DOI:
10.1039/C7SC03124F
(Edge Article)
Chem. Sci., 2017,
8, 6904-6910
Stereochemistry and biological activity of chlorinated lipids: a study of danicalipin A and selected diastereomers†
Received
17th July 2017
, Accepted 7th August 2017
First published on 9th August 2017
Abstract
The syntheses of (+)-16-epi- and (+)-11,15-di-epi-danicalipin A (2 and 3) are reported. The conformations of the parent diols 5 and 6 as well as the corresponding disulfates 2 and 3 were determined on the basis of J-based configuration analysis and supported by calculations. The impact of configuration on membrane permeability in Gram-negative bacteria and mammalian cell lines was assessed as well as cytotoxicity. Although diastereomer 2 showed similar behavior to natural (+)-danicalipin A (1), strikingly, the more flexible C11,C15-epimer 3 had no effect on permeability and proved equally or more toxic towards multiple cell lines.
Introduction
(+)-Danicalipin A (1) is a prominent member of the chlorosulfolipid family of natural products isolated in the 1960's from microalgae, which were later anecdotally associated with seafood poisoning (Fig. 1A). More recently, it has captured the interest of research groups globally.1,2 Hexachlorosulfolipid 1 is the main polar component in the flagellar membrane of the golden-brown alga Ochromonas danica. The presence of other unusual, rare lipids renders its membrane a fascinating system for study.3 Coinciding with our interest in halogenated compounds of relevance to drug discovery and halosulfolipids, we set out to identify ways of investigating the chemical properties and biological activities of (+)-danicalipin A (1).4,5 Halogenation is known to influence electronic properties, lipophilicity, and metabolic stability of bioactive molecules.6,7 Additional subtle effects may be manifest in conformational preferences8 especially in aliphatic systems as notably highlighted by Hoffmann,8b O'Hagan,8c and more recently by Gademann.8d We were interested in the question: Do configurational isomers 2 and 3 (Fig. 2B and C) exhibit differences, chemically or biologically, from the natural product? Herein we report the syntheses of diastereomers 2 and 3 and accompanying structure–activity studies. Strikingly, a significant deviation of toxicity and membrane permeability was revealed and correlated to flexibility.
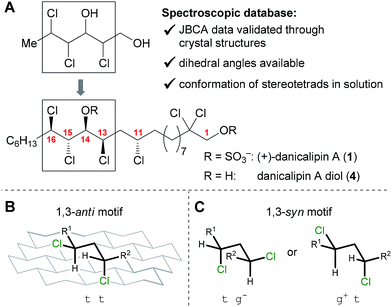 |
| Fig. 1 Identification of interesting danicalipin A isomers. (A) Information of the stereotetrad set by a spectroscopic database. (B) Conformation of the 1,3-anti motif (R1 ≠ R2). (C) Possible conformations of the 1,3-syn motif (R1 ≠ R2). | |
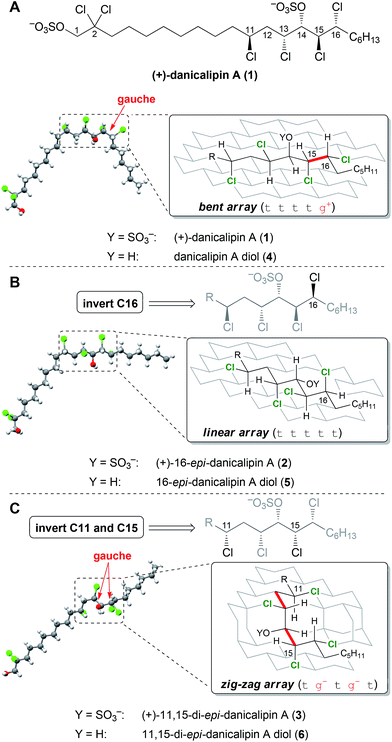 |
| Fig. 2 (A) (+)-Danicalipin A (1) and danicalipin A diol (4), (B) (+)-16-epi-danicalipin A (2) and 16-epi-danicalipin A diol (5), (C) (+)-11,15-di-epi-danicalipin A (3) and 11,15-di-epi-danicalipin A diol (6); R = (CH2)8(CCl2)CH2OY. Lowest-energy structures (DFT) of diols 4, 5, and 6 shown in ball-and-stick models; 3D representation of the C11 to C16 array superimposed on a diamond lattice using JBCA and DFT (along the principle chain: g+ = +60°, g− = −60°, t = 180°). | |
Many biologically active natural products feature characteristic configurational patterns that enable seemingly flexible molecules to adopt defined shapes.9 For example, the configuration of polyketides has been linked to their biological mode of action. Vicinal dichlorides and chlorohydrins are known to be conformationally biasing in simple systems when compared to the parent hydrocarbons.10,11 In contrast to polyketides, complex polychlorinated natural products have not been the focus of stereochemical investigations to date. The enigmatic biological role of (+)-danicalipin A (1) and its structure, in particular its chlorination pattern, render it an ideal target to probe a link, if any, between configuration and bioactivity. Therefore, we set out to identify diastereomers whose conformations would differ from 1.
