DOI:
10.1039/C6SC02285E
(Edge Article)
Chem. Sci., 2016,
7, 7007-7012
Converting disulfide bridges in native peptides to stable methylene thioacetals†
Received
23rd May 2016
, Accepted 24th July 2016
First published on 28th July 2016
Abstract
Disulfide bridges play a crucial role in defining and rigidifying the three-dimensional structure of peptides. However, disulfides are inherently unstable in reducing environments. Consequently, the development of strategies aiming to circumvent these deficiencies – ideally with little structural disturbance – are highly sought after. Herein, we report a simple protocol converting the disulfide bond of peptides into highly stable methylene thioacetal. The transformation occurs under mild, biocompatible conditions, enabling the conversion of unprotected native peptides into analogues with enhanced stability. The developed protocol is applicable to a range of peptides and selective in the presence of a multitude of potentially reactive functional groups. The thioacetal modification annihilates the reductive lability and increases the serum, pH and temperature stability of the important peptide hormone oxytocin. Moreover, it is shown that the biological activities for oxytocin are retained.
Introduction
Peptides have recently been enjoying a renewed interest in their application as therapeutic agents.1 They provide a large chemical space with a diverse array of molecular frameworks for the development of novel therapeutics for a plethora of biomedical applications. However, peptides exhibit inherent drawbacks as drug candidates, due to their conformational flexibility and low stability.1a,2 Several strategies have evolved to address these unfavourable pharmacokinetics by increasing stability through local or global constraints. This involves the incorporation of non-natural amino acids3 or amide bond surrogates,2,4 as well as various cyclisation modes,5 side chain functionalisations6 and other modifications.7,8 These approaches stabilise the secondary and tertiary structure of the peptide by constraining it to a more defined geometry. This increases protease resistance as the vulnerable bonds are less easily accessible.
Disulfide bridges play a crucial role in defining and rigidifying the three-dimensional structure of natural peptides and proteins. However, disulfides are inherently unstable in reducing environments and towards nucleophiles. Reduction or scrambling of the disulfide bonds is caused by transhydrogenases, thiol oxidoreductases, disulfide isomerases and other thiol containing agents, such as serum albumin or glutathione.9 All of them possess the ability to decrease the lifespan of therapeutic disulfide containing agents. Therefore, disulfide bond engineering emerged as an important strategy to improve the metabolic stability of disulfide-containing peptides, whilst maintaining their biological activity. For instance, replacements of the disulfide group with a lactam,10 thioether,11 selenium12 or dicarba13 analogues have been reported.5a Many of these methods require significant modification of the synthetic building blocks, at the initiation of the synthesis towards the final peptide product, which can be problematic.11b,14 In addition to the synthetic efforts of a de novo approach, these modifications may introduce steric distortions that can negatively impact activity and selectivity.15
Concept
Hence, we envisioned a disulfide stapling procedure performed under mild, biocompatible reaction conditions (aqueous media, low to ambient temperature and mild pH) that can be used directly with unprotected native peptides. A key aim of our strategy was to insert a minimal linker into the disulfide bond and maintain the structural integrity necessary for the biological activity of the peptides. We proposed to achieve this by the insertion of a methylene group in between the two sulfur atoms, giving a thioacetal functional group (Scheme 1). Thioacetals are utilised in organic synthesis as a rugged protecting group for carbonyl groups. Chemically, they are inert towards nucleophiles and most reductive conditions.
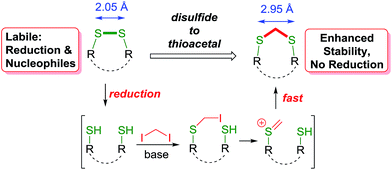 |
| Scheme 1 Thioacetal linkages by methylene insertion into disulfides for enhanced stability. | |
The thioacetal group has been sporadically previously used to functionally rebridge disulfide bonds within peptide structures.16 However, these reports suffer from significant shortcomings by using either strongly basic conditions, employing the linker reagent as the solvent or organic and non-biocompatible solvents. Furthermore, these methods were not applied to unprotected native peptides. Moreover, this method would also allow the convenient introduction of radiolabeled methylene groups for tracing or imaging purposes. In line with our aims for minimising structural disturbance with the disulfide modification, CH2X2 (X = Cl, Br or I) was selected as the smallest possible electrophilic linker. This is complementary to the highly reactive multi-bromo benzylic linkers employed to invoke the cyclisation of prior non-cyclised peptide segments due to the sterically larger size of this linker.17 For our approach, one end of the reduced disulfide bridge will be alkylated with the electrophilic reagent. A facile ionisation provides a thiocarbenium ion18 of superior electrophilicity, leading to a rapid cyclisation to the desired methylene thioacetal before any additional reaction with another linker molecule would occur.
