DOI:
10.1039/C5SC04706D
(Edge Article)
Chem. Sci., 2016,
7, 3036-3046
Benzo-thia-fused [n]thienoacenequinodimethanes with small to moderate diradical characters: the role of pro-aromaticity versus anti-aromaticity†
Received
7th December 2015
, Accepted 17th January 2016
First published on 19th January 2016
Abstract
Open-shell singlet diradicaloids have recently received much attention due to their unique optical, electronic and magnetic properties and promising applications in materials science. Among various diradicaloids, quinoidal π-conjugated molecules have become the prevailing design. However, the need for a fundamental understanding on how the fusion mode and pro-aromaticity/anti-aromaticity affect their diradical character and physical properties remains unaddressed. In this work, a series of pro-aromatic benzo-thia-fused [n]thienoacenequinodimethanes (Thn-TIPS (n = 1–3) and BDTh-TIPS) were synthesized and compared with the previously reported anti-aromatic bisindeno-[n]thienoacenes (Sn-TIPS, n = 1–4). The ground-state geometric and electronic structures of these new quinoidal molecules were systematically investigated by X-ray crystallographic analysis, variable temperature NMR, ESR, SQUID, Raman, and electronic absorption spectroscopy, assisted by DFT calculations. It was found that the diradical character index (y0) increased from nearly zero for Th1-TIPS to 2.4% for Th2-TIPS, 18.2% for Th3-TIPS, and 38.2% for BDTh-TIPS, due to the enhanced aromatic stabilization. Consequently, with the extension of molecular size, the one-photon absorption spectra are gradually red-shifted, the two-photon absorption (TPA) cross section values increase, and the singlet excited state lifetimes decrease. By comparison with the corresponding anti-aromatic analogues Sn-TIPS (n = 1–3), the pro-aromatic Thn-TIPS (n = 1–3) exhibit larger diradical character, longer singlet excited state lifetime and larger TPA cross section value. At the same time, they display distinctively different electronic absorption spectra and improved electrochemical amphotericity. Spectroelectrochemical studies revealed a good linear relationship between the optical energy gaps and the molecular length in the neutral, radical cationic and dicationic forms. Our research work discloses a significant difference between the pro-aromatic and anti-aromatic quinoidal compounds and provides guidance for the design of new diradicaloids with desirable properties.
Introduction
Quinoidal π-conjugated compounds exhibit distinctively different features from those of aromatic molecules, for example, low band gap, redox amphotericity, and open-shell diradical character, which render them useful for application in organic near-infrared (NIR) dyes, n-channel or ambipolar field effect transistors (FETs), non-linear optics and spin-based electronics.1 In particular, their natural tendency to recover the aromaticity of the non-aromatic quinoidal units could lead to an open-shell singlet diradical ground state where the singlet diradical solution has lower energy than the closed-shell singlet. In recent years, various open-shell singlet diradicaloids based on quinoidal π-conjugated molecules have been developed, including quinoidal oligothiophenes,2 quinoidal thienoacenes,3 and many quinoidal polycyclic hydrocarbons such as bisphenalenyls,4 zethrenes,5 indenofluorenes,6 and extended para-quinodimethane (p-QDM) oligomers.7 The typical designs include: (1) construction of oligomers of quinoidal benzene or thiophene; and (2) incorporation of a quinodimethane unit into an aromatic π-framework. To ensure stability, kinetic blocking of the most reactive sites is usually necessary. Previous studies also revealed that several factors such as aromatic stabilization and steric strain release could significantly affect the singlet diradical character and consequently their physical properties and chemical reactivity. Generally, larger aromatic stabilization energy and lower steric strain in the diradical form favor an open-shell singlet diradical ground electronic state. These studies also demonstrated that pro-aromatic and anti-aromatic π-conjugated molecules have an irresistible tendency to be diradicals. “Anti-aromaticity” is a characteristic of compounds that contain 4n π-electrons in a cyclic planar, or nearly planar, system of alternating single and double bonds. A “pro-aromatic” compound refers to a non-aromatic molecule containing a quinodimethane like structure which has high tendency to become an open-shell diradical and/or zwitterionic form containing one or more aromatic sextets. The diradical character index (y0) and singlet–triplet energy gap (ΔES–T) are two important parameters that can be correlated to third-order non-linear optical (NLO) properties and magnetic activity of open-shell singlet diradicaloids.8 However, a fundamental understanding of how the fusion mode and the pro-aromaticity/anti-aromaticity affect their ground-state electronic structures and consequently their physical properties has not yet been attained.