Results and discussion
Target selection
Collectively, there are 34
992 possible staggered conformations (C10 to C17) for the 16 diastereomers of (+)-danicalipin A (1, 2187 conformers per diastereomer). Consideration of previous work suggests that various derivatives of 1 (Y = H, SO3−, Ac, TBS) have similar conformations.12 Consequently, the parent diols were chosen as the focus of our efforts in this study. Utilization of a conformational database of stereodefined trichlorinated hexane-1,3-diols10,13 (Fig. 1) narrowed the selection of low-energy conformers centered around the C13 to C16 stereotetrad to 18 structures (see ESI†).
According to models generated from the database, 5 and 6 were suggested to display defined structures different from danicalipin A diol (4, Fig. 2). Examination of models for 4, 5, and 6 revealed one, zero, and two gauche interactions in the C11 to C16 region, respectively. Conformational DFT analysis of 4 led to a structure that was in agreement with one produced from a solution-state NMR study (ttttg+).14–16 Calculations also supported the predicted all-trans arrangement in the chlorinated segment of 16-epi-danicalipin A diol (5, ttttt). A priori, substituents in a 1,3-syn relationship (cf. C11 to C13 in 6) ought to give rise to two energetically similar conformers (tg− or g+t, Fig. 1).9c,d,17 Yet, in 11,15-di-epi-danicalipin A diol (6), computations showed a preference for one (tg−tg−t). In order to gain insight into their solution-state conformations, diols 5 and 6 were prepared through de novo synthesis.
Syntheses of diastereomers
In prior syntheses of (+)-danicalipin A (1), the anti-relative configuration of the C15,C16-vic-dichloride employed trans-olefin dichlorination18a,b,d,e or cis-epoxide2a,18c opening (Fig. 3A). The anti-configuration of the C14,C15-chlorohydrin was set by substrate-controlled diastereoselective transformations (Fig. 3B).2a,18 The relative configuration present in 2/5 and 3/6 precluded the implementation of these earlier strategies (Fig. 3C).
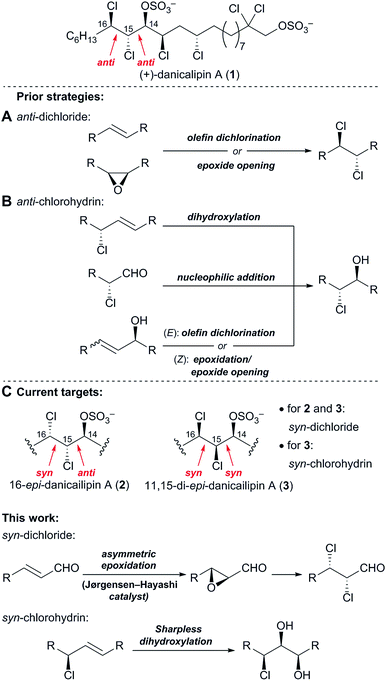 |
| Fig. 3 (A) Prior strategies to access the anti-dichloride and (B) the anti-chlorohydrin in (+)-danicalipin A (1), (C) different configurations in diastereomers 2 and 3. | |
Synthesis of 16-epi-danicalipin A.
The synthesis of (+)-16-epi-danicalipin A (2) (Scheme 1) commenced with the asymmetric organocatalytic epoxidation of (E)-non-2-enal (7) using the (S)-Jørgensen–Hayashi catalyst,19 followed by reduction and protection of the hydroxy group as its pivaloate ester. The enantiomeric ratio was determined to be >20
:
1 by Mosher ester analysis of the free alcohol (not shown).