Results and discussion
Investigation into a one-pot biocompatible disulfide bond modification
The initial assessment and optimisation of the disulfide to thioacetal conversion, was conducted on N,N′-bis-Boc L-cystine dimethyl ester as a simple model substrate (see ESI†). A completely chemoselective thiol alkylation of the sulfhydryl group forming the desired methylene thioacetal was observed, with no detectable side products. Potentially interfering amino acids were added to the reaction mixture and were innocent bystanders. Diiodomethane was the most efficient linker reagent, although dibromomethane and even dichloromethane reacted. Despite its higher reactivity, it did not result in any undesired side products. A mild base is required for the reaction to proceed as thiols themselves do not react. The reaction performs well at ambient temperature. The addition of an organic co-solvent helps solubilise the linker reagent and THF was favoured due to its miscibility with water and low boiling point. The protocol was completed by a prior disulfide bond reduction using water soluble tris-(2-carboxyethyl)phosphine (TCEP) (Scheme 2).19 Pleasingly, CH2I2 was found to be compatible with TCEP under the reaction conditions, even though it had been reported to impede bioconjugations with maleimides and α-haloacyl groups.20 For instance, after the reduction of glutathione disulfide (GSSG) to glutathione (GSH), addition of diiodomethane and triethylamine provided the thioacetal modified version GSCSG in 53% yield.
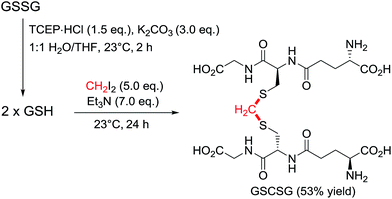 |
| Scheme 2 Performing the mild one-pot thioacetal transformation on glutathione. | |
Application of the thioacetal modification to a scope of bioactive peptides
With this simple and effective protocol we aimed to apply this method to bioactive peptides and drugs, containing a plethora of proteinogenic amino acids, to rigorously test the compatibility of our approach (Scheme 3). The selected cyclic peptides have a broad variety of relevant biological activities, which would allow a comparative study of the native parent peptide to the modified analogue. Firstly, we focused on cyclic octapeptide octreotide as more complex prototype substrate. Octreotide is pharmacologically related to somatostatin and is a potent growth hormone inhibitor.21 It is an important drug marketed for the treatment of a variety of hormone producing tumors.22 Application of the one-pot methylene insertion protocol provided the desired modified SCS-Octreotide (1) in a good yield of 78%. Importantly, the transformation remained efficient under dilute conditions (5 mM) as well as a largely reduced amount of the organic co-solvent (5
:
1 ratio water/THF). Exposure of octreotide to CH2I2 and NEt3 without prior disulfide reduction led to full recovery. No chemical modifications occurred at any other sites, highlighting the high chemoselectivity of the linker protocol. The pharmacologically related natural tetradecapeptide hormone Somatostatin (4),23 performed efficiently under the one-pot transformation to provide the thioacetal bridged analogue in 72% yield. Next, neurohypophysial nonapeptide hormones oxytocin (OXT)24 and related Arginine Vasopressin (AVP),25 were targeted. Oxytocin is marketed as a drug to instigate uterine contractions as well to prevent post-partum hemorrhage. Vasopressin constricts the blood vessels to increase arterial blood pressure and regulates the body's retention of water. Both OXT and AVP smoothly underwent the cut-and-sew methylene insertion procedure, affording the desired SCS-analogues 2 and 3 in high yields, 78% and 85% respectively.‡ The antibacterial peptide Bactenecin26 was chosen as a challenging substrate, due to the presence of multiple nucleophilic guanidine groups of the arginine residues, that potentially could react with the electrophilic linker reagent. Nonetheless, SCS-Bactenecin 4 was isolated in 53% yield without the formation, of detectable side-products.