Recently, our group and Haley's group independently reported the synthesis and properties of a series of quinoidal bisindeno-[n]thienoacenes (Sn-TIPS) (n = 1–4) (Fig. 1), which are better regarded as an anti-aromatic system because the NICS(1)zz values of the cyclopenta- and the central thiophene rings are significantly positive.9 It turns out that the monomer to trimer (n = 1–3) all have negligible diradical character and only the tetramer has a significant diradical character (y0 = 20.2% at the UCAM-B3LYP/6-31G* level of theory). Our attention was then switched to their analogues, the benzo-thia-fused [n]thienoacenequinodimethanes (Thn-TIPS (n = 1–3) and BDTh-TIPS), in which the diradical resonance form is supposed to contribute significantly to the ground-state structure due to the recovery of aromaticity of the central quinoidal thienoacene unit (Fig. 1). In principle, a monocycle containing 4n π electrons can be also drawn for these molecules, implying a potential anti-aromatic character. However, our calculations indicate that the NICS(1)zz values of the central thiophene/benzene rings are significantly negative (vide infra), and thus they are better regarded as a pro-aromatic system with small anti-aromaticity. Comparison between these two systems will provide us with further knowledge about the influence of aromaticity or anti-aromaticity in the electronic and physical properties. Therefore, in this work, we will present systematic studies on: (1) the efficient synthesis of these pro-aromatic quinoidal molecules; (2) their ground-state geometric and electronic structures probed by various experimental methods and theoretical calculations; (3) their optical, electrochemical and magnetic properties; (4) a detailed comparison with the anti-aromatic compounds, Sn-TIPS; and (5) the relationships between the pro-aromaticity/anti-aromaticity, diradical character and physical properties. Our intention is to provide better insight into the design of quinoidal diradicaloids with desirable properties.
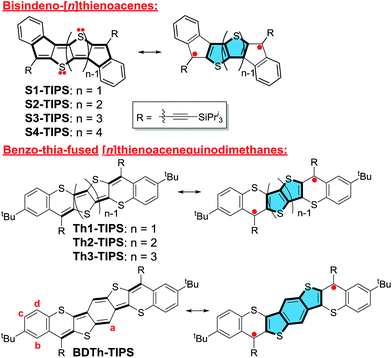 |
| Fig. 1 Structures of anti-aromatic bisindeno-[n]thienoacenes and pro-aromatic quinoidal benzo-thia-fused [n]thienoacenequinodimethanes. | |
Results and discussion
Synthesis
The synthetic strategy towards these quinoidal compounds was based on the synthesis of the corresponding diketones by intramolecular Friedel–Crafts acylation reaction, followed by nucleophilic addition with triisopropylsilylethynyl lithium (Li-TIPSE) and then by reduction of the intermediate diols with SnCl2 (Schemes 1 and 2).
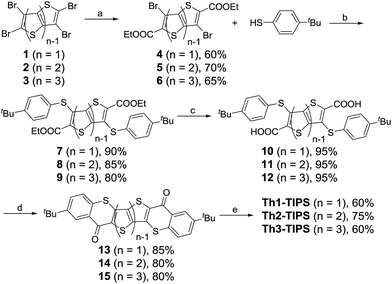 |
| Scheme 1 Synthetic route to Thn-TIPS. Reagents and conditions: (a) (i) n-BuLi, dry THF, −78 °C; (ii) NCCOOEt, −78 °C – r.t., overnight; (iii) H2O, 0 °C; (b) Pd2(dba)3, dppf, DMF, iPr2NEt, 100 °C, overnight; (c) (i) NaOH, EtOH, reflux overnight; (ii) 10% HCl (aq.); (d) (i) SOCl2, DMF, DCM, reflux; (ii) AlCl3, dry DCM, 0 °C – r.t., overnight; (e) (i) TIPSCCLi, dry THF, 0 °C – r.t.; (ii) SnCl2, 6 h. | |
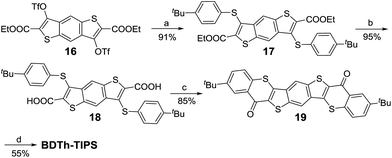 |
| Scheme 2 Synthetic route to BDTh-TIPS. Reagents and conditions: (a) 4-tert-butylbenzenethiol, K2CO3, DMF, r.t., 12 h; (b) (i) NaOH, EtOH, reflux overnight; (ii) 10% HCl (aq.); (c) (i) SOCl2, DMF, dry DCM, reflux; (ii) AlCl3, dry dichloromethane, 0 °C – r.t., overnight; (d) (i) TIPSCCLi, THF, 0 °C – r.t.; (ii) SnCl2, 6 h. | |
The key intermediates for the synthesis of Thn-TIPS (n = 1–3) are the dibromo-diesters 4–6, which were first synthesized in 60–70% yield by regio-selective lithiation of the corresponding tetrabromides 1–3 followed by quenching with ethyl cyanoformate in a one-pot protocol (Scheme 1).3b,10 Compounds 4–6 underwent palladium-catalyzed cross-coupling reaction11 with 4-tert-butylbenzenethiol to give compounds 7–9 in 80–90% yield. Compounds 7–9 were then hydrolyzed and acidified to form diacids 10–12 in 95% yield and the diacids were converted into the corresponding carboxylic acid chlorides by reaction with thionyl chloride in dry dichloromethane (DCM). Subsequent double Friedel–Crafts acylation with aluminium chloride afforded the desired diketones 13–15 in 80–85% yield. Compound Thn-TIPS (n = 1–3) was then obtained in an overall 60–75% yield by addition of Li-TIPSE to the corresponding diketones 13–15 followed by reductive dehydroxylation with SnCl2.