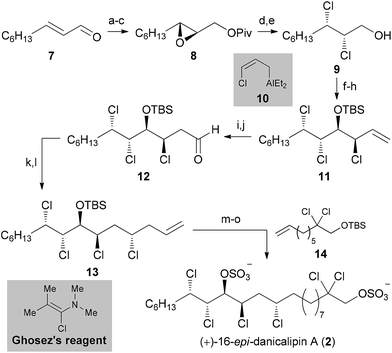 |
| Scheme 1 Reagents and conditions: (a) (S)-Jørgensen–Hayashi catalyst (10 mol%), H2O2, CH2Cl2, r.t., e.r. > 20 : 1; (b) NaBH4, MeOH, 0 °C, 62% over 2 steps; (c) PivCl, pyridine, CH2Cl2, r.t., 82%; (d) PPh3, NCS, toluene, 90 °C; (e) (iBu)2AlH, CH2Cl2, −78 °C, 78% over 2 steps; (f) DMP, NaHCO3, CH2Cl2, r.t.; (g) 10, THF, −78 °C, d.r. > 6 : 1; (h) TBSOTf, Et3N, CH2Cl2, r.t., 47% over 3 steps; (i) Cy2BH, THF, 0 °C, then NaBO3·4H2O, r.t., 69%; (j) DMP, CH2Cl2, r.t.; (k) (+)-Ipc2B(allyl), THF, −100 °C to r.t., 83% over 2 steps, d.r. > 5 : 1; (l) Ghosez's reagent, CHCl3, then Et3N, r.t., 86%; (m) 14 (3 equiv.), Grubbs II (10 mol%), CH2Cl2, 45 °C, then PtO2 (10 mol%), H2 (1 atm), r.t., 90%; (n) AcCl, MeOH, 80 °C, 84%; (o) SO3·pyridine, THF, r.t., 87%. | |
Opening of epoxide 8 with NCS and PPh3 in toluene at 90 °C afforded a vicinal dichloride,20a which was deprotected using (iBu)2AlH to give 9. Dess–Martin oxidation of alcohol 9 afforded an unstable and highly volatile α,β-dichloroaldehyde, which was directly subjected to chloroallylation conditions. For this purpose, γ-chloroallylaluminum reagent 10 was generated in situ from allyl chloride, LiTMP and Et2AlCl.21 As expected, the stereochemical outcome followed both the Felkin–Anh and Cornforth models with d.r. > 6
:
1. Protection of the secondary hydroxy group provided trichloride 11. Hydroboration and subsequent oxidation furnished unstable aldehyde 12. Brown allylation was performed using (+)-Ipc2BCl and allylmagnesium bromide in THF at −100 °C.22 The homoallylic alcohol was obtained in high yield (83%) and d.r. (>5
:
1).
Based on the knowledge gained in our research group,2a this allylic hydroxy group was converted to the corresponding chloride in high yield and with complete inversion utilizing Ghosez's reagent.23 This highly reactive α-chloroenamine is proposed to be ionized in situ to a keteneiminium ion, which is readily attacked by nucleophiles (e.g. the free secondary hydroxy group at C11). Hence, fragment 13 was synthesized in 12 steps from commercially available material. One-pot cross metathesis/hydrogenation with known olefin 14 (ref. 2a) established the complete C22 chain.24 The two TBS groups were removed with an excess of acetyl chloride in MeOH at 80 °C. Final sulfation with SO3·pyridine gave (+)-16-epi-danicalipin A (2) in 87% yield.
Synthesis of 11,15-di-epi-danicalipin A.
In a similar manner, the synthesis of (+)-11,15-di-epi-danicalipin A (3) (Scheme 2) commenced by epoxidizing (E)-non-2-enal (7) enantioselectively19 and subjecting the formed epoxyaldehyde to stabilized Wittig reagent 15. Subsequent epoxide opening under modified Yoshimitsu's conditions20b (PPh2Cl/NCS in CH2Cl2) gave vicinal dichloride 16 in 45% yield, along with 28% of an elimination product (see ESI†). To overcome the inherent preference of the molecule for anti-functionalization,18 α,β-unsaturated ester 16 was dihydroxylated under catalyst-controlled Sharpless' conditions25 in high yield (70%) and d.r. (8
:
1) favoring the all-syn product. Prolonged reaction times furnished substantial amounts of elimination products, further demonstrating the instability of the all-syn motif. Surprisingly, after selective nosylation of the α-hydroxy group26cis-epoxide 17 directly formed in situ. Reduction of the ester with (iBu)2AlH was followed by Wittig reaction to give a terminal olefin. According to previous results by our group,4a,27 epoxide opening with TMSCl in EtOAc occurred with inversion of configuration (d.r. = 8
:
1).
