DOI:
10.1039/C6SC03303B
(Edge Article)
Chem. Sci., 2017,
8, 654-660
Chromium photocatalysis: accessing structural complements to Diels–Alder adducts with electron-deficient dienophiles†
Received
25th July 2016
, Accepted 11th September 2016
First published on 12th September 2016
Abstract
A chromium-catalyzed, visible light-activated net [4 + 2] cycloaddition between dienes and electron-deficient alkenes is described. Gathered evidence, via control experiments, isolated intermediates, and measured redox potentials, points to several converging reaction pathways that afford the cyclohexene adducts, including a photochemical [2 + 2] cycloaddition/vinylcyclobutane rearrangement cascade and a substrate excitation/oxidation sequence to a radical cation intermediate. Notably, the accompanying mechanistic stipulations result in a process that yields regioisomeric compounds from those generated by traditional Diels–Alder cycloadditions.
Introduction
In the past decade, the renaissance of photoredox catalysis has generated renewed interest in radical cation accelerated reactions initiated through photoinduced electron transfer (PET).1 Notable examples in the area of radical cation [2 + 2] and [4 + 2] cycloadditions2 have utilized metal photoredox catalysts containing Ru3 and Ir4 ions, as well as triarylpyrylium salts,5 as light-activated single-electron oxidants. In the interest of advancing sustainable chemical transformations,6 there has recently been a shift toward developing photocatalysts based on more earth-abundant metals, with particular achievements reported using Cu-7 and Fe-containing8 systems. Our groups have investigated strongly oxidizing poly-pyridyl and -phenanthrolinyl Cr photocatalysts.9 We reported that these light-activated Cr(III) complexes (E1/2 = +1.33–1.84 V vs. SCE10) are capable of promoting radical cation Diels–Alder cycloadditions of electron-rich alkenes,9b akin to the Ru-initiated examples from Yoon in 2011.11 Mechanistically, however, the Cr-photocatalyzed cycloaddition differs from the Ru version12 in that the reaction catalyzed by [Cr(Ph2phen)3](BF4)3 favored an oxygen-mediated catalytic cycle over radical chain propagation.9c This unique behavior encouraged us to investigate the synthetic utility of the Cr photocatalysts further.
The radical cation [4 + 2] cycloaddition using Ru or Cr with light was relatively constrained by the requirement of sufficiently oxidizable alkenes, a common stipulation in these reactions.13 Being mindful that photocatalysis has often provided an entry point for the construction of nonintuitive bonds via novel reaction manifolds,14 we wondered whether electron-poor dienophiles outside of the oxidizable realm could also yield cycloaddition products. Though electron-poor olefins would typically be expected to participate in [4 + 2] cycloadditions through more conventional, LUMO-lowering activation modes (e.g., thermal, Lewis acid), a photochemical strategy could broaden the range of Diels–Alder cycloadducts attainable, and perhaps offer orthogonal selectivity profiles (e.g., regio-, diastereo-). Herein, we report the Cr-photocatalyzed cycloaddition of electron-poor olefins with dienes (Fig. 1). Experiments implicate multiple operative mechanistic pathways that converge to form the same cycloadducts. Importantly, the described transformation results in Diels–Alder products of reversed regioselectivity, yielding “meta” adducts as opposed to the “ortho” and “para” adducts generated under conventional activation.
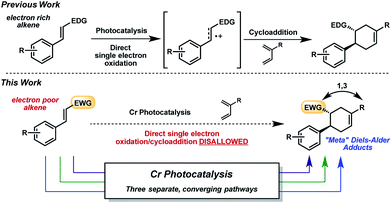 |
| Fig. 1 Photocatalyzed [4 + 2] cycloadditions with electron deficient alkenes. | |
Results and discussion
Reaction development
The reactivity of 4-methoxychalcone is illustrative (1, Fig. 2). In intermolecular Diels–Alder cycloadditions using conventional activation, enones of this type routinely require rather forcing conditions and yield predominantly adduct 4.15 Based on our previous efforts, we reasoned that the cycloaddition of 4-methoxychalcone through a photocatalytic oxidation pathway would be out of the question, as the oxidation potential of enone 1 (+2.00 V, in CH3NO2) is too positive to be oxidized by the [Cr(Ph2phen)3]3+ catalyst (
in CH3NO2) in the pathway as described for the electron-rich dienophiles.9b,16 Thus, under Cr-photocatalysis conditions, no cycloaddition between 4-methoxychalcone (1) and isoprene (2) should occur.