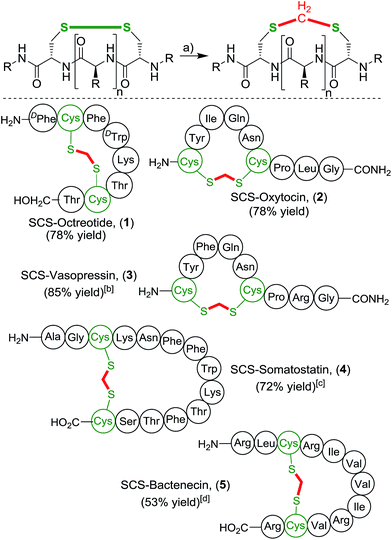 |
| Scheme 3 Employing the thioacetal modification on a variety of cyclic bioactive peptides. Conditions: [a] 1.5 eq. TCEP·HCl, 3.0 eq. K2CO3, 5 : 1 H2O/THF, 23 °C, 2 h, then 4.0 eq. CH2I2, 5.0 eq. NEt3, 23 °C, 9 h; [b] 2.5 eq. CH2I2 used; [c] 1.5 eq. TCEP·HCl, 3.0 eq. K2CO3, 10 : 1 H2O/THF, 23 °C, 2 h, then 10 eq. CH2I2, 15 eq. NEt3, 23 °C, 12 h; [d] 8.0 eq. CH2I2, 12 eq. NEt3. | |
Besides the illustrated modification of unprotected native peptides, the thioacetal introduction can also be embedded in classical peptide synthesis. For instance, the neurotoxic bicyclic hexadecapeptide α-conotoxin MII containing two critical disulfide bridges is a potent and specific competitive antagonist of neuronal nicotinic acetylcholine receptors.27 To prevent disulfide scrambling and to ensure correct oxidative folding of α-conotoxin MII following SPPS, an orthogonal 4-monomethoxytrityl/acetamidomethyl (Mmt/Acm) protecting group strategy was pursued (Scheme 4).28 The thioacetal group proved to be stable towards the acetic acid/iodine deprotection-cyclisation conditions in the formation of 8. The application of our thioacetal stapling protocol was successfully performed twice, yielding the double modified SCS2-α-conotoxin MII in a good overall yield.
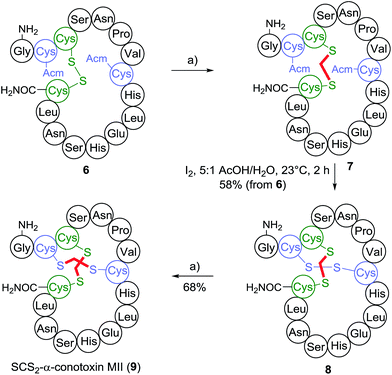 |
| Scheme 4 Synthesis of double thioacetal modified SCSα-conotoxin MII (9) conditions: (a) 1.5 eq. TCEP·HCl, 3.0 eq. K2CO3, 10 : 1 H2O/THF, 23 °C, 2 h, then 6.0 eq. CH2I2, 10 eq. NEt3, 23 °C, 9 h. | |
Stability enhancing thioacetal modification
One key goal of this thioacetal stapling technology was to improve the stability of the modified peptides to various adverse storage and metabolic conditions. The native disulfide bridge is particularly labile under reductive environments and suffers from scrambling reactions in the presence of free thiols. To verify the anticipated reductive stability of the thioacetal modified peptides, OXT and SCS-OXT were incubated at 37 °C in pH 7.0 PBS buffer containing blood levels of L-glutathione (1.0 mM).29 Under these conditions, the half-life of oxytocin is 8 hours (Fig. 1). In contrast, SCS-OXT is, as expected, completely stable and even after 5 days no degradation was observed.