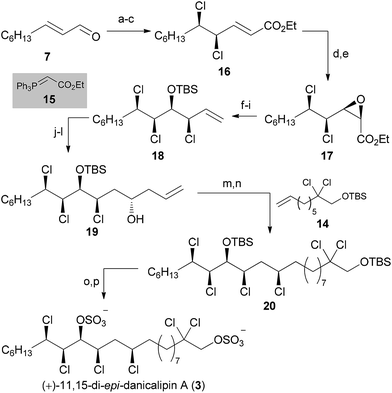 |
| Scheme 2 Reagents and conditions: (a) (R)-Jørgensen–Hayashi catalyst (10 mol%), H2O2, CH2Cl2, r.t., e.r. > 20 : 1; (b) 15, CH2Cl2, 0 °C, 61% over 2 steps; (c) NCS, Ph2PCl, CH2Cl2, r.t., 45%; (d) AD-mix β, NaHCO3, MeSO2NH2, tBuOH/H2O (1 : 1), 0 °C, 70%, d.r. = 6 : 1; (e) p-nitrobenzenesulfonyl chloride, Et3N, CH2Cl2, 0 °C, 74%; (f) (iBu)2AlH, toluene, −78 °C; (g) MePPh3Br, KN(TMS)2, THF −78 to 0 °C, 91% over 2 steps; (h) TMSCl, EtOAc; r.t.; (i) TBSOTf, Et3N, CH2Cl2, r.t., 76%, d.r. = 8 : 1; (j) Cy2BH, THF, 0 °C to r.t., then NaBO3·4H2O, 0 °C to r.t., 85%; (k) DMP, CH2Cl2, r.t.; (l) (−)-Ipc2B(allyl), THF, −100 °C to r.t., then NaBO3·4H2O, 0 °C to r.t., 73% over 2 steps, d.r. > 10 : 1; (m) 14 (3 equiv.), Grubbs II (10 mol%), CH2Cl2, 45 °C, then PtO2 (10 mol%), H2 (1 atm), 66%; (n) Ghosez's reagent, CHCl3, 0 °C to r.t., then Et3N, 0 °C to r.t., 78%; (o) AcCl, MeOH, 80 °C, 93%; (p) SO3·pyridine, THF, r.t., quant. | |
With a route to all-syn trichloride 18, we pursued the same approach as for (+)-16-epi-danicalipin A (2). Thus, hydroboration/oxidation preceded Dess–Martin oxidation and Brown allylation.22 When homoallylic alcohol 19 was subjected to Ghosez's reagent,23 an inseparable 1
:
1 mixture of tetrachloride and conjugated diene was isolated (not shown).28 Although this mixture could then be taken forward to 20, a higher yielding procedure resulted from cross metathesis of homoallylic alcohol 19 with 14 using Grubbs 2nd generation catalyst, and direct reduction of the olefin.24 The secondary alcohol could then be substituted using Ghosez's reagent, without concomitant elimination. Deprotection and sulfation of 20 was then achieved via the same procedure as for (+)-16-epi-danicalipin A (2).29
Biological investigations
The consequence of the configurational differences in disulfates 1, 2, and 3 on biological activity was then examined. We have previously reported that (+)-danicalipin A (1) affects the integrity of cell membranes in Gram-negative bacteria (E. coli DH5α) and mammalian cell lines (Hepa 1–6, HT-29).2a
In the assay, incubation of E. coli with 1 compromised bacterial membranes, an effect quantified by measuring an increase in fluorescence of a DNA stain.30 At 125 μM concentrations of (+)-danicalipin A (1), incorporation of Hoechst 33342 into E. coli was increased 5-fold over the negative control (Fig. 4),31 while incubation with (+)-16-epi- danicalipin A (2) showed a 3-fold increase. Remarkably, no permeability enhancement was observed with (+)-11,15-di-epi-danicalipin A (3) even at toxic concentrations ≥ 250 μM (see ESI†).
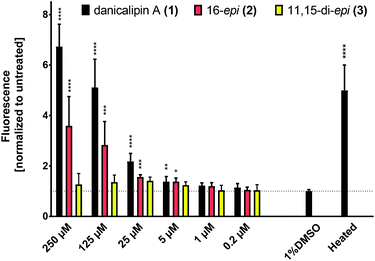 |
| Fig. 4 Membrane permeability enhancement in bacteria: fluorescence response due to nuclear staining of E. coli DH5α by Hoechst 33342 as a function of the concentration of 1, 2, or 3 as well as positive and negative control experiments. Data are normalized to the untreated results. The significance of each result vs. DMSO is shown above the bar: p < 0.033 = *; p < 0.01 = **; p < 0.001 = ***; p < 0.0002 = ****. | |
Additional experiments were conducted in Hepa 1–6 murine liver cells to assess permeability enhancement (Table 1). At 25 μM concentrations, both (+)-danicalipin A (1) and (+)-16-epi-danicalipin A (2) compromised cell membranes as shown with a Hoechst 33342/Sytox Green assay (cf. A/B with C/D),2a,32 consistent with positive control (20% EtOH, see ESI†). By contrast, using the same assay, we observed that (+)-11,15-di-epi-danicalipin A (3) resulted in minimal Sytox Green staining (E/F, Table 1) similar to negative control (1% DMSO, see ESI†).
