. Overall, the electrochemical results demonstrate that the TiO2–GO/CPE provides enhancements over other possible electrode alternatives.
The influence of solution pH upon the electrochemical oxidation of BEN and ANT using the TiO2–GO/CPE was next explored; pH is an important parameter, which can affect the electrochemical (analytical) sensitivity and voltammetric peak separation. Fig. S4† depicts the response upon the electrochemical oxidation of BEN and ANT from changing the solution pH from 2 to 8 which shows that the electrochemical oxidation peak potential for BEN and ANT is pH dependent as indicated by the following equations: BEN: Ep (V) = 1.17–0.062 pH (R2 = 0.992); ANT: Ep (V) = 1.45–0.052 pH (R2 = 0.991). Both responses are close to the anticipated Nernstian value of 59.2 mV for an electrochemical process with equal electrons and protons. Additionally, ESI Fig. S4† shows the response of the peak current as a function of pH where it is observed that pH 2 BRB gives rise to the largest peak currents/analytical signals for both BEN and ANT.
Furthermore, the effect of the voltammetric scan rate on the electrochemical signal of BEN and ANT was explored; these results are presented in ESI Fig. S5,† which demonstrate that the electrochemical oxidations of both BEN and ANT are diffusion-controlled processes. Additionally, chronoamperometric measurements were performed providing information on the diffusion coefficients, which were calculated to be 4.95 × 10−6 cm2 s−1 and 2.34 × 10−6 cm2 s−1, for BEN and ANT, respectively; further details are provided in the ESI.†
Lastly, the influence of the amount of TiO2 and GO used in the fabrication of the TiO2–GO/CPE on the magnitude of the analytical signal/peak current was explored. The anodic peak currents for both drugs were investigated towards changing the quantity of TiO2–GO over the range from 5.0 mg to 30.0 mg. The results indicated that the anodic peak current was optimal when the CPE was modified with a quantity equivalent to 30.0 mg of the TiO2–GO nanocomposite (15.0 mg of TiO2 and 15.0 mg of GO). More quantities of TiO2–GO increased the background current and poorer repeatability of the BEN and ANT response was observed.
3.2 Individual and simultaneous voltammetric determination of BEN and ANT using square wave voltammetry (SWV)
The electrochemical sensing of BEN and ANT was first explored at the TiO2–GO/CPE using SWV in pH 2 BRB individually, as shown in Fig. 2. From the inspection of Fig. 2 it is clear that the voltammetric response/analytical signal increases with increasing concentrations of BEN and ANT with the corresponding calibration plots shown as insets in Fig. 2. In the case of the electrochemical sensing of BEN, a linear response over the range from 1.0 × 10−6 to 1.0 × 10−4 M was found with the following linear regression: Ip (μA) = 0.193C (μM) + 0.845; R2 = 0.9991. The calculated limit of detection (LOD) was found to correspond to 2.48 × 10−7 M with a limit of quantitation (LOQ) of 8.26 × 10−7 M. For the sensing of ANT, a linear response was observed over the range from 1.20 × 10−8 to 8.0 × 10−5 M Ip (μA) = 0.353C (μM) + 1.930; R2 = 0.9992. The calculated values of the LOD and LOQ were found to correspond to 3.00 × 10−9 and 9.99 × 10−9, respectively, with repeatability and intermediate precisions of 0.81–0.90% and 0.95–1.09%, for BEN and ANT, respectively. Additionally the stability of the TiO2–GO/CPE was investigated, where the peak current was not found to change following storage in air for 30 days. The modified electrode retained 98.80–99.11% of its initial response.