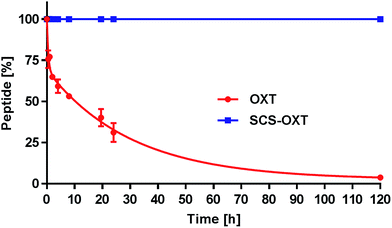 |
| Fig. 1 Reductive stability of SCS-OXT vs. OXT (1.0 mM glutathione in pH 7.0 PBS buffer at 37 °C). Data points are displayed as the mean value ± SEM of three independent experiments. Curves for the stability assays were fitted by non-linear, least squares regression analysis using Prism (GraphPad software version 6.07, La Jolla, CA). | |
This confirmed the inherent chemical stability of a thioacetal moiety against reduction. In further tests, we exposed the SCS analogues of OXT and AVP to human serum at 37 °C (Table 1). For both analogues, we found a significant improvement in the serum half-lives. For SCS-OXT the half-life was more than doubled, equating to an additional 40 hours. In addition, SCS-AVP, displayed an approximately 1.5-fold enhancement in the serum half-life in relation to its parent peptide. OXT is included in the WHO's list of essential medicines. A major concern is its thermal liability, particularly in countries where it is difficult to ensure proper refrigerated handling and storage, as stability studies indicate that liquid oxytocin formulations rapidly degrade at temperatures greater than 30 °C.30 Thus, OXT analogues with an enhanced thermal stability profile would be of significant value. Consequently, we undertook a comparative study to compile thermal and pH stability data between SCS-OXT and its unmodified parent peptide (Table 1). The rate of degradation of the peptides were compared by subjecting them to two different temperatures (40 °C and 55 °C) under four pH values (2.0, 4.5, 7.0 and 9.0). Native oxytocin is most stable under slightly acidic or neutral conditions.30a Overall, we observed sizeable improvements in stability across the majority of temperature and pH conditions. The most significant gains in stability of the thioacetal modified analogue were obtained at the most medically useful, physiological pH (7.0) increasing the half-life approximately 4 to 5-fold. Even at the best storage pH 4.5, the SCS-OXT displays superior stability at 55 °C. Harsher conditions (high temperature and extremes of pH) were more destructive to both peptides due to the relative ease of peptide bond cleavage under such conditions. Nevertheless, at 55 °C and pH 9.0, our SCS-OXT showed a 3.3-fold increase in half-life.
Table 1 Serum, temperature and pH-stability of OXT and SCS-OXTa
Entry |
pH |
OXT half-life [d] |
SCS-OXT half-life [d] |
40 °C |
55 °C |
40 °C |
55 °C |
All data are displayed as the mean value ± SEM (calculated by Prism: GraphPad software version 6.07, La Jolla, CA), of three independent experiments for each pH and temperature variable, and five for the oxytocin serum half-life assay.
At 37 °C.
|
1 |
Serum |
1.2 ± 0.2b |
— |
3.0 ± 0.1b |
— |
2 |
2.0 |
14 ± 0.9 |
3.2 ± 0.4 |
27 ± 0.4 |
3.6 ± 0.4 |
3 |
4.5 |
121 ± 6.2 |
18 ± 1.3 |
133 ± 7.0 |
32 ± 1.7 |
4 |
7.0 |
7.2 ± 0.5 |
2.0 ± 0.2 |
34 ± 1.2 |
8.0 ± 0.4 |
5 |
9.0 |
3.9 ± 0.5 |
0.9 ± 0.15 |
8.6 ± 0.8 |
3.0 ± 0.2 |
Evaluation of the biological activities of the thioacetal modified peptides
Although the introduction of the methylene bridge is the smallest possible reinforcement of a disulfide, it involves a minor structural disturbance. The length and the directionality of the disulfide and the methylene thioacetal are slightly different. The impact of such change might level out with the increasing size of the peptide and the length of the loop. We have therefore assessed the binding affinities of the modified OXT and AVP peptides (Table 2). For the oxytocin receptor (OXTR), SCS-OXT demonstrated a slight decrease in binding affinity, (Ki = 1.41 nM) compared to OXT (Ki = 0.24 nM). For SCS-AVP, the vasopressin receptors V1AR (vasopressor activity), V2R (water retention) and V1BR (neurotransmitter/neuromodulator)31 were assessed. Notably, SCS-AVP has a modulated selectivity profile at the different receptors compared to AVP. SCS-AVP displays similar binding affinity (Ki = 0.77 nM) as the benchmark standard for the V1A receptor, [d(CH2)15, Tyr(Me)2]-AVP (Ki = 0.74 nM).32 For the V2 receptor, SCS-AVP exhibited a slight decrease in affinity (Ki = 4.52 nM vs. AVP's Ki = 1.22 nM). Concerning the V1B receptor, SCS-AVP binds weaker than the parent AVP (Ki = 0.87 nM vs. AVP's Ki = 0.096 nM).
Table 2 Binding affinity and functional activity of OXT, SCS-OXT, AVP and SCS AVPa,b
Entry |
Receptor |
SCS-OXT |
OXT |
SCS-AVP |
AVP |
For each assay, five concentrations were performed in duplicate.