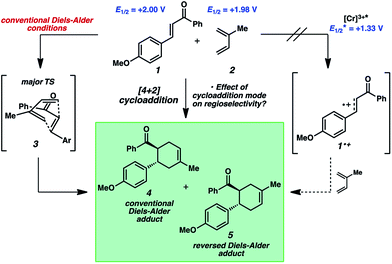 |
| Fig. 2 Cycloaddition reactivity of 4-methoxychalcone. | |
Surprisingly, however, when enone 1 was exposed to [Cr(Ph2phen)3](BF4)3 in the presence of isoprene (2) and irradiation with a 23 W compact fluorescent light bulb, cycloadduct 5 was formed in 85% yield (80% isolated yield, Scheme 1). Remarkably, the regioselectivity of this cycloaddition was 13
:
1 favoring the reversed Diels–Alder adduct (5). We found that increased catalyst loading and equivalents of diene did not increase the yield. Near-UV (NUV), blue LEDs, and sunlight also effected this transformation, but optimal results were achieved with the 23 W CFL source. Other photoredox catalysts were less effective (vide infra). A control experiment confirmed that no reaction occurred without light. Notably, with light but in the absence of catalyst, vinylcyclobutane 6 was formed in 20% yield, presumably via photochemical [2 + 2] cycloaddition between enone 1 and isoprene,17 but no [4 + 2] products were detected. Lastly, performing the reaction in the absence of air significantly decelerated the formation of cyclohexene 5, while vinylcyclobutane 6 also formed. We had previously found oxygen to be important for Cr-mediated photocatalysis with electron-rich substrates (vide infra).9b,c,18
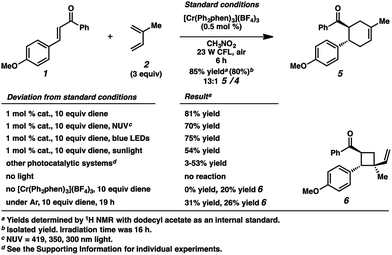 |
| Scheme 1 Selected optimization experiments. | |
Substrate scope
Encouraged by the success of this reaction, we set out to test the limits of these cycloadditions by examining other electron-poor olefins (Scheme 2). We were delighted to find this process was successful for a range of cycloaddition partners. Several differentially substituted chalcone derivatives were found to be viable substrates for this transformation (5, 7–9). Other α,β-unsaturated carbonyl compounds reacted in moderate to high yields (10–13). A nitroolefin was also a competent substrate, forming cyclohexene 14 in 74% yield. Consistent with the 4-methoxychalcone case, the transformations with isoprene all proceeded with high levels of regioselectivity (8
:
1 to 19
:
1). Symmetrical 2,3-dimethyl-1,3-butadiene could be used with several of these dienophiles, affording cyclohexenes 15–17 in good yields. Regioselectivity considerations are not applicable for this diene, but it is still remarkable that this method circumvents the forcing conditions generally required to obtain these adducts.19 Differentially substituted dienes were also proficient in this reaction. The cycloaddition conditions were tolerant of a variety of functional groups on the diene (18–21, 24, 26). In addition, terminally substituted dienes gave cyclohexenes 22–26 in high yields. Somewhat lower regioselectivity ratios were observed, but stereoselectivity was excellent in these processes, as the major constitutional isomer was observed as a single diastereomer.20 Lastly, enones containing different electron-rich aryl groups at the β-position also participated in the reaction (27, 28), although we note here the scope was more limited.21 For example, an enone with an electron-neutral arene was not productive in this transformation (29).