Table 1 Membrane permeability enhancement in mammalian cellsa
|
(+)-Danicalipin A (1) |
(+)-16-epi-Danicalipin A (2) |
(+)-11,15-Di-epi-danicalipin A (3) |
Fluorescent images of Hepa 1–6 cells after incubation with compounds 1, 2, and 3 at 25 μM concentration. Blue: sampled cells, visualized with Hoechst 33342. Green: cells with compromised cell membranes, visualized with Sytox Green.33
|
Hoechst 33342 (sampled cells) |
|
|
|
Sytox Green (cells with compromised membranes) |
|
|
|
To enable direct comparison with other known halosulfolipids and analogs toxicity towards brine shrimp was examined.2,15,18b,c The observed LC50 values, 1
:
2.5 μM; 2
:
5.7 μM; 3
:
4.5 μM, indicate that configuration has no influence on brine shrimp toxicity. Interestingly, the fact that all diastereomers (1–3) are significantly more toxic than the parent non-chlorinated lipid (1,14-docosane disulfate)2a emphasizes the importance of chlorination on activity. In mammalian-cell toxicity assays 1–3 showed similar EC50 values against Hepa 1–6 and A549 cell lines (see ESI†). However, C11,C15-epimer 3 was noticeably more toxic towards HT-29 cells (3.7 ± 0.6 μM) than both C16-epimer 2 (10.9 ± 0.1 μM) and (+)-danicalipin A (1, 14.7 ± 0.4 μM).
Given the biological data, the answer to the question serving as the focus of this study is yes: configurational isomers 1–3 display differential biological profiles. We were then interested in understanding the nature of the relationship between observed bioactivity and configuration. The data resulting from investigation of disulfates 1–3 by NMR spectroscopy was insufficient to confidently determine their solution-state conformations. In order to elucidate the limited spectroscopic data, additional DFT analyses were undertaken.34 The calculated conformations of the chlorinated segments in 1 and 2 were identical to those found in diols 4 and 5 (Fig. 2), respectively. These were also consistent with data obtained from NMR spectra. Interestingly, computational analysis of 3 revealed a small energy difference (ΔΔG = 0.1 kcal mol−1) between two conformations along C11–C13.35 The inconclusive spectroscopic data in conjunction with DFT analysis suggests 3 exists as a mixture of rapidly interconverting conformers (see ESI†). Thus, while the chlorinated segments of 1 and 2 have defined minima in solution, the syn-C11,C13-configuration promotes flexibility for 3.
The biological observations indicate that the configuration of naturally-occurring (+)-danicalipin A (1) plays a pronounced role in structurally compromising phospholipid-based membranes, such as those in E. coli, murine, and human cell lines. This feature may be relevant to its unspecified role in the membrane of O. danica. In this respect, the membrane domains in spermatozoa from the fish Sparus aurata have been reported to consist of varying proportions of (poly)unsaturated fatty acids in accordance with their function. Specifically, a high content of such lipids in the flagellar membrane is correlated with improved sperm viability and motility, as well as increased membrane fluidity.36 We speculate that the chlorinated array in 1 acts in analogy to cis-unsaturation in fatty acids by introducing a kink in the lipid chain.37 Consequently, we hypothesize that 1 confers similar effects on motility in the flagellum of O. danica.38 This suggests new directions to advance understanding of this unicellular organism.
Conclusions
In conclusion, we have synthesized two unnatural diastereomers of (+)-danicalipin A (1), namely, 16-epi- and 11,15-di-epi-danicalipin A (2 and 3). These were selected by a combined database/computational approach to examine, for the first time, the effect of complex chlorination patterns on chemical and biological properties. We observed a striking result in which one of the diastereomers displays a significantly different biological profile when compared to the natural product, which can be correlated to solution-state flexibility. Thus, 1 and 2 had an effect on permeability, while 3 displayed no such activity. Yet, 3 was more toxic against HT-29 cell lines. Noteworthy, comparison of the biological data for 1 and 3 further reveals that there is no obvious link between toxicity and permeability enhancement. Our work underscores that the configurational pattern of chlorinated lipids influences the conformational landscape and also impacts biological profiles. More broadly, stereodefined chlorinated arrays may find use as conformationally biasing elements with applications in materials science and medicinal chemistry.
Conflicts of interest
There are no conflicts to declare.
Acknowledgements
We are grateful to Dr Stefan Ruider for assistance with DFT calculations, Dr M.-O. Ebert, R. Arnold, R. Frankenstein, P. Zumbrunnen and S. Burkhardt for NMR measurements, and to the European Research Council (320666-CHLIP) for financial support.
Notes and references
- For reviews on chlorosulfolipids, see:
(a) D. K. Bedke and C. D. Vanderwal, Nat. Prod. Rep., 2011, 28, 15 RSC;
(b) C. Nilewski and E. M. Carreira, Eur. J. Org. Chem., 2012, 1685 CrossRef CAS;
(c) T. Umezawa and F. Matsuda, Tetrahedron Lett., 2014, 55, 3003 CrossRef CAS;
(d) For a review on shellfish toxins, see: P. Ciminiello and E. Fattorusso, Eur. J. Org. Chem., 2004, 2533 CrossRef CAS.