The synthetic route to BDTh-TIPS shown in Scheme 2 is slightly different from that to Thn-TIPS (n = 1–3). Instead of dibromo-diesters 4–6, the ditriflate-diester compound 1612 was used to carry out a direct nucleophilic substitution reaction with 4-tert-butylbenzenethiol under basic conditions to give compound 17 in 91% yield as the triflate is a good leaving group. Subsequent reactions following a similar protocol to that shown in Scheme 1 gave the target compound BDTh-TIPS in an acceptable yield. Compounds Thn-TIPS (n = 1–3) and BDTh-TIPS showed good stability both in solution and in the solid state, and their structures were unambiguously confirmed by X-ray crystallographic analysis (vide infra).
Electronic absorption spectra
Recent studies demonstrated that electronic absorption spectra give an initial insight to identify an open-shell singlet diradical ground electronic state based on the presence of a weak low-energy absorption band in the NIR region.13 The UV-vis-NIR absorption spectra of all compounds are shown in Fig. 2 and the data are collected in Table 1. Th1-TIPS and Th2-TIPS show an intense band with maxima at 570 nm and 640 nm, respectively, which is very similar to the p-band of many closed-shell polycyclic aromatic hydrocarbons such as acenes and rylenes14 and closed-shell quinoidal compounds such as heptazethrene.5e The structure of this band is dramatically different from that of their counterparts S1-TIPS and S2-TIPS, in which a broad absorption band was observed in this region attributed to their anti-aromatic character.9b The main absorption peak red-shifts to 666 nm in Th3-TIPS and 702 nm in BDTh-TIPS, and the spectra again are very different from their anti-aromatic analogue S3-TIPS.
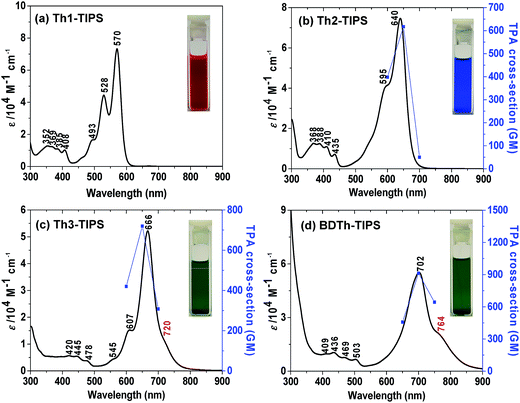 |
| Fig. 2 One-photon absorption spectra (solid lines and left vertical axes) in DCM and two-photon absorption spectra in toluene (blue symbols and right vertical axes) of (a) Th1-TIPS, (b) Th2-TIPS, (c) Th3-TIPS and (d) BDTh-TIPS. The TPA spectrum of Th1-TIPS was not recorded due to the limit of measurement range. TPA spectra are plotted at λex/2. Inserted are the photos of the solutions. | |
Table 1 Summary of the photophysical and electrochemical dataa
Comp |
λ
abs (nm) |
ε (104 M−1 cm−1) |
E
ox1/2 (V) |
E
red1/2 (V) |
HOMO (eV) |
LUMO (eV) |
E
ECg (eV) |
E
optg (eV) |
τ (ps) |
σ
(2)
max (GM) (λex) |
λ
abs: absorption maxima. ε: molar extinction coefficient for the corresponding absorption maximum. Eox1/2 and Ered1/2: half-wave potentials of the oxidative and reductive waves, respectively, with potentials vs. Fc/Fc+ couple. HOMO = −(4.8 + Eonsetox) and LUMO = −(4.8 + Eonsetred), where Eonsetox and Eonsetred are the onset potentials of the first oxidative and reductive waves, respectively. EECg: electrochemical band gap. Eoptg: optical band gap estimated from the absorption onset. τ: singlet excited lifetime obtained from TA. σmax(2): maximum TPA cross section. λex: excitation wavelength in TPA measurements.
|
Th1-TIPS
|
570 |
7.32 |
0.05 |
−1.83 |
−4.77 |
−3.06 |
1.71 |
2.05 |
3 (τ1) |
— |
0.55 |
−2.11 |
5800 (τ2) |
Th2-TIPS
|
640 |
7.46 |
−0.13 |
−1.71 |
−4.56 |
−3.17 |
1.39 |
1.90 |
1.2 (τ1) |
620 (1300 nm) |
0.32 |
−1.90 |
2800 (τ2) |
Th3-TIPS
|
666 |
5.23 |
−0.25 |
−1.59 |
−4.42 |
−3.35 |
1.07 |
1.65 |
20 (τ1) |
720 (1300 nm) |
0.14 |
−1.80 |
1700 (τ2) |
BDTh-TIPS
|
702 |
5.52 |
−0.26 |
−0.83 |
−4.39 |
−3.40 |
0.99 |
1.46 |
40 (τ1) |
910 (1400 nm) |
0.07 |
−1.73 |
820 (τ2) |
The most distinctive feature, however, is that the spectra of Th3-TIPS and BDTh-TIPS become broadened, and weak low-energy absorptions, as shoulders of the main bands, appear at 720 and 764 nm, respectively. This is strong evidence of the change of the ground state of this series of molecules with extension of the molecular size. This change was also observed in the zethrene series5 and the lowest energy absorption shoulder originates from the presence of a low-lying singlet excited state dominated by a doubly excited electronic configuration (H,H → L,L).13 This observation indicates an increase of diradical character with the increase of the molecular length which can be explained by the recovery of more aromatic thiophene rings in the diradical form. The more broadened and red-shifted spectrum observed in BDTh-TIPS compared with its analogue Th3-TIPS also implies a larger diradical character, which can be accounted for by the more aromatic character of the benzene ring compared to the thiophene ring. In addition, only Th1-TIPS exhibits a modest fluorescence signal at 593 nm (Fig. S1 in ESI†).