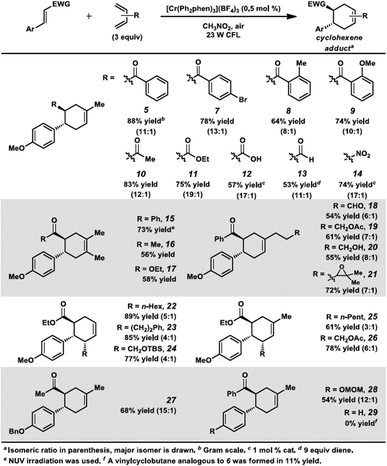 |
| Scheme 2 The Cr-photocatalyzed [4 + 2] cycloaddition between dienes and electron-deficient alkenes-scope. | |
Mechanistic pathways
We have found in our initial mechanistic studies the potentially operative pathways deviate significantly from the direct oxidation route. As mentioned previously, accounting for reduction potentials it is unlikely that the photoexcited Cr catalyst (Cr3+*/2+E1/2 = +1.33 V in CH3NO2) could be directly oxidizing 4-methoxychalcone (1+/0, +2.00 V) or isoprene (2+/0, +1.98 V (ref. 22)) to initiate the cycloaddition.23 Furthermore, all attempts to catalyze the cycloaddition using photocatalysts with more positive excited state reduction potentials, such as [Ru(bpz)3]2+, triphenylpyrilium, Mes-Acr, or a variety of cyanoarenes and chloranil,24 provided lower yields than [Cr(Ph2phen)3](BF4)3 (see the ESI†), indicating that this transformation is likely not proceeding via simple direct oxidation of the electron deficient alkene. An energy transfer pathway could also be ruled out since the long-lived excited state of
(38 kcal mol−1) is considerably lower than the transfer energies of either the 4-methoxychalcone (1) or isoprene (the triplet excited state energies are both ∼60 kcal mol−1).25
We thus considered other mechanistic pathways (Fig. 3). One possibility is that the reaction is proceeding through intermediate vinylcyclobutane 6 (Pathway A), which we observed when the reaction was performed without catalyst.26 Indeed, when vinylcyclobutane 6 (E1/2 = +1.68 V in CH3NO2) was exposed to the Cr conditions, product 5 was formed in 67% yield as a single isomer (Scheme 3).27 When this rearrangement was performed in the presence of excess diene 31, only the rearrangement product was observed and not the cross-adduct between 4-methoxychalcone and the added diene, confirming that cyclohexene 5 is forming through direct rearrangement of vinylcyclobutane 6 and not through cycloreversion/recombination.28,29 Thus, we believe that a cascade pathway involving photochemical [2 + 2] cycloaddition (1, λmax = 340 nm) followed by single-electron oxidative vinylcyclobutane rearrangement30 is viable and occurring. We note, however, that cyclobutane 6 was formed in only 20% yield without catalyst, while 85% yield of cyclohexene 5 was formed with catalyst in the same duration, suggesting that this [2 + 2]/rearrangement may not be the only mechanistic pathway.
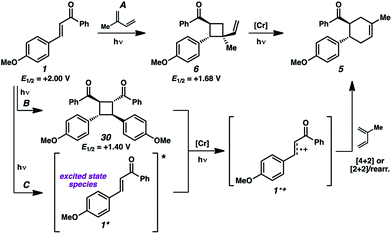 |
| Fig. 3 Possible mechanistic pathways. | |
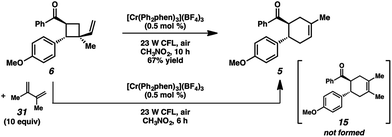 |
| Scheme 3 Rearrangement of vinylcyclobutane 6. | |
A different route, Pathway B offers a means of accessing a reactive enone radical cation (1˙+, Fig. 3). If in situ dimerization of enone 1 occurs,31 the resulting dimer (30) has a significantly lower reduction potential (E1/2 = +1.40 V, in CH3NO2) than the starting enone. Oxidation could then induce cycloreversion to the radical cation, and interception of this putative radical cation with the diene could afford the cycloaddition product (via direct [4 + 2] and/or [2 + 2]/rearrangement).32 Control experiments suggest this pathway is a minor contributor at most. First, enone dimer 30 was found to form in only trace amounts under the standard Cr conditions in the absence of diene. Furthermore, independently synthesized enone dimer 30 subjected to irradiation affords retrocyclobutanation with and without catalyst, but in low yields (Scheme 4). When dimer 30 was exposed to the cycloaddition conditions in place of enone 1, cyclohexene 5 formed in 20% yield, at least implicating the feasibility of this pathway. Notwithstanding, the low production of dimer 30 and the relatively sluggish cycloreversion suggests a lesser role in the formation of cyclohexene 5.
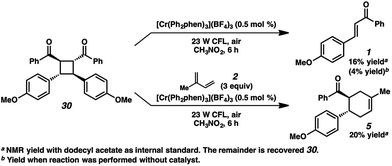 |
| Scheme 4 Cycloreversion of enone dimer 30. | |
An alternative pathway for the generation of 1˙+ recognizes that the photoexcited enone (1*) will be easier to oxidize than its ground state form (Fig. 3, Pathway C). Select reports indicate that the excitation of chalcone derivatives and related α,β-unsaturated carbonyl species lead to excimers33 and/or charge-transfer complexes,34 which enable single-electron oxidation.35 The oxidation of enone 1* in this manner would generate radical cation 1˙+, which would proceed to product 5 as described earlier. Here, we note that enone 1 shows weak emission at 443 nm when excited at 340 nm in acetonitrile, from which we estimate an excited state reduction potential (1˙+/0*) of −0.80 V, which suggests thermodynamic competency for oxidation of 1* by the photoexcited Cr catalyst. The triplet excited state lifetime of enone 1 was reported to be 23 and 29 ns in heptane and methanol, respectively,25a suggesting this is well within the range of feasibility for oxidation by the long-lived excited state of the Cr catalyst.