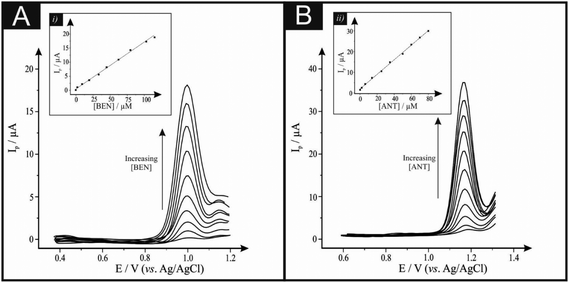 |
| Fig. 2 SWVs for BEN and ANT (A & B respectively) utilising the TiO2–GO/CPE in pH 2.0 BRB, with additional calibration plots for the individual detection of BEN (inset i) and ANT (inset ii). Note: BEN concentrations correspond to: 1.0 × 10−6 to 1.0 × 10−4 M and ANT concentrations correspond to: 1.20 × 10−8 to 8.0 × 10−5 M. | |
3.3 Simultaneous determination of BEN and ANT
To test the specificity of the TiO2–GO/CPE proposed sensor, its electroanalytical response was explored towards the sensing of BEN in the presence of the frequently co-formulated drug ANT. This was performed by simultaneously changing the concentrations of the drugs and recording the SWVs using the TiO2–GO/CPE. Fig. 3 depicts the voltammetric response of the TiO2–GO/CPE sensor towards the sensing of BEN in the presence of ANT. The results demonstrate well-defined anodic peaks at potentials of +1.01 and +1.22 V (vs. Ag/AgCl), corresponding to the oxidation of BEN and ANT, respectively, indicating that the simultaneous determination of these compounds is feasible. The inset of Fig. 3 shows the plot of the peak current (Ip) as a function of BEN and ANT concentrations. The calibration plots (inset) were linearly related to BEN and ANT over the ranges from 4.0 × 10−6 to 1.0 × 10−4 M and 2.9 × 10−6 to 73.5 × 10−6 M, respectively. The regression equations were: Ip (μA) = 0.192C (μM) + 0.507, R2 = 0.9978; and Ip (μA) = 0.349C (μM) + 0.684, R2 = 0.998; for BEN and ANT, respectively. The analytical sensitivities of the TiO2–GO/CPE sensor towards the sensing of BEN and ANT are found to be 0.193 and 0.353 μA μM−1, respectively. These values are very close to the value obtained in the absence of an interfering drug (0.192 and 0.349 μA μM−1, see Fig. 2), indicating that the electrochemical oxidation processes of these compounds on the surface of the TiO2–GO/CPE are independent and therefore, simultaneous determination of their mixtures is possible without significant interference.
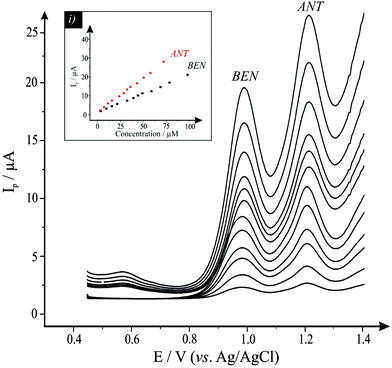 |
| Fig. 3 SWVs utilising a TiO2–GO/CPE sensing platform containing different concentrations of BEN and ANT correspond to 4.0 × 10−6 to 1.0 × 10−4 M and 2.9 × 10−6 to 73.5 × 10−6 M, respectively, within a pH 2.0 BRB. Scan rate: 0.1 V s−1. Inset i: calibration plots for the simultaneous detection of BEN and ANT. | |
3.4 Reproducibility and stability of the TiO2–GO/CPE
The reproducibility and stability of the TiO2–GO/CPE was examined by measuring the simultaneous electrochemical signal of 0.1 mM BEN and ANT. The relative standard deviation (RSD) for the peak current of BEN and ANT in six successive measurements was 1.9% and 2.2%, respectively, Fig. S8A.† In addition, the fabrication reproducibility was examined by using three modified sensors prepared independently, using the same method, and the RSD was 2.5% and 1.8% for BEN and ANT, respectively, as shown in Fig. S8B.† Also, the inter-day precision was deduced using different drug concentrations over a period of three days. Intra-day and inter-day precisions were expressed as the relative standard deviation (RSD%), Table S1.† For all concentration levels, the RSD didn't exceed 2.78%, revealing good precision of the TiO2–GO/CPE.
3.5 Analysis of real and spiked plasma samples
The applicability of the TiO2–GO/CPE for the sensing of BEN in oral fluid (saliva as the real sample), both BEN and ANT in ear drops (Otosept ear drops (300 mg ANT 100 mg BEN, spiked samples)) and BEN in Zora-C lozenges; 1 mg per tablet was explored. The concentrations were measured via the standard addition method. The results are shown in Table 1. The recovery values show good accuracy of the proposed TiO2–GO/CPE sensor. It is very clear that this TiO2–GO/CPE sensor has potential for the determination of trace amounts of these compounds in biological fluids and pharmaceutical products.