Magnetic measurements
Compounds Thn-TIPS (n = 1–3) all show well-resolved 1H NMR spectra in solution even at elevated temperatures. However, significant signal broadening was observed for BDTh-TIPS in toluene-d8 at room temperature and this became more obvious upon heating, while cooling of the solution resulted in signal sharpening (Fig. 3). This is a typical phenomenon for many open-shell singlet diradicaloids and the NMR signal broadening results from a thermally excited triplet species which is usually slightly higher in energy than the singlet diradical form. The gradual low-field shift of the resonance for protons in the central benzene ring (protons a in Fig. 1) with the increase of temperature also indicates a transformation to a larger aromatic character at higher temperatures.
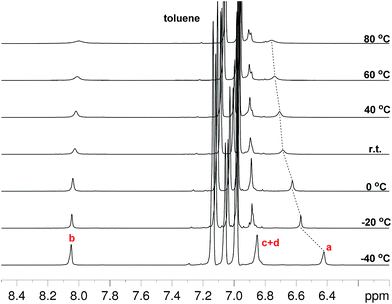 |
| Fig. 3 VT 1H NMR spectra (aromatic region) of BDTh-TIPS in toluene-d8 and assignment of all aromatic protons. The resonance assignment referred to the structure displayed in Fig. 1. | |
In accordance with the NMR measurements, solutions of Thn-TIPS (n = 1–3) all exhibited no ESR signal, which can be correlated to either a closed-shell singlet ground state or an open-shell singlet diradical ground state but with a large singlet-triplet energy gap. However, BDTh-TIPS in toluene displayed a broad single-line ESR spectrum with ge = 2.0038 and no half-field spectrum (Δms = ±2 transition) could be detected due to the large spin delocalization (vide infra), and a similar phenomenon was observed for other delocalized singlet diradicaloids (Fig. 4a). Temperature-dependent magnetic susceptibility data on the powder of BDTh-TIPS at 5–380 K were collected using a superconducting quantum interference device (SQUID) magnetometer. Fig. 4b plots χT as a function of T. An increasing susceptibility above 200 K was observed for the powder BDTh-TIPS, and fitting the data by using the Bleaney–Bowers equation15 gave an approximate singlet–triplet energy gap (ΔES–T or 2J/kB) of −1969.7 K (−0.17 eV or −3.91 kcal mol−1). Therefore, BDTh-TIPS has a singlet open-shell ground state, and at room temperature its first triplet excited state can be populated because of the small singlet–triplet energy gap, which explains the ESR signal.
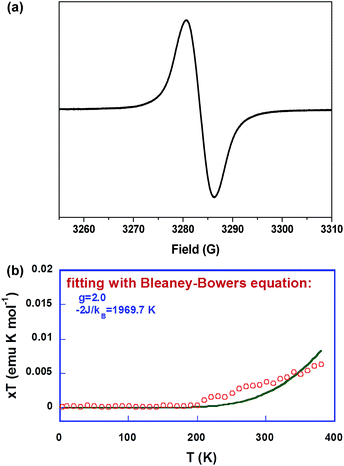 |
| Fig. 4 (a) ESR spectrum of BDTh-TIPS in toluene solution measured at room temperature. (b) χT–T plot for the solid BDTh-TIPS. The measured data was plotted as open circles, and the fitting curve was drawn using the Bleaney–Bowers equation with g = 2.00. | |
X-ray crystallographic analysis
Single crystals suitable for X-ray crystallographic analysis were obtained for Thn-TIPS (n = 1–3) and BDTh-TIPS by slow diffusion of acetonitrile or methanol into their solutions.16 The Oak Ridge Thermal Ellipsoid Plot (ORTEP) drawings and 3D packing structures are shown in Fig. 5. The π-conjugated frameworks of all molecules are nearly planar. All three Thn-TIPS (n = 1–3) molecules crystallize in a triclinic lattice system, with space group P
, whereas BDTh-TIPS crystallizes in a monoclinic lattice system, with space group P2(1)/n. For Th1-TIPS, two molecules form an anti-parallel packed dimer via π–π interactions with a distance about 3.57 Å. The average distance between adjacent dimers is more than 4.2 Å hence no close π-stacking was observed between each dimer. For Th2-TIPS, two molecules also form an anti-parallel packed dimer via π–π interaction (distance: 3.51 Å), which further arranges into a columnar structure with an inter-dimer distance of 3.59 Å. Similarly, two of the Th3-TIPS molecules also form a dimer via π–π interaction (distance: 3.37 Å) and the dimers are further packed into columnar structure via multiple close π–π interactions but the neighbouring dimers are rotated for about 70° to suppress steric hindrance. BDTh-TIPS shows a π-stacked columnar structure with a close π–π distance of about 3.35 Å. The observed close packing in Th2-TIPS, Th3-TIPS and BDTh-TIPS indicates that they could serve as good semiconductors in FETs in future studies.