Bauld and coworkers have discussed the inhibitory effect of trans-anethole on competing radical cation cycloadditions and vinylcyclobutane rearrangements due to its highly oxidizable nature (E1/2 = +1.35 V (ref. 9c)).22,36 Thus, if the cycloaddition of enone 1 and isoprene (2) is proceeding through a radical cation (e.g., 1˙+), we would expect the addition of trans-anethole to considerably impede the formation of cycloadduct 5. In accordance with this hypothesis, in a competition experiment using a 1
:
1 mixture of trans-anethole (32) and enone 1 with excess isoprene, the cycloaddition with enone 1 proceeded only after the majority of 32 was consumed (Scheme 5). The inhibitory effect of alkene 32 may implicate the intermediacy of the enone radical cation (1˙+) in the overall process.
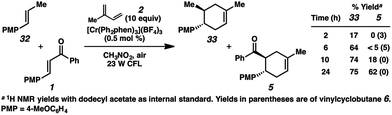 |
| Scheme 5 Competition experiment with trans-anethole. | |
In an effort to probe the termination step(s) concerning all possible pathways, quantum yield experiments were performed. Since compounds 1, 6, and 30 can all be invoked as starting materials or intermediates in the mechanism, each was tested for their photochemical efficiency. At 350 nm excitation, maximum quantum yield values for species 1, 6, and 30 were Φ = 0.013, 0.45, and 0.013, respectively. Compounds 1, 6, and 30 do absorb light at 350 nm (as does nitromethane), and their equilibria in solution complicate the calculation of an accurate quenching factor. Therefore compound 6, which showed the largest quantum yield, was excited at 400 nm in the presence of [Cr(Ph2phen)3]3+ in CD3NO2 where the maximum quantum yield was Φ = 0.93. Since only [Cr(Ph2phen)3]3+ absorbs light at this wavelength, the quenching factor is assumed to be ∼1, giving a chain length value of <1. These data corroborate the lack of a predominant radical chain mechanism12 in the reaction manifold. Moreover, when a deoxygenated sample containing 6 and [Cr(Ph2phen)3]3+ was irradiated at 400 nm in nitromethane, the quantum yield decreased to a maximum value of Φ = 0.21, further implicating this reactivity as photocatalytic instead of photoinitiated, akin to our previous report.9c
The presence of oxygen in this transformation also deserves mention. In our earlier study, we had discussed the roles of O2 in the cycloaddition using electron-rich alkenes. O2 was essential, and the absence of it shut down catalysis altogether. Singlet oxygen was formed in these cycloadditions via a quench of the long-lived Cr(III) excited state. The singlet oxygen is then reduced to superoxide by Cr(II), and the superoxide then reduces the cycloadduct radical cation. In this specific reaction with electron-deficient alkenes, the effect of O2 is still beneficial to the overall reaction progress, but not nearly to the same extent as the earlier transformation (i.e., reaction rates are slower, but the cyclohexene product is still formed via catalytic turnover). We believe singlet oxygen is formed in these cycloadditions as well. In the analysis of the reaction and the substrate scope, in several cases we noted the formation of a minor byproduct (average <5% yield). We determined this byproduct to be an allylic hydroperoxide, presumably arising from the oxidation of the cyclohexene product by 1O2.37 It is possible that we may be amplifying or diminishing specific reaction pathways in the presence of O2; future studies may elucidate its multifaceted effects.