IC50 (or EC50) values were determined by a non-linear, least squares regression analysis using MathIQ™ (ID Business Solutions Ltd., UK) by Eurofins Pharma Discovery Services. Inhibition constants (Ki) were calculated with the Cheng and Prusoff equation using the observed IC50 of the tested compound, the concentration of radioligand employed in the assay, and the historical values for the KD of the ligand.
|
1 |
OXTR Ki [nM] |
1.41 ± 0.3 |
0.24 ± 0.04 |
41 ± 10 |
16 (ref. 31) |
2 |
OXTR EC50 [nM] |
4.90 ± 0.34 |
1.2033 |
— |
— |
3 |
V1AR Ki [nM] |
15 ± 3.5 |
6431 |
0.77 ± 0.23 |
0.74 ± 0.14 |
4 |
V1AR EC50 [nM] |
— |
— |
290 ± 20 |
0.71 ± 0.10 |
5 |
V2R Ki [nM] |
— |
— |
4.52 ± 0.52 |
1.22 ± 0.31 |
6 |
V1BR Ki [nM] |
— |
— |
0.87 ± 0.24 |
0.10 ± 0.04 |
Besides binding affinities, the functional activity of the SCS-analogues was also investigated. An in vitro uterine contraction assay yielded an EC50 = 4.90 nM for SCS-OXT, thus remaining a full agonist with a potency of similar magnitude as OXT (EC50 = 1.20 nM).33 Prior investigations into the effect of changing the ring size in native OXT demonstrated a significant decrease in the agonist potency of the ring-adjusted analogue by a factor of up to 500.34 The macrocyclic ring size modification in oxytocin previously involved the carbon framework of the cysteine residues (homocysteine) and not within the disulfide motif. However, our data suggests that increasing the size of oxytocin's 20-membered ring by insertion of the extra methylene group in between the two sulfur atoms does not significantly reduce its agonist potency. Assaying the activation of the V1A receptor by SCS-AVP resulted in an EC50 of 290 nM (EC50 = 0.71 nM for AVP) with both compounds having essentially the same binding affinity (Ki values for SCS-AVP: 0.77 nM, AVP 0.74 nM). Consequently, the thioacetal modification transforms SCS-AVP to a partial agonist at the V1A receptor. Overall, the modified compounds SCS-OXT and SCS-AVP remain biologically active. The thioacetal bridge can alter the selectivity profile of the parent peptides at the different receptors and has the potential to modulate their ligand efficacy.
Conclusions
In conclusion, we have developed an experimentally facile, one-pot transformation reinforcing disulfide linkages in peptides as thioacetals. No synthetic incorporation of engineered amino acids is needed for this stapling process. The protocol operates under mild, bio-compatible conditions (aqueous solution, mild pH and at ambient temperature) and is tolerant towards unprotected amino acids possessing a variety of functional groups. Furthermore, the high site specificity for the insertion into disulfide bonds further enhanced the reactions utility and versatility. The methylene thioacetal bridge completely eliminates the reductive liability of the disulfide group, contributing to an enhanced structural stability. We have exemplarily shown for oxytocin that the thioacetal analogue exhibits superior stability while maintaining binding affinities and functional activities of the same magnitude. This methodology is an additional tool for stapling peptides using natural linking points with potential impact for the medicinal application of bioactive peptides.
Acknowledgements
This work is supported by the Swiss National Centre of Competence in Research Chemical Biology. We gratefully acknowledge C. Heinis for the use of the peptide synthesiser in his laboratory and K. Johnsson for helpful discussions.
Notes and references
-
(a) A. K. Sato, M. Viswanathan, R. B. Kent and C. R. Wood, Curr. Opin. Biotechnol., 2006, 17, 638–642 CrossRef CAS PubMed;
(b) D. Goodwin, P. Simerska and I. Toth, Curr. Med. Chem., 2012, 19, 4451–4461 CrossRef CAS PubMed;
(c) P. Vlieghe, V. Lisowski, J. Martinez and M. Khrestchatisky, Drug Discovery Today, 2010, 15, 40–56 CrossRef CAS PubMed.
- M. Góngora-Benítez, J. Tulla-Puche and F. Albericio, Chem. Rev., 2014, 114, 901–926 CrossRef PubMed.
-
(a) R. P. Cheng, S. H. Gellman and W. F. DeGrado, Chem. Rev., 2001, 101, 3219–3232 CrossRef CAS PubMed;
(b) S. Y. Hong, J. E. Oh and K.-H. Lee, Biochem. Pharmacol., 1999, 58, 1775–1780 CrossRef CAS PubMed;
(c) M. Tanaka, Chem. Pharm. Bull., 2007, 55, 349–358 CrossRef CAS PubMed;
(d) M. T. Dohm, R. Kapoor and A. E. Barron, Curr. Pharm. Des., 2011, 17, 2732–2747 CrossRef CAS PubMed;
(e) R. Lemmens-Gruber, M. R. Kamyar and R. Dornetshuber, Curr. Med. Chem., 2009, 16, 1122–1137 CrossRef CAS PubMed.