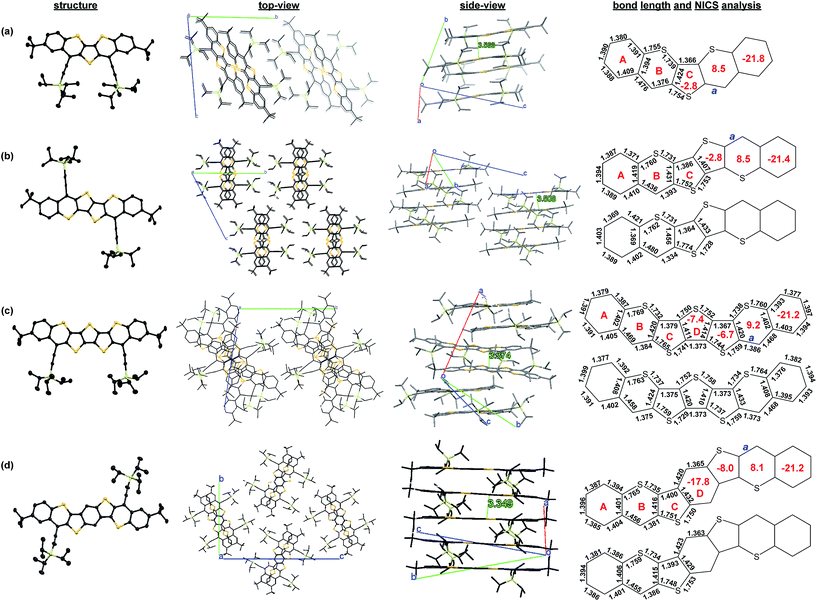 |
| Fig. 5 ORTEP drawings, 3D packing structures (both top-view and side-view), and selected bond lengths of (a) Th1-TIPS, (b) Th2-TIPS, (c) Th3-TIPS and (d) BDTh-TIPS. Hydrogen atoms are omitted for clearance. The red numbers in the rings denote the calculated NICS(1)zz values. | |
Bond length analysis was performed to better analyze the ground-state geometry. Although these molecules are in central C2v plane symmetry, two sets of molecules with different bond lengths are observed for Th2-TIPS, Th3-TIPS and BDTh-TIPS in the crystalline form due to the packing effect (Fig. 5). In all cases, large bond length alternation was observed for the central thienoacenequinodimethane unit, indicating a dominant quinoidal structure. The exo-methylene bond has a length of 1.376 Å, 1.393/1.334 Å, 1.384/1.386/1.373/1.375 Å and 1.381/1.386 Å for Th1-TIPS, Th2-TIPS, Th3-TIPS and BDTh-TIPS, respectively. DFT calculations (vide infra) predict the respective length as 1.363, 1.374, 1.389, 1.399 Å, implying an increase of diradical character with chain length, which is in accordance with the above electronic absorption spectroscopic and NMR/ESR/SQUID studies. The outermost two benzene rings in all four molecules display small bond length alternation, indicating their large aromatic character and minor impact onto the electronic properties of this series of molecules.
DFT calculations
Broken symmetry DFT calculations (UCAM-B3LYP/6-31G*) were conducted to understand the ground-state electronic structures. The results showed that the shortest molecule Th1-TIPS indeed favors a closed-shell ground state. However, for Th2-TIPS, Th3-TIPS and BDTh-TIPS, the energies of their open-shell singlet diradical states are 9.27/1.36, 5.14/3.56 and 3.38/8.26 kcal mol−1 lower than the triplet biradical/closed-shell state, respectively, thus defining their singlet diradical ground electronic states. The HOMO and LUMO wave-functions of Th1-TIPS are delocalized along the whole π-conjugated framework (Fig. 6a). The highest SOMO-α and SOMO-β profiles showed an increasing disjoint character from Th2-TIPS to Th3-TIPS and then to BDTh-TIPS, with the spins evenly distributed along the whole π-conjugated framework (Fig. 6b–d). The singlet diradical character index (y0) values were calculated as 0.024, 0.182 and 0.382 for Th2-TIPS, Th3-TIPS and BDTh-TIPS, respectively, while negligible for Th1-TIPS. Therefore, the singlet diradical character increases with the extension of molecular size. Although the molecular length is almost the same in Th3-TIPS and BDTh-TIPS, the latter shows a significantly larger diradical character, which can be ascribed to their different quinoidal structures. That is, instead of the thiophene ring in Th3-TIPS, the benzene ring in BDTh-TIPS would lead to more aromatic resonance energy recovery in the diradical form and hence promote the diradical character. Another noticeable observation is that Th2-TIPS and Th3-TIPS have a remarkably larger diradical character than their anti-aromatic counterparts S2-TIPS (y0 = 0) and S3-TIPS (y0 = 0.03), which have negligible diradical character.9b Considering their structural similarity, such differences must be associated with their differential fusion modes. It appears that replacing two indene units in Sn-TIPS by two benzo-thia units in Thn-TIPS significantly promotes the diradical character and consequently affects their physical properties. This can be correlated to a fundamental change from anti-aromatic Sn-TIPS to pro-aromatic Thn-TIPS. These results also demonstrated that the diradical character and physical properties of quinoidal compounds are not only dependent on the length of the central quinoidal unit, but also the overall pro-aromaticity and anti-aromaticity of the molecules. It seems that pro-aromatic molecules show larger diradical character in comparison to their anti-aromatic analogues with a similar molecular size. In fact, the diradical character of BDTh-TIPS is larger than for the higher order anti-aromatic S4-TIPS (y0 = 0.202).9b
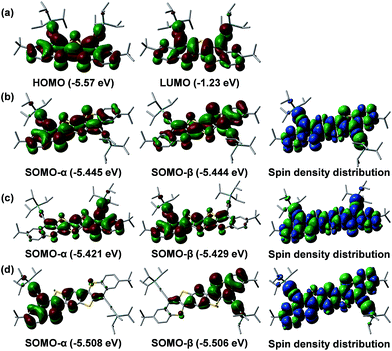 |
| Fig. 6 Calculated frontier MO profiles of Th1-TIPS (a) and SOMO profiles and spin density distribution of the singlet diradical of Th2-TIPS (b), Th3-TIPS (c) and BDTh-TIPS (d). | |