From the evidence amassed thus far we conclude that this Cr-photocatalyzed cycloaddition using an electron deficient dienophile can occur through several reaction pathways involving photochemical and radical cation processes, all outside of the direct electron transfer or energy transfer pathways. Coincidentally, the operative pathways all converge to the same cyclohexene adducts. Further mechanistic studies are underway.38
Diels–Alder regioselectivity analysis
A hallmark characteristic of the Diels–Alder reaction is its highly predictive regioselective outcomes. An electron-withdrawing group on the dienophile and an electron-donating group on the diene will impact the coefficients of the FMOs so as to dictate the overall regioselectivity of the cycloaddition (i.e., the “ortho–para rule”). Efforts to reverse this natural regioselectivity of Diels–Alder cycloadditions have been reported, but only a handful of strategies have been successful.39,40 These include the incorporation of electronically steering substituents that can be subsequently removed,41 and catalyzed vs. thermal/noncatalyzed cycloadditions that adjust the molecular orbital coefficients of the reactants (e.g., selective coordination of a sterically unhindered carbonyl).42Table 1 depicts an alternative strategy to achieve this unnatural regioselectivity. Example dienes and β-(para-methoxyphenyl)nitroethylene were combined using both previously described LiClO4/CH3NO2 conditions43 and our chromium photocatalyzed conditions. As can be seen, these transformations each proceed to afford complementary regiochemistries, where the photocatalyzed reactions occur with high selectivity for the unconventional Diels–Alder adducts. This unconventional regioselectivity tracks consistently with all of the selectivities observed in the cases in Scheme 2. These transformations represent a novel, conditions-based approach to invert the Diels–Alder regioselectivity with reactive diene/dienophile partners.
Table 1 Reversed regioselectivity under Cr-photocatalysis conditions
Our hypothesis for the observed regioselectivity is illustrated in Fig. 4, using chalcone 1 and isoprene as an example. Notably, whether the cycloaddition is proceeding through a photochemical “head-to-tail” [2 + 2] cycloaddition44 followed by a radical cation vinylcyclobutane rearrangement, or an enone radical cation pathway, the net reversed Diels–Alder regioselectivity should be favored. With isoprene (2), the methyl substituent allows selective generation of the more stable diradical or radical cation intermediate. Presumably, the aryl group also stabilizes this intermediate species to some extent.
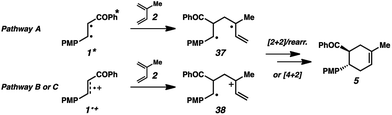 |
| Fig. 4 General regiochemical explanation. | |
Conclusions
The deliberate investigations of photocatalytic manifolds with various components have unlocked new transformations that possess tremendous potential for the chemistry community. We have shown that that the Cr-catalyzed [4 + 2] cycloaddition of dienes with electron-poor alkenes (i.e., outside of the oxidizable realm) can yield cyclohexene adducts, and, perhaps most pertinently, with opposite regioselectivities to those provided via the traditional Diels–Alder cycloaddition. In as much as the previous Cr-catalyzed [4 + 2] cycloaddition is a chemoselective complement to the Diels–Alder reaction, this example represents a regioselective complement to the transformation. This process is optimally effective using Cr photocatalysis. Mechanistic investigations elucidating the unique impact of Cr, and efforts to expand this chemistry, will be reported in due course.
Acknowledgements
This research was carried out by the Catalysis Collaboratory for Light-activated Earth Abundant Reagents (C-CLEAR), which is supported by the NSF and EPA through the Network for Sustainable Molecular Design and Synthesis program (NSF-CHE-1339674). We acknowledge collaborators Niels Damrauer and Anthony Rappé and their groups for enriching discussions. Sang Lee is acknowledged for experimental assistance.
Notes and references
- For a recent discussion of this topic, see, A. Studer and D. P. Curran, Angew. Chem., Int. Ed., 2016, 55, 58–102 CrossRef CAS PubMed.
-
(a) F. Müller and J. Mattay, Chem. Rev., 1993, 93, 99–117 CrossRef;
(b) T. P. Yoon, ACS Catal., 2013, 3, 895–902 CrossRef CAS PubMed;
(c) Y. Liu, R. Song and J. Li, Sci. China: Chem., 2016, 59, 161–170 CrossRef CAS.
-
(a) M. A. Ischay, Z. Lu and T. P. Yoon, J. Am. Chem. Soc., 2010, 132, 8572–8574 CrossRef CAS PubMed;
(b) M. A. Ischay, M. S. Ament and T. P. Yoon, Chem. Sci., 2012, 3, 2807–2811 RSC;
(c) S. Lin, C. E. Padilla, M. A. Ischay and T. P. Yoon, Tetrahedron Lett., 2012, 53, 3073–3076 CrossRef CAS PubMed.