-
(a) A. M. Bailey, S. Wolfrum and E. M. Carreira, Angew. Chem., Int. Ed., 2016, 55, 639 CrossRef CAS PubMed;
(b) S. Fischer, N. Huwyler, S. Wolfrum and E. M. Carreira, Angew. Chem., Int. Ed., 2016, 55, 2555 CrossRef CAS PubMed.
- The novelty of the membrane composition of O. danica has raised significant questions and stimulated debate regarding its ability to self-assemble.37a In addition to chlorosulfolipids, other major components include rare betaine lipids, such as 1(3),2-diacylglyceryl-(3)-O-4′-(N,N,N-trimethyl)homoserine, incorporating saturated as well as unsaturated C14–C20 fatty acids, and sterols, with poriferasterol as the most abundant. None are commercially available or are easily accessible. For the isolation of these membrane components, see:
(a) A. E. Brown and J. Elovson, Biochemistry, 1974, 13, 3476 CrossRef CAS PubMed;
(b) M. G. Gershengorn, A. R. H. Smith, G. Goulston, L. J. Goad, T. W. Goodwin and T. H. Haines, Biochemistry, 1968, 7, 1698 CrossRef CAS PubMed.
-
(a) C. Nilewski, R. W. Geisser and E. M. Carreira, Nature, 2009, 457, 573 CrossRef CAS PubMed;
(b) C. Nilewski, N. R. Deprez, T. C. Fessard, D. B. Li, R. W. Geisser and E. M. Carreira, Angew. Chem., Int. Ed., 2011, 50, 7940 CrossRef CAS PubMed.
-
(a) Q. A. Huchet, B. Kuhn, B. Wagner, H. Fischer, M. Kansy, D. Zimmerli, E. M. Carreira and K. Müller, J. Fluorine Chem., 2012, 152, 119 CrossRef;
(b) M. V. Westphal, B. T. Wolfstädter, J.-M. Plancher, J. Gatfield and E. M. Carreira, ChemMedChem, 2015, 10, 461 CrossRef CAS PubMed;
(c) A. Joliton and E. M. Carreira, Synlett, 2015, 26, 737 CrossRef CAS;
(d) C. Nilewski, C. Le Chapelain, S. Wolfrum and E. M. Carreira, Org. Lett., 2015, 17, 5602 CrossRef CAS PubMed;
(e) Q. A. Huchet, B. Kuhn, B. Wagner, N. A. Kratochwil, H. Fischer, M. Kansy, D. Zimmerli, E. M. Carreira and K. Müller, J. Med. Chem., 2015, 58, 9041 CrossRef CAS PubMed;
(f) A. Joliton, J.-M. Plancher and E. M. Carreira, Angew. Chem., Int. Ed., 2016, 55, 2113 CrossRef CAS PubMed;
(g) R. Vorberg, N. Trapp, D. Zimmerli, B. Wagner, H. Fischer, N. A. Kratochwil, M. Kansy, E. M. Carreira and K. Müller, ChemMedChem, 2016, 11, 2216 CrossRef CAS PubMed;
(h) Q. A. Huchet, W. B. Schweizer, B. Kuhn, E. M. Carreira and K. Müller, Chem.–Eur. J., 2016, 22, 16920 CrossRef CAS PubMed.
- For an overview of halogenated natural products and their biosynthesis, see:
(a) F. H. Vaillancourt, E. Yeh, D. A. Vosburg, S. Garneau-Tsodikova and C. T. Walsh, Chem. Rev., 2006, 106, 3364 CrossRef CAS PubMed;
(b) C. S. Neumann, D. G. Fujimori and C. T. Walsh, Chem. Biol., 2008, 15, 99 CrossRef CAS PubMed;
(c) C. Wagner, M. El Omari and G. M. König, J. Nat. Prod., 2009, 72, 540 CrossRef CAS PubMed;
(d) A. Butler and M. Sandy, Nature, 2009, 460, 848 CrossRef CAS PubMed;
(e) C. Paula and G. Pohnert, Nat. Prod. Rep., 2011, 28, 186 RSC;
(f) W.-j. Chung and C. D. Vanderwal, Angew. Chem., Int. Ed., 2016, 55, 4396 CrossRef CAS PubMed;
(g) V. Weichold, D. Milbredt and K.-H. van Pée, Angew. Chem., Int. Ed., 2016, 55, 6374 CrossRef CAS PubMed.
-
(a) E. Rodrigues Pereira, L. Belin, M. Sancelme, M. Prudhomme, M. Ollier, M. Rapp, D. Sevère, J.-F. Riou, D. Fabbro and T. Meyer, J. Med. Chem., 1996, 39, 4471 CrossRef PubMed;
(b) K. Naumann, J. Prakt. Chem., 1999, 341, 417 CrossRef CAS;
(c) S. Krautwald, C. Nilewski, M. Mori, K. Shiomi, S. Ōmura and E. M. Carreira, Angew. Chem., Int. Ed., 2016, 55, 4049 CrossRef CAS PubMed;
(d) B. Gál, C. Bucher and N. Z. Burns, Mar. Drugs, 2016, 14, 206 CrossRef PubMed.