Table 1 Determination of BEN and ANT in pharmaceutical formulations and an oral fluid (saliva) sample using the new proposed sensing protocol
Sample |
Amount added standard (μM) |
Amount found (μM) |
Apparent recoverya % |
Average of three determinations.
|
Oral fluid (saliva) |
|
6.00 |
5.98 |
99.67% |
BEN |
30.00 |
29.78 |
99.27% |
|
90.00 |
89.94 |
99.93% |
Recovery% ± R.S.D |
99.62% ± 0.333 |
Otosept ear drops (300 mg ANT 100 mg BEN) |
|
4.00 |
3.99 |
99.75% |
BEN |
10.00 |
10.28 |
100.28% |
|
25.00 |
24.91 |
99.64% |
Recovery% ± R.S.D |
99.89% ± 0.342 |
|
|
12.00 |
12.10 |
100.83% |
ANT |
30.0 |
30.27 |
100.90% |
|
75.00 |
75.42 |
100.56% |
Recovery% ± R.S.D |
100.76% ± 0.179 |
Zora-C lozenges (1 mg per tablet) |
|
6.00 |
5.89 |
98.20% |
BEN |
20.00 |
19.73 |
98.65% |
|
85.00 |
84.86 |
99.84% |
Recovery% ± R.S.D |
98.89% ± 0.847 |
4. Conclusions
We have reported the simultaneous determination of BEN and ANT using a TiO2–GO/CPE based electrochemical sensing platform that provides a simple, selective and reliable sensor for simultaneous determination of TiO2–GO/CPE. We believe this to be the first example using this sensor towards the electrochemical detection of both BEN and ANT. The sensor is found to exhibit useful analytical ranges in a model matrix and is applied for the sensing of BEN and ANT in oral fluid (saliva) and pharmaceutical products. The TiO2–GO/CPE provides a simple, selective and economical sensor for BEN and ANT and precludes expensive and time-consuming pre-treatments such as those used in chromatographic methods.
References
- M. Taleb, Z. Ashraf, S. Valavoor and J. Tinkel, Am. J. Cardiovasc. Drugs, 2013, 13, 325–330 CrossRef CAS PubMed.
- S. Weiss-Smith, G. Deshpande, S. Chung and V. Gogolak, Arch. Intern. Med., 2011, 171, 591–593 CrossRef PubMed.
- G. Duţu, C. Cristea, B. Ede, V. Hârceagă, A. Saponar, E. Popovici and R. Săndulescu, Farmacia, 2011, 59, 147–160 Search PubMed.
- T. J. Moore, C. S. Walsh and M. R. Cohen, Arch. Intern. Med., 2004, 164, 1192–1196 CrossRef PubMed.
- M. S. Srikanth, R. Kahlstrom, K. H. Oh, S. R. Fox, E. R. Fox and K. M. Fox, Obes. Surg., 2005, 15, 584–590 CrossRef PubMed.
- J. R. Meinertz, G. R. Stehly, T. D. Hubert and J. A. Bernardy, J. Chromatogr. A, 1999, 855, 255–260 CrossRef CAS PubMed.
- P. Perez-Lozano, E. Garcia-Montoya, A. Orriols, M. Minarro, J. Tico and J. Sune-Negre, J. Pharm. Biomed. Anal., 2005, 39, 920–927 CrossRef CAS PubMed.
- H. Dejmkova, V. Vokalova, J. Zima and J. Barek, Electroanalysis, 2011, 23, 662–666 CAS.
- P. Scherpenisse and A. A. Bergwerff, Anal. Chim. Acta, 2007, 586, 407–410 CrossRef CAS PubMed.
- D. De Orsi, M. Pellegrini, E. Marchei, P. Nebuloni, B. Gallinella, G. Scaravelli, A. Martufi, L. Gagliardi and S. Pichini, J. Pharm. Biomed. Anal., 2009, 50, 362–369 CrossRef CAS PubMed.
- R. T. Kachoosangi, G. G. Wildgoose and R. G. Compton, Electroanalysis, 2008, 20, 2495–2500 CrossRef CAS.
- X. Zhang, W. Baeyens, G. Van der Weken, A. Calokerinos and K. Imai, Anal. Chim. Acta, 1995, 303, 137–142 CrossRef CAS.
- H. Paseková and M. Polášek, Talanta, 2000, 52, 67–75 CrossRef.
- J. M. Barbosa-Filho, M. R. Piuvezam, M. D. Moura, M. S. Silva, K. V. B. Lima, E. V. L. da-Cunha, I. M. Fechine and O. S. Takemura, Rev. Bras. Farmacogn., 2006, 16, 109–139 CrossRef CAS.
-
C. United States Pharmacopeial, The United States Pharmacopeia: USP 35: the National Formulary: NF30: by authority of the United States Pharmacopeial Convention, United States Pharmacopeial Convention Inc., Rockville, MD, 2012 Search PubMed.