-
(a) Z. Ma, X. Wang, X. Wang, R. A. Rodriguez, C. E. Moore, S. Gao, X. Tan, Y. Ma, A. L. Rheingold, P. S. Baran and C. Chen, Science, 2014, 346, 219–224 CrossRef CAS PubMed;
(b) X. Wang and C. Chen, Tetrahedron, 2015, 71, 3690–3693 CrossRef CAS PubMed.
- M. Riener and D. A. Nicewicz, Chem. Sci., 2013, 4, 2625–2629 RSC.
- T. P. Yoon, M. A. Ischay and J. Du, Nat. Chem., 2010, 2, 527–532 CrossRef CAS PubMed.
- For select examples, see:
(a) S. E. Creutz, K. J. Lotito, G. C. Fu and J. C. Peters, Science, 2012, 338, 647–651 CrossRef CAS PubMed;
(b) M. Pirtsch, S. Paria, T. Matsuno, H. Isobe and O. Reiser, Chem.–Eur. J., 2012, 18, 7336–7340 CrossRef CAS PubMed;
(c) M. Majek and A. Jacobi von Wangelin, Angew. Chem., Int. Ed., 2013, 52, 5919–5921 CrossRef CAS PubMed;
(d) A. C. Hernandez-Perez and S. K. Collins, Angew. Chem., Int. Ed., 2013, 52, 12696–12700 CrossRef CAS PubMed;
(e) S. Paria and O. Reiser, ChemCatChem, 2014, 6, 2477–2483 CrossRef CAS;
(f) Q. M. Kainz, C. D. Matier, A. Bartoszewicz, S. L. Zultanski, J. C. Peters and G. C. Fu, Science, 2016, 351, 681–684 CrossRef CAS PubMed.
-
(a) A. Gualandi, M. Marchini, L. Mengozzi, M. Natali, M. Lucarini, P. Ceroni and P. G. Cozzi, ACS Catal., 2015, 5, 5927–5931 CrossRef CAS;
(b) J. Zhang, D. Campolo, F. Dumur, P. Xiao, J. P. Fouassier, D. Gigmes and J. Lalevée, J. Polym. Sci., Part A: Polym. Chem., 2015, 53, 42–49 CrossRef CAS;
(c) J. Zhang, D. Campolo, F. Dumur, P. Xiao, J. P. Fouassier, D. Gigmes and J. Lalevée, J. Polym. Sci., Part A: Polym. Chem., 2016, 54, 2247–2253 CrossRef CAS.
-
(a) A. M. McDaniel, H.-W. Tseng, N. H. Damrauer and M. P. Shores, Inorg. Chem., 2010, 49, 7981–7991 CrossRef CAS PubMed;
(b) S. M. Stevenson, M. P. Shores and E. M. Ferreira, Angew. Chem., Int. Ed., 2015, 54, 6506–6510 CrossRef CAS PubMed;
(c) R. F. Higgins, S. M. Fatur, S. G. Shepard, S. M. Stevenson, D. J. Boston, E. M. Ferreira, N. H. Damrauer, A. K. Rappé and M. P. Shores, J. Am. Chem. Soc., 2016, 138, 5451–5464 CrossRef CAS PubMed.
- All reduction/oxidation potentials are in V vs. SCE in CH3CN unless otherwise noted. Those measured in CH3NO2 are also vs. SCE.
- S. Lin, M. A. Ischay, C. G. Fry and T. P. Yoon, J. Am. Chem. Soc., 2011, 133, 19350–19353 CrossRef CAS PubMed.
- M. A. Cismesia and T. P. Yoon, Chem. Sci., 2015, 6, 5426–5434 RSC.
- N. L. Bauld, D. J. Bellville, B. Harirchian, K. T. Lorenz, R. A. Pabon, D. W. Reynolds, D. D. Wirth, H.-S. Chiou and B. K. Marsh, Acc. Chem. Res., 1987, 20, 371–378 CrossRef CAS.
-
(a) C. K. Prier, D. A. Rankic and D. W. C. MacMillan, Chem. Rev., 2013, 113, 5322–5363 CrossRef CAS PubMed;
(b) J. Xuan and W.-J. Xiao, Angew. Chem., Int. Ed., 2012, 51, 6828–6838 CrossRef CAS PubMed;
(c) J. M. R. Narayanam and C. R. J. Stephenson, Chem. Soc. Rev., 2011, 40, 102–113 RSC;
(d) R. A. Angnes, Z. Li, C. R. D. Correia and G. B. Hammond, Org. Biomol. Chem., 2015, 13, 9152–9167 RSC.
- For a list of select examples, see the ESI.†.