-
(a) C. M. Harris, R. Kannan, H. Kopecka and T. M. Harris, J. Am. Chem. Soc., 1985, 107, 6652 CrossRef CAS;
(b) R. W. Hoffmann, D. Stenkamp, T. Trieselmann and R. Göttlich, Eur. J. Org. Chem., 1999, 2915 CrossRef CAS;
(c) D. O'Hagan, J. Org. Chem., 2012, 77, 3689 CrossRef PubMed;
(d) M. Scherer, D. Bezold and K. Gademann, Angew. Chem., Int. Ed., 2016, 55, 9427 CrossRef CAS PubMed;
(e) F. Scheidt, P. Selter, N. Santschi, M. C. Holland, D. V. Dudenko, C. Daniliuc, C. Mück-Lichtenfeld, M. R. Hansen and R. Gilmour, Chem.–Eur. J., 2017, 23, 6142 CrossRef CAS PubMed.
-
(a) P. W. Smith and W. C. Still, J. Am. Chem. Soc., 1988, 110, 7917 CrossRef CAS;
(b) X. Wang, S. D. Erickson, T. Iimori and W. C. Still, J. Am. Chem. Soc., 1992, 114, 4128 CrossRef CAS;
(c) R. W. Hoffmann, Angew. Chem., Int. Ed., 1992, 31, 1124 CrossRef;
(d) R. W. Hoffmann, Angew. Chem., Int. Ed., 2000, 39, 2054 CrossRef;
(e) A. Wei, K. M. Boy and Y. Kishi, J. Am. Chem. Soc., 1995, 117, 9432 CrossRef CAS;
(f) S. Higashibayashi, W. Czechtizky, Y. Kobayashi and Y. Kishi, J. Am. Chem. Soc., 2003, 125, 14379 CrossRef CAS PubMed;
(g) D. L. Boger, T. M. Ramsey, H. Cai, S. T. Hoehn and J. Stubbe, J. Am. Chem. Soc., 1998, 120, 9149 CrossRef CAS;
(h) M. Burns, S. Essafi, J. R. Bame, S. P. Bull, M. P. Webster, S. Balieu, J. W. Dale, C. P. Butts, J. N. Harvey and V. K. Aggarwal, Nature, 2014, 513, 183 CrossRef CAS PubMed.
- C. Nilewski, R. W. Geisser, M.-O. Ebert and E. M. Carreira, J. Am. Chem. Soc., 2009, 131, 15866 CrossRef CAS PubMed.
-
(a) N. L. Borkar, C. A. Kingsbury and E. P. Rack, J. Mol. Struct., 1988, 178, 287 CrossRef CAS;
(b) A. Chung-Phillips, J. Mol. Struct.: THEOCHEM, 1992, 257, 417 CrossRef.
- For examples: see ESI† of ref. 2b, 15, and this work.
- JBCA allows the determination of the predominant
conformation in solution through measurement of homo- and hetereonuclear coupling constants; see: N. Matsumori, D. Kaneno, M. Murata, H. Nakamura and K. Tachibana, J. Org. Chem., 1999, 64, 866 CrossRef CAS PubMed.
- DFT calculations were performed with Gaussian 09 for structures obtained from MacroModel on a M06-2X/6-311++G**/LANL2DZpd(Cl)/SMD(chloroform)//M06-2X/6-31+G**/LANL2DZpd(Cl)/SMD(chloro-form) level of theory. See ESI† for further details.
- T. Kawahara, Y. Kumaki, T. Kamada, T. Ishii and T. Okino, J. Org. Chem., 2009, 74, 6016 CrossRef CAS PubMed.
- Direction of analysis follows the numbering of (+)-danicalipin A (1).
- The 1,3-syn motif (A) has no clear preference between two conformers (tg− or g+t). In contrast, the 1,3-anti motif (B) favors one conformer (tt), see Fig. 1 and ref. 8b, 9c and d.
-
(a) D. K. Bedke, G. M. Shibuya, A. Pereira, W. H. Gerwick, T. H. Haines and C. D. Vanderwal, J. Am. Chem. Soc., 2009, 131, 7570 CrossRef CAS PubMed;
(b) T. Umezawa, M. Shibata, K. Kaneko, T. Okino and F. Matsuda, Org. Lett., 2011, 13, 904 CrossRef CAS PubMed;
(c) T. Yoshimitsu, R. Nakatani, A. Kobayashi and T. Tanaka, Org. Lett., 2011, 13, 908 CrossRef CAS PubMed;
(d) W.-j. Chung, J. S. Carlson and C. D. Vanderwal, J. Org. Chem., 2014, 79, 2226 CrossRef CAS PubMed;
(e) M. L. Landry, D. X. Hu, G. M. McKenna and N. Z. Burns, J. Am. Chem. Soc., 2016, 138, 5150 CrossRef CAS PubMed.