Nucleus independent chemical shift (NICS) calculations were conducted to provide the trend of aromaticity of each ring (Fig. 5). From Th1-TIPS to Th2-TIPS, to Th3-TIPS and to BDTh-TIPS, the NICS(1)zz values for the central quinoidal rings (ring C and D) become more negative, indicating an increase of aromaticity with the extension of the molecular size, which is in accordance with the increased diradical character. At the same time, in contrast to their counterparts Sn-TIPS compounds, the NICS(1)zz values for the central quinoidal rings of Thn-TIPS and BDTh-TIPS are more negative, indicating a larger aromaticity in Thn-TIPS and BDTh-TIPS while Sn-TIPS are typically anti-aromatic. The sulfur-containing six member rings (ring B) show positive NICS(1)zz values, indicating a non-aromatic to anti-aromatic character. Such sulfur containing six member rings are further annulated and stabilized by two aromatic benzene rings (ring A) possessing large negative NICS(1)zz values.
Raman spectroscopic measurements
Raman spectroscopy has been proven to be very useful for the characterization of the ground electronic state of π-conjugated molecules and in particular those oligomer series with quinoidal/aromatic transformations.17 Therefore, the Raman spectra of Thn-TIPS and BDTh-TIPS were recorded in a powder form with different excitation wavelengths (Fig. 7). Generally, there are two main characteristics in the frequency and intensity behavior: (1) there is a main band that dominates the spectra of Th1-TIPS–Th3-TIPS and that progressively downshifts from 1434 cm−1 in Th1-TIPS to 1358 cm−1 in Th3-TIPS whose frequency positions are typical of quinoidal tetracyano oligothiophenes.2d The overall shift is of 76 cm−1, which compares with the 61 cm−1 in the S1-TIPS–S3-TIPS compounds, and is in agreement with a more efficient electron delocalized Thn-TIPS systems free of any anti-aromatic electron pinning effect; (2) passing from Th3-TIPS to BDTh-TIPS, the main Raman band is split in two 1354/1347 cm−1 components indicating that from the main band in Th3-TIPS at 1358 cm−1 the whole 76 cm−1 downshift on Th1-TIPS → Th3-TIPS collapses or is close to collapse on Th3-TIPS → BDTh-TIPS. Furthermore, a new band at 1423 cm−1 appears in BDTh-TIPS which is at frequencies in between those of Th1-TIPS and Th2-TIPS allowing it to be assigned as a quinoidal feature in BDTh-TIPS. This spectral behaviour can be explained by a saturation of the quinoidization effect from Thn-TIPS (n = 1–3) to BDTh-TIPS resulting from a pseudo-aromatization in the central benzene of the conjugated backbone accompanied by a portion of quinoidal contribution on the thiophene rings. A similar behaviour has been reported in a quinoidal tetracyano-quaterthiophene and ascribed to the formation of a diradicaloid singlet ground electronic state with a pseudo-aromatic (pseudo-quinoidal) central (peripheral) structure on its rings.2d
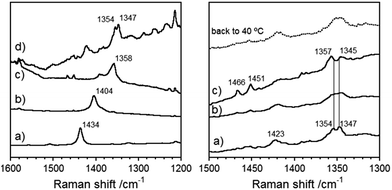 |
| Fig. 7 Left: 785 nm Raman spectrain solid state of: (a) Th1-TIPS, (b) Th2-TIPS, (c) Th3-TIPS and (d) BDTh-TIPS. Right: Raman spectra of BDTh-TIPS in solid state at (a) −140 °C, (b) 40 °C, (c) +140 °C. | |
Variable-temperature Raman experiments on BDTh-TIPS in Fig. 7 are performed to scan the evolution of the diradical ground electronic state (singlet) into the lowest energy lying excited state (triplet). On heating up to 140 °C, the most noticeable finding is the coalescence of the 1423 cm−1 thieno-quinoidal band and the simultaneous emergence of two new medium bands at 1466/1451 cm−1. Characteristic Raman bands around 1460 cm−1 have been reported for aromatic thienoacene cores18 meaning that the clearance of the quinoidal band (1423 cm−1) is at the expenses of the rise of aromatic ones (1466/1451 cm−1). In addition the pre-existing most intense Raman scatterings around 1350 cm−1 enlarge their separation from low temperature (7 cm−1) to high (12 cm−1) temperature revealing the transformation between two species, one at low temperature with a less defined aromatic structure (pseudo-aromatic or singlet with incipient diradical structure) and another at high temperatures with more distinguished aromatic feature typical of diradical triplets. This study is also in agreement with the above mentioned VT NMR/ESR/SQUID measurements.