- We note here that in our experience the reduction potentials can be sensitive to the conditions of measurement (solvent, additives, etc.) and thus should only be used as a general guide. Reduction potentials cited from the literature are compiled in the ESI.†.
- Intermolecular photochemical [2 + 2] cycloadditions of acyclic chalcone derivatives and related α,β-unsaturated carbonyls are rare due to the propensity of these species for cis/trans-isomerization upon photoexcitation. See:
(a) S. Poplata, A. Tröster, Y.-Q. Zou and T. Bach, Chem. Rev., 2016 DOI:10.1021/acs.chemrev.5b00723 , in press;
(b)
P. Margaretha, in Molecular and Supramolecular Photochemistry, ed. A. G. Griesbeck and J. Mattay, Marcel Dekker, New York, 2005, vol. 12, ch. 8 Search PubMed;
(c) A. K. F. Albertson and J.-P. Lumb, Angew. Chem., Int. Ed., 2015, 54, 2204–2208 CrossRef CAS PubMed.
- When the reaction was performed where O2 was present, we also observed in very minor quantities (average <5% yield) the formation of an allylic hydroperoxide byproduct.
- In sample cases (e.g., 16, 17), this method provided noticeably enhanced dienophile reactivity over conventional activation techniques. See the ESI for an analysis.†.
- Relative stereochemistry confirmed by NMR analysis.
- In the interest of synthetic utility, the PMP group can be oxidatively cleaved. See ESI.†.
- N. L. Bauld, Tetrahedron, 1989, 45, 5307–5363 CrossRef CAS.
-
(a) The reduction potential value is measured based on the emission from the 2E state. In an earlier report on the [Cr(Ph2Phen)3]3+ complex (ref. 9a), there was an extra emissive feature which was assigned to a 2T1 state that has a much shorter lifetime. This emission corresponds to a Cr3+*/2+E1/2 = +1.42 V in CH3NO2, still well below the reduction potentials of the substrates investigated here. To date, there is no observed evidence of the higher energy state (i.e., 4T2) using picosecond spectroscopy, so we believe this 4T2 state is likely insignificant in this photocatalytic system;
(b) For a related reference discussing the 4T2 state in Cr(acac)3-type complexes, see: J. N. Schrauben, K. L. Dillman, W. F. Beck and J. K. McCusker, Chem. Sci., 2010, 1, 405–410 RSC;
(c) For a recent example of a Cr(III) complex that takes advantage of strong-field ligands to establish a large energy gap between the 2E/2T1 and the 4T2 states, see: S. Otto, M. Grabolle, C. Förster, C. Kreitner, U. Resch-Genger and K. Heinze, Angew. Chem., Int. Ed., 2015, 54, 11572–11576 CrossRef CAS PubMed.
- In other examples, triphenylpyrilium, 1,4-dicyanobenzene, and 9,10-dicyanoanthracene have been observed to oxidize chalcone derivatives for intramolecular nucleophilic attack. See:
(a) S. Kar and S. Lahiri, Indian J. Chem., 2001, 40B, 1121–1124 CAS;
(b) G. Pandey, A. Krishna and G. Kumaraswamy, Tetrahedron Lett., 1987, 28, 4615–4616 CrossRef CAS;
(c) M. J. Climent, H. García, S. Iborra, M. A. Miranda and J. Primo, Heterocycles, 1989, 29, 115–121 CrossRef CAS.
-
(a) R. A. Caldwell and M. Singh, J. Am. Chem. Soc., 1983, 105, 5139–5140 CrossRef CAS;
(b) R. A. Caldwell, J. Am. Chem. Soc., 1970, 92, 3229–3230 CrossRef CAS;
(c) A static emission spectrum of 4-methoxychalcone is provided in the ESI.†.
- Vinylcyclobutane 6 was also detected in the crude NMR spectra of incomplete reactions.
- The allylic hydroperoxide byproduct (ref. 18) was formed more prominently in this specific experiment, leading to the diminshed yield as compared to the full cycloaddition. No rearrangement occurred in the absence of light; trace cyclohexene 5 was formed in the absence of catalyst.
- For examples of cyclobutane cycloreversion, see:
(a) F. D. Lewis and M. Kojima, J. Am. Chem. Soc., 1988, 110, 8664–8670 CrossRef CAS;
(b)
Ref. 3b
;
(c)
Ref. 5
.
- The reverse trapping experiments were also performed. See ESI for details.†.