- M. Marigo, J. Franzen, T. B. Poulsen, W. Zhuang and K. A. Jørgensen, J. Am. Chem. Soc., 2005, 127, 6964 CrossRef CAS PubMed.
-
(a) Y. Kamada, Y. Kitamura, T. Tanaka and T. Yoshimitsu, Org. Biomol. Chem., 2013, 11, 1598 RSC;
(b) T. Yoshimitsu, N. Fukumoto and T. Tanaka, J. Org. Chem., 2009, 74, 696 CrossRef CAS PubMed.
- A. Hosomi, S. Kohra, Y. Tominaga, M. Ando and H. Sakurai, Chem. Pharm. Bull., 1987, 35, 3058 CrossRef CAS.
- H. C. Brown and P. K. Jadhav, J. Am. Chem. Soc., 1983, 105, 2092 CrossRef CAS.
- F. Munyemana, A.-M. Frisque-Hesbain, A. Devos and L. Ghosez, Tetrahedron Lett., 1989, 30, 3077 CrossRef CAS.
- Although olefin 13 (or 19) is theoretically a type I olefin, no evidence for “homodimer” derived from 13 (or 19) was found. We surmise that the electron-withdrawing effect of the chlorinated array gives it the reactivity of a type II olefin, see A. K. Chatterjee, T.-L. Choi, D. P. Sanders and R. H. Grubbs, J. Am. Chem. Soc., 2003, 125, 11360 CrossRef CAS PubMed.
- H. C. Kolb, M. S. VanNieuwenhze and K. B. Sharpless, Chem. Rev., 1994, 94, 2483 CrossRef CAS.
- P. R. Fleming and K. B. Sharpless, J. Org. Chem., 1991, 56, 2869 CrossRef CAS.
- A. Shemet, D. Sarlah and E. M. Carreira, Org. Lett., 2015, 17, 1878 CrossRef CAS PubMed.
- A compound possessing a similar C11,C13-syn dichloride was isolated by Vanderwal and co-workers as an undesired byproduct in an earlier synthesis but was not further investigated, see ref. 18a.
- Chlorosulfolipids 1, 2, and 3 showed no decomposition in solution (MeOH/DMSO) over several months.
- M. J. Lydon, K. D. Keeler and D. B. Thomas, J. Cell. Physiol., 1980, 102, 175 CrossRef CAS PubMed.
- During the experiment bacterial viability remained >90%. See ESI.†.
-
(a) S. Langsrud and G. Sundheim, J. Appl. Bacteriol., 1996, 81, 411 CrossRef CAS PubMed;
(b) B. L. Roth, M. Poot, S. T. Yue and P. Millard, J. Appl. Environ. Microbiol., 1997, 63, 2421 CAS;
(c) M. P. Bova, D. Tam, G. MeMahon and M. N. Mattson, Toxicol. Lett., 2005, 155, 41 CrossRef CAS PubMed.
-
1, 2, and 3 have similar toxicities towards Hepa 1-6 cells, see ESI.† The data in this table was collected at a concentration close to the EC50 values, yet a difference in permeability is observed. The results are consistent with the permeability enhancement (Sytox Green) stemming from the compounds and not toxicity (Hoechst 33342).
- DFT calculations were performed with Gaussian 09 for structures obtained from MacroModel on a M06-2X/6-311++G**/LANL2DZpd(Cl)/SMD(methanol)//M06-2X/6-31+G**/LANL2DZpd(Cl)/SMD(methanol) level of theory. See ESI† for further details.
- MacroModel did not predict structures which were in agreement with the limited solution-state NMR data. Therefore, manually generated structures were submitted to DFT calculations. See ESI.†.
- J. Beirão, L. Zilli, S. Vilella, E. Cabrita, C. Fernández-Díez, R. Schiavone and M. P. Herráez, J. Appl. Ichthyol., 2012, 28, 1017 CrossRef.
-
(a) M. Tavasli, D. O'Hagan, C. Pearson and M. C. Petty, Chem. Commun., 2002, 1226 RSC;
(b) G. Ewald and P. Sundin, Pharmacol. Toxicol., 1991, 69, 459 CrossRef.
-
(a) L. L. Chen, M. Pousada and T. H. Haines, J. Biol. Chem., 1976, 251, 1835 CAS;
(b) L. L. Chen and T. H. Haines, J. Biol. Chem., 1976, 251, 1828 CAS.
Footnotes |
† Electronic supplementary information (ESI) available. See DOI: 10.1039/c7sc03124f |
‡ These authors contributed equally to this work and share first authorship. |
|
This journal is © The Royal Society of Chemistry 2017 |