TA and TPA measurements
Femtosecond transient absorption (TA) measurements were then utilized to explore the excited-state dynamics of Thn-TIPS (n = 1–3) and BDTh-TIPS. Their TA spectra in toluene exhibit a strong ground-state bleaching (GSB) band near their steady-state absorption maximum (Fig. 8). On the other hand, they all display weak excited-state absorption (ESA) bands which are similar to the pro-aromatic diradicaloids such as heptazethrene and octazethrene derivatives5e but distinctively different from their anti-aromatic counterparts Sn-TIPS.9b The decay curves can be fitted by a bi-exponential process and the singlet excited-state lifetimes of Thn-TIPS (n = 1–3) and BDTh-TIPS were estimated to be 5800, 2800, 1700 and 820 ps (Table 1 and Fig. S2 in ESI†), respectively, which are much longer than for the anti-aromatic Sn-TIPS (7–12 ps),9b again highlighting the dramatic difference between the pro-aromatic and anti-aromatic electronic structures and their impact on the electronic excited states. Notably, the singlet excited-state lifetimes decrease with the increment of molecular length, which is consistent with a gradually increased diradical character. As a consequence, modest fluorescence was observed for Th1-TIPS but no emission was detected for Th2-TIPS, Th3-TIPS, and BDTh-TIPS.19
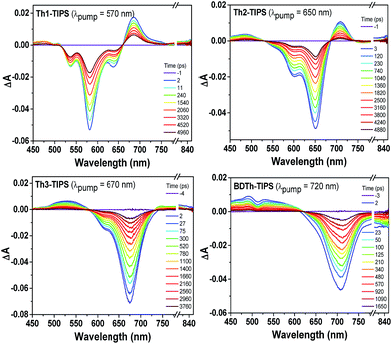 |
| Fig. 8 Femtosecond transient absorption spectra of Thn-TIPS (n = 1–3) and BDTh-TIPS measured in toluene with photoexcitation at 570, 650, 670, and 720 nm, respectively. | |
π-Conjugated molecules with a small and moderate diradical character are theoretically predicted by Nakano et al. to show a largely enhanced third-order NLO response with large TPA cross sections.20 Thus, TPA measurements were also conducted for Thn-TIPS (n = 2 and 3) and BDTh-TIPS in toluene by using Z-scan technique in the NIR region from 1200 to 1500 nm where the OPA contribution is negligible (Fig. 2, Table 1 and Fig. S3 in ESI†). The maximum TPA cross section values (σmax(2)) increase from 620 GM (λex: 1300 nm) for Th2-TIPS to 720 GM (λex: 1300 nm) for Th3-TIPS and to 910 GM (λex: 1400 nm) for BDTh-TIPS with the increment of the diradical character, and they are much larger than those of their anti-aromatic counterparts Sn-TIPS (σmax(2) = 420 GM for S2-TIPS, and σmax(2) = 520 GM for S3-TIPS). Unfortunately, the short OPA absorption maximum of Th1-TIPS pushes its TPA measurements out of our instrument measuring range. Anyway, again, we showed that the pro-aromatic compounds exhibited a stronger third-order NLO response than their anti-aromatic counterparts with a similar molecular size, and the larger the diradical character, the larger the TPA cross section value.
Electrochemical and spectroelectrochemical studies
The electrochemical properties of all compounds were investigated by cyclic voltammetry (CV) in dry DCM solution (Fig. 9 and Table 1). All compounds display amphoteric redox behaviour with four-stage (quasi-) reversible redox waves. Th1-TIPS exhibited two reversible oxidation waves with half-wave potential Eox1/2 at 0.05 V, 0.55 V and two quasi-reversible reduction waves at Ered1/2 = −1.83 V, −2.11 V (vs. Fc+/Fc, Fc: ferrocene). Th2-TIPS and Th3-TIPS also displayed two oxidation waves at Eox1/2 = −0.13 V, 0.32 V and Eox1/2 = −0.25 V, 0.14 V as well as two reduction waves at Ered1/2 = −1.71 V, −1.90 V and Ered1/2 = −1.59 V, −1.80 V, respectively. Similarly, BDTh-TIPS showed two oxidation waves at Eox1/2 = −0.26 V, 0.07 V and two reduction waves at Ered1/2 = −0.83 V, −1.73 V. The HOMO and LUMO energy levels determined from the onset of the first oxidation and reduction waves, respectively, are summarized in Table 1. It is clear that extension of the molecular size from Th1-TIPS to BDTh-TIPS leads to a rising of the HOMO energy level and lowering of LUMO energy level, and consequently a decrease of the electrochemical energy band gaps (EECg), which is consistent with the optical energy gap (Eoptg) determined from the lowest-energy absorption band onset. For comparison, the anti-aromatic counterparts Sn-TIPS (n = 1–3) are difficult to oxidize due to the local anti-aromatic character of the cyclopentadienyl cation but easy to reduce due to the aromatic character of the cyclopentadienyl anion. The pro-aromatic character of our new series of molecules showed good amphotericity because both oxidation and reduction will lead to recovery of the aromaticity of the central quinoidal thienoacene moiety and there are no other anti-aromatic units.