-
(a) C. Proulx, D. Sabatino, R. Hopewell, J. Spiegel, Y. García-Ramos and W. D. Lubell, Future Med. Chem., 2011, 3, 1139–1164 CrossRef CAS PubMed;
(b) J. Vagner, H. Qu and V. J. Hruby, Curr. Opin. Chem. Biol., 2008, 12, 292–296 CrossRef CAS PubMed;
(c) I. E. Valverde, A. Bauman, C. A. Kluba, S. Vomstein, M. A. Walter and T. L. Mindt, Angew. Chem., Int. Ed., 2013, 52, 8957–8960 CrossRef CAS PubMed.
-
(a) Y. H. Lau, P. de Andrade, Y. Wu and D. R. Spring, Chem. Soc. Rev., 2015, 44, 91–102 RSC;
(b) C. J. White and A. K. Yudin, Nat. Chem., 2011, 3, 509–524 CrossRef CAS PubMed.
-
(a) H. Jo, N. Meinhardt, Y. Wu, S. Kulkarni, X. Hu, K. E. Low, P. L. Davies, W. F. DeGrado and D. C. Greenbaum, J. Am. Chem. Soc., 2012, 134, 17704–17713 CrossRef CAS PubMed;
(b) A. M. Spokoyny, Y. Zou, J. J. Ling, H. Yu, Y.-S. Lin and B. L. Pentelute, J. Am. Chem. Soc., 2013, 135, 5946–5949 CrossRef CAS PubMed.
- S. Shaunak, A. Godwin, J.-W. Choi, S. Balan, E. Pedone, D. Vijayarangam, S. Heidelberger, I. Teo, M. Zloh and S. Brocchini, Nat. Chem. Biol., 2006, 2, 312–313 CrossRef CAS PubMed.
-
(a) C. E. Schafmeister, J. Po and G. L. Verdine, J. Am. Chem. Soc., 2000, 122, 5891–5892 CrossRef CAS;
(b) G. L. Verdine and G. J. Hilinksi, Methods Enzymol., 2012, 503, 3–33 CAS;
(c) L. D. Walensky, A. L. Kung, I. Escher, T. J. Malia, S. Barbuto, R. D. Wright, G. Wagner, G. L. Verdine and S. J. Korsmeyer, Science, 2004, 305, 1466–1470 CrossRef CAS PubMed.
-
(a)
A. Holmgren and M. Bjornstedt, in Methods Enzymol., Academic Press, 1995, vol. 252, pp. 199–208 Search PubMed;
(b) G. Powis and W. R. Montfort, Annu. Rev. Pharmacol. Toxicol., 2001, 41, 261–295 CrossRef CAS PubMed.
- C. W. Smith, R. Walter, S. Moore, R. C. Makofske and J. Meienhofer, J. Med. Chem., 1978, 21, 117–120 CrossRef CAS PubMed.
-
(a) N. Assem, D. J. Ferreira, D. W. Wolan and P. E. Dawson, Angew. Chem., Int. Ed., 2015, 54, 8665–8668 CrossRef CAS PubMed;
(b) Z. Dekan, I. Vetter, N. L. Daly, D. J. Craik, R. J. Lewis and P. F. Alewood, J. Am. Chem. Soc., 2011, 133, 15866–15869 CrossRef CAS PubMed;
(c) G. Ösapay, L. Prokai, H.-S. Kim, K. F. Medzihradszky, D. H. Coy, G. Liapakis, T. Reisine, G. Melacini, Q. Zhu, S. H. H. Wang, R.-H. Mattern and M. Goodman, J. Med. Chem., 1997, 40, 2241–2251 CrossRef PubMed.
-
(a) A. D. de Araujo, M. Mobli, J. Castro, A. M. Harrington, I. Vetter, Z. Dekan, M. Muttenthaler, J. Wan, R. J. Lewis, G. F. King, S. M. Brierley and P. F. Alewood, Nat. Commun., 2014, 5, 3165 Search PubMed;
(b) H. Vogt and S. Brase, Org. Biomol. Chem., 2007, 5, 406–430 RSC;
(c) M. Muttenthaler, A. Andersson, A. D. de Araujo, Z. Dekan, R. J. Lewis and P. F. Alewood, J. Med. Chem., 2010, 53, 8585–8596 CrossRef CAS PubMed;
(d) R. Walter and V. du Vigneaud, J. Am. Chem. Soc., 1966, 88, 1331–1332 CrossRef CAS.