-
(a) D. W. Reynolds, B. Harirchan, H.-S. Chiou, B. K. Marsh and N. L. Bauld, J. Phys. Org. Chem., 1989, 2, 57–88 CrossRef CAS;
(b) T. Kim, R. J. Pye and N. L. Bauld, J. Am. Chem. Soc., 1990, 112, 6285–6290 CrossRef CAS.
-
(a) F. Toda, K. Tanaka and M. Kato, J. Chem. Soc., Perkin Trans. 1, 1998, 1315–1318 RSC;
(b) M. D'Auria and A. Vantaggi, Tetrahedron, 1992, 48, 2523–2528 CrossRef;
(c)
Ref. 24a
.
- The bifurcation of [4 + 2] vs. [2 + 2]/VCBR in electron transfer processes has been the subject of intense investigation. See the ESI for a list of select studies.†.
- S. Chatterjee, S. Kar, S. Lahiri and S. Basu, Spectrochim. Acta, Part A, 2004, 60, 1713–1718 CrossRef PubMed.
-
(a) P. Wang and S. Wu, J. Photochem. Photobiol., A, 1994, 77, 127–131 CrossRef CAS;
(b) Y. Wang, J. Phys. Chem., 1985, 89, 3799–3805 CrossRef CAS;
(c) R. J. DeVoe, M. R. V. Sahyun and E. Schmidt, Can. J. Chem., 1989, 67, 1565–1575 CrossRef CAS;
(d) S. Kar, S. Aich, S. Basu and S. Lahiri, Res. Chem. Intermed., 1999, 25, 903–913 CrossRef CAS.
-
(a) S. Kar and S. Lahiri, J. Chem. Soc., Chem. Commun., 1995, 957–958 RSC;
(b) D. P. Kranz, A. G. Griesbeck, R. Alle, R. Perez-Ruiz, J. M. Neudörfl, K. Meerholz and H.-G. Schmalz, Angew. Chem., Int. Ed., 2012, 51, 6000–6004 CrossRef CAS PubMed.
- W. Yueh and N. L. Bauld, J. Phys. Org. Chem., 1996, 9, 529–538 CrossRef CAS.
- For a review discussing allylic oxidation by 1O2, see: H. H. Wasserman and J. L. Ives, Tetrahedron, 1981, 37, 1825–1852 CrossRef CAS.
- In preliminary experiments, we have seen a nonlinear dependence of the light intensity on the overall rate of reactivity. This observation is also consistent with multiple two-photon reaction pathways.
- F. Peng, R. E. Grote, R. M. Wilson and S. J. Danishefsky, Proc. Natl. Acad. Sci. U. S. A., 2013, 110, 10904–10909 CrossRef CAS PubMed.
- The Yoon Ru-catalyzed [4 + 2] cycloaddition using electron-rich alkenes in ref. 11 also relates how the photocatalytic strategy can be used to access regioisomeric Diels–Alder adducts.
-
(a) S. Danishefsky, M. P. Prisbylla and S. Hiner, J. Am. Chem. Soc., 1978, 100, 2918–2920 CrossRef CAS;
(b) N. Ono, H. Miyake and A. Kaji, J. Chem. Soc., Chem. Commun., 1982, 33–34 RSC.
-
(a) B. M. Trost, J. Ippen and W. C. Vladuchick, J. Am. Chem. Soc., 1977, 99, 8116–8118 CrossRef CAS;
(b) Z. Stojanac, R. A. Dickinson, N. Stojanac, R. J. Woznow and Z. Valenta, Can. J. Chem., 1975, 53, 616–618 CrossRef CAS;
(c) J. S. Tou and W. Reusch, J. Org. Chem., 1980, 45, 5012–5014 CrossRef CAS;
(d) P. V. Alston, M. D. Gordon, R. M. Ottenbrite and T. Cohen, J. Org. Chem., 1983, 48, 5051–5054 CrossRef CAS.
- M. Ayerbe and F. P. Cossío, Tetrahedron Lett., 1995, 36, 4447–4450 CrossRef CAS.
- E. J. Corey, J. D. Bass, R. LeMahieu and R. B. Mitra, J. Am. Chem. Soc., 1964, 86, 5570–5583 CrossRef CAS.
Footnote |
† Electronic supplementary information (ESI) available: Experimental procedures, characterization data, electrochemistry data, UV/visible data, NMR spectra. See DOI: 10.1039/c6sc03303b |
|
This journal is © The Royal Society of Chemistry 2017 |
Click here to see how this site uses Cookies. View our privacy policy here.