-
C. British Pharmacopoeia, British Pharmacopoeia 2010, The Stationery Office, London, 2008 Search PubMed.
- G. Santoni, P. Mura, S. Pinzauti, E. Lombardo and P. Gratteri, Int. J. Pharm., 1990, 64, 235–238 CrossRef CAS.
-
H. A. Merey, Bulletin of Faculty of Pharmacy, Cairo University, 2016, vol. 54, pp. 181–189 Search PubMed.
- M. a. S. Collado, V. c. E. Mantovani, H. C. Goicoechea and A. C. Olivieri, Talanta, 2000, 52, 909–920 CrossRef CAS PubMed.
- M. É. Ribone, A. P. Pagani and A. C. Olivieri, Anal. Lett., 2001, 34, 2077–2088 CrossRef CAS.
- C. Atsriku, D. Watson, J. Tettey, M. Grant and G. Skellern, J. Pharm. Biomed. Anal., 2002, 30, 979–986 CrossRef CAS PubMed.
- M. Zuo, G. L. Duan and Z. G. Ge, Biomed. Chromatogr., 2004, 18, 752–755 CrossRef CAS PubMed.
- M. El Sadek, A. El Shanawany, A. A. Kheir and G. Rücker, J. Pharm. Biomed. Anal., 1991, 9, 87–89 CrossRef CAS PubMed.
- I. D. Wilson, Ther. Drug Monit., 1996, 18, 484–492 CrossRef CAS PubMed.
- G. Engel, U. Hofmann and M. Eichelbaum, J. Chromatogr. B: Biomed. Sci. Appl., 1995, 666, 111–116 CrossRef CAS PubMed.
- M. Moeder, S. Schrader, M. Winkler and P. Popp, J. Chromatogr. A, 2000, 873, 95–106 CrossRef CAS PubMed.
- D. Perrett and G. A. Ross, J. Chromatogr., A, 1995, 700, 179–186 CrossRef CAS PubMed.
- I. Shukrallah and A. Sakla, J. Chin. Chem. Soc., 1986, 33, 221–225 CrossRef CAS.
-
D. A. Brownson and C. E. Banks, The handbook of graphene electrochemistry, Springer, 2014 Search PubMed.
- Q. Quan, X. Lin, N. Zhang and Y.-J. Xu, Nanoscale, 2017, 9, 2398–2416 RSC.
- N. Zhang, M.-Q. Yang, S. Liu, Y. Sun and Y.-J. Xu, Chem. Rev., 2015, 115, 10307–10377 CrossRef CAS PubMed.
- M.-Q. Yang, N. Zhang, Y. Wang and Y.-J. Xu, J. Catal., 2017, 346, 21–29 CrossRef CAS.
- Y. Zhang, Z.-R. Tang, X. Fu and Y.-J. Xu, ACS Nano, 2010, 4, 7303–7314 CrossRef CAS PubMed.
- S. Stankovich, D. A. Dikin, R. D. Piner, K. A. Kohlhaas, A. Kleinhammes, Y. Jia, Y. Wu, S. T. Nguyen and R. S. Ruoff, Carbon, 2007, 45, 1558–1565 CrossRef CAS.
- M. A. Mohamed, A. M. Yehia, C. E. Banks and N. K. Allam, Biosens. Bioelectron., 2017, 89(Part 2), 1034–1041 CrossRef CAS PubMed.
- M. A. Mohamed, S. A. Atty, N. N. Salama and C. E. Banks, Electroanalysis, 2017, 29, 1038–1048 CrossRef CAS.
- J. Shen, B. Yan, M. Shi, H. Ma, N. Li and M. Ye, J. Mater. Chem., 2011, 21, 3415–3421 RSC.
- X. Chen and S. S. Mao, Chem. Rev., 2007, 107, 2891–2959 CrossRef CAS PubMed.
- W. S. Hummers Jr. and R. E. Offeman, J. Am. Chem. Soc., 1958, 80, 1339–1339 CrossRef.
- P. Maffei, S. L. Borgia, A. Sforzini, A. Yasin, C. Ronchi and G. Ceschel, J. Drug Delivery Sci. Technol., 2004, 14, 363–372 CrossRef CAS.
Footnote |
† Electronic supplementary information (ESI) available. See DOI: 10.1039/c7an01101f |
|
This journal is © The Royal Society of Chemistry 2017 |
Click here to see how this site uses Cookies. View our privacy policy here.