-
(a) R. F. Nutt, D. F. Veber and R. Saperstein, J. Am. Chem. Soc., 1980, 102, 6539–6545 CrossRef CAS;
(b) J. L. Stymiest, B. F. Mitchell, S. Wong and J. C. Vederas, Org. Lett., 2003, 5, 47–49 CrossRef CAS PubMed.
- S. Brocchini, S. Balan, A. Godwin, J.-W. Choi, M. Zloh and S. Shaunak, Nat. Protoc., 2006, 1, 2241–2252 CrossRef CAS PubMed.
-
(a) R. J. Clark, H. Fischer, L. Dempster, N. L. Daly, K. J. Rosengren, S. T. Nevin, F. A. Meunier, D. J. Adams and D. J. Craik, Proc. Natl. Acad. Sci. U. S. A., 2005, 102, 13767–13772 CrossRef CAS PubMed;
(b) J. A. Ember, S. D. Sanderson, S. M. Taylor, M. Kawahara and T. E. Hugli, J. Immunol., 1992, 148, 3165–3173 CAS.
-
(a) S. Lindman, G. Lindeberg, A. Gogoll, F. Nyberg, A. Karlén and A. Hallberg, Bioorg. Med. Chem., 2001, 9, 763–772 CrossRef CAS PubMed;
(b) H. I. Mosberg and J. R. Omnaas, J. Am. Chem. Soc., 1985, 107, 2986–2987 CrossRef CAS;
(c) H. I. Mosberg, J. R. Omnaas and A. Goldstein, Mol. Pharmacol., 1987, 31, 599–602 CAS;
(d) M. Ueki, T. Ikeo, K. Hokari, K. Nakamura, A. Saeki and H. Komatsu, Bull. Chem. Soc. Jpn., 1999, 72, 829–838 CrossRef CAS;
(e) M. Ueki, T. Ikeo, M. Iwadate, T. Asakura, M. P. Williamson and J. Slaninova, Bioorg. Med. Chem. Lett., 1999, 9, 1767–1772 CrossRef CAS PubMed.
-
(a) S. Chen, J. Morales-Sanfrutos, A. Angelini, B. Cutting and C. Heinis, ChemBioChem, 2012, 13, 1032–1038 CrossRef CAS PubMed;
(b) C. Heinis, T. Rutherford, S. Freund and G. Winter, Nat. Chem. Biol., 2009, 5, 502–507 CrossRef CAS PubMed;
(c) P. Timmerman, J. Beld, W. C. Puijk and R. H. Meloen, ChemBioChem, 2005, 6, 821–824 CrossRef CAS PubMed.
- Y. Apeloig and M. Karni, J. Chem. Soc., Perkin Trans. 2, 1988, 625–636 RSC.
- J. A. Burns, J. C. Butler, J. Moran and G. M. Whitesides, J. Org. Chem., 1991, 56, 2648–2650 CrossRef CAS.
- D. E. Shafer, J. K. Inman and A. Lees, Anal. Biochem., 2000, 282, 161–164 CrossRef CAS PubMed.
- W. Bauer, U. Briner, W. Doepfner, R. Haller, R. Huguenin, P. Marbach, T. J. Petcher and J. Pless, Life Sci., 1982, 31, 1133–1140 CrossRef CAS PubMed.
-
(a) S. W. J. Lamberts, E. P. Krenning and J.-C. Reubi, Endocr. Rev., 1991, 12, 450–482 CrossRef CAS PubMed;
(b) G. Ösapay and K. Ösapay, Expert Opin. Ther. Pat., 1998, 8, 855–870 CrossRef;
(c) L. Degen and C. Beglinger, Digestion, 1999, 60(suppl. 2), 9–14 CrossRef CAS PubMed.
- P. Brazeau, W. Vale, R. Burgus, N. Ling, M. Butcher, J. Rivier and R. Guillemin, Science, 1973, 179, 77–79 CAS.
-
(a) H.-J. Lee, A. H. Macbeth, J. H. Pagani and W. S. Young 3rd, Prog. Neurobiol., 2009, 88, 127–151 CAS;
(b) V. du Vigneaud, C. Ressler, C. J. M. Swan, C. W. Roberts, P. G. Katsoyannis and S. Gordon, J. Am. Chem. Soc., 1953, 75, 4879–4880 CrossRef CAS.