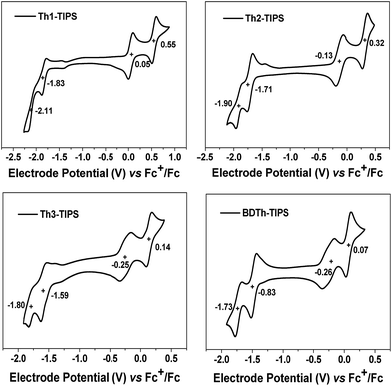 |
| Fig. 9 Cyclic voltammograms of Th1-TIPS, Th2-TIPS, Th3-TIPS and BDTh-TIPS in dry CH2Cl2 containing 0.1 M Bu4NPF6 as the supporting electrolyte, AgCl/Ag as the reference electrode, Au as the working electrode, Pt wire as the counter electrode, and a scan rate of 50 mV s−1. The potential was externally calibrated against the ferrocene/ferrocenium redox couple. | |
The multistage reversible redox waves and the large separation between the redox waves allow us to quantitatively attain the singly and doubly charged species by electrochemistry. UV-vis-NIR spectroelectrochemical measurements were thus conducted for Thn-TIPS (n = 1–3) and BDTh-TIPS in DCM containing 0.1 M n-Bu4NPF6 as the supporting electrolyte by applying different electrode potentials and the absorption spectra were monitored in situ in the UV-vis-NIR range. Fig. 9 shows the electronic absorption spectra of the four compounds in their different redox states according to their electrochemical properties measured by cyclic voltammetry.
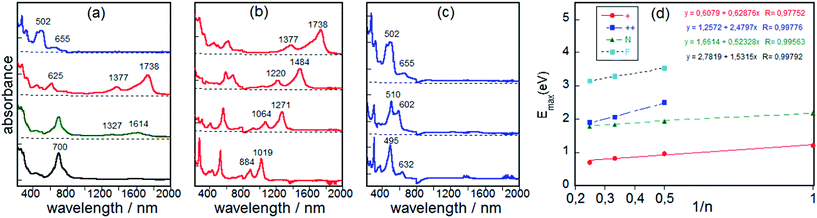 |
| Fig. 10 (a) UV-vis-NIR absorption spectra obtained by electrochemical redox treatment of the neutral BDTh-TIPS (black line), radical cation-1 (green line), radical cation (red line) and dication (blue line); (b) spectra of the radical cations of Th1-TIPS, Th2-TIPS, Th3-TIPS and BDTh-TIPS (from the bottom to the top); (c) spectra of the dications of Th2-TIPS, Th3-TIPS and BDTh-TIPS (from the bottom to the top); (d) representation of the absorption maxima as a function of the reciprocal of the chain length (N: neutral; +: radical cation; ++: dication; F: thienoacenes). | |
One electron oxidation of BDTh-TIPS gives rise to the appearance, at the initial stage of the oxidation, of two weak bands at 1327 and 1614 nm which quickly convert into a spectrum with stronger bands at 625, 1377 and 1738 nm (Fig. 10a). During this first oxidation, the two described spectra coexist. The 625/1377/1738 nm spectrum of the radical cation of BDTh-TIPS is surprisingly similar to that described for the radical anions of the Sn-TIPS (n = 1–3).9b The UV-vis-NIR absorption spectra of the radical cations of Th1-TIPS, Th2-TIPS and Th3-TIPS have also been recorded in Fig. 10 which show quite similar patterns to that of BDTh-TIPS (Fig. 10b).
One-electron oxidation of the radical cation produces the clearance of the NIR bands and the growth of intense absorption bands in the visible 500–600 nm region (Fig. 10a and c; a reliable spectrum for the dication of Th1-TIPS was not obtained). We assign the characteristic bands of the dication to the bands at 495 nm for Th2-TIPS, at 602 nm for Th3-TIPS, and at 655 nm for BDTh-TIPS. Interestingly, a linear relationship between the energy gap λmax (eV) with the reciprocal number of the chain length (1/n, herein n is the number of thiophene rings in the central quinoidal thienoacene unit and BDTh-TIPS was assumed to be an equivalence of Th4-TIPS which was not synthesized due to synthetic challenges) was observed for the neutral, radical cationic, and dicationic species (Fig. 10d). The slope for the dications is greater than for the radical cations, likely revealing the strongest pinning of the electron wavefunction. We have also represented the variation λmax (eV)–1/n for neutral thienoacenes21 (Fig. 10d) and obtained a very similar behavior to that found for our dications in consonance with the progressive aromatization of the initial quinoidal structure of Thn-TIPS (n = 1–3) and BDTh-TIPS after two-electron extraction.
Conclusions
In summary, a series of quinoidal benzo-thia-fused