-
(a) Z. R. Donaldson and L. J. Young, Science, 2008, 322, 900–904 CrossRef CAS PubMed;
(b) V. du Vigneaud, H. C. Lawler and E. A. Popenoe, J. Am. Chem. Soc., 1953, 75, 4880–4881 CrossRef CAS.
- D. Romeo, B. Skerlavaj, M. Bolognesi and R. Gennaro, J. Biol. Chem., 1988, 263, 9573–9575 CAS.
-
(a) G. E. Cartier, D. Yoshikami, W. R. Gray, S. Luo, B. M. Olivera and J. M. McIntosh, J. Biol. Chem., 1996, 271, 7522–7528 CrossRef CAS PubMed;
(b) S. C. Harvey, J. M. Mcintosh, G. E. Cartier, F. N. Maddox and C. W. Luetje, Mol. Pharmacol., 1997, 51, 336–342 CAS.
-
(a) K. B. Akondi, M. Muttenthaler, S. Dutertre, Q. Kaas, D. J. Craik, R. J. Lewis and P. F. Alewood, Chem. Rev., 2014, 114, 5815–5847 CrossRef CAS PubMed;
(b) M. C. Munson and G. Barany, J. Am. Chem. Soc., 1993, 115, 10203–10210 CrossRef CAS.
- F. Michelet, R. Gueguen, P. Leroy, M. Wellman, A. Nicolas and G. Siest, Clin. Chem., 1995, 41, 1509–1517 CAS.
-
(a) A. Hawe, R. Poole, S. Romeijn, P. Kasper, R. van der Heijden and W. Jiskoot, Pharm. Res., 2009, 26, 1679–1688 CrossRef CAS PubMed;
(b)
H. V. Hogerzeil, G. J. A. Walker and M. J. de Goeje, WHO Action Programme on Essential Drugs and Vaccines, 1993, WHO/DAP/93.6 Search PubMed.
-
M. Thibonnier, D. M. Conarty, J. A. Preston, P. L. Wilkins, L. N. Berti-Mattera and R. Mattera, in Oxytocin and Vasopressin: Molecular, Cellular, and Clinical Advances, ed. H. H. Zingg, C. W. Bourque and D. G. Bichet, Plenum Press, New York, 1998, vol. 449, ch. 34, pp. 251–276 Search PubMed.
- M. Kruszynski, B. Lammek, M. Manning, J. Seto, J. Haldar and W. H. Sawyer, J. Med. Chem., 1980, 23, 364–368 CrossRef CAS PubMed.
- Standard reference value for this assay as determined by Eurofins Pharma Discovery Services.
-
(a) D. Jarvis, M. Bodanszky and V. D. Vigneaud, J. Am. Chem. Soc., 1961, 83, 4780–4784 CrossRef CAS;
(b) C. W. Smith and M. F. Ferger, J. Med. Chem., 1976, 19, 250–254 CrossRef CAS PubMed.
Footnotes |
† Electronic supplementary information (ESI) available: Experimental procedures and characterisation of all new compounds. See DOI: 10.1039/c6sc02285e |
‡ General procedure for the synthesis of SCS-OXT To a round bottom flask containing a stirrer bar was added 1.00 eq. of oxytocin acetate powder (3.20 mg, 3.20 μmol) and H2O (3.60 mM). A solution of TCEP·HCl (1.50 eq.) and K2CO3 (3.00 eq.) in H2O (29.0 mM) was added via syringe. The reaction was stirred at room temperature for 1.25 hours. The progress of the reaction was monitored by HPLC-MS. Upon completion of this first step of the process, triethylamine (5.00 eq.) in THF (0.30 M) and then diiodomethane (4.00 eq.) in THF (0.25 M) were added sequentially to the reaction mixture via micro-syringe. The reaction mixture was stirred at room temperature for 6.5 hours. The progress of the reaction was monitored by HPLC-MS. Once adjudged complete, the reaction mixture was diluted with H2O (5.00 mL) and lyophilised. Then, the crude peptide was purified by RP preparative HPLC, using a linear gradient of 5% B to 50% B over 30 minutes at a flow rate of 25.0 mL min−1 with UV detection at 220 nm, 254 nm and 280 nm. (solvent A = 99.9% v/v H2O and 0.1% v/v formic acid; solvent B = 95% v/v MeCN : 5% v/v H2O and 0.1% v/v formic acid). The pure product was isolated in 78% yield (2.50 mg) as a fluffy white powder. |
|
This journal is © The Royal Society of Chemistry 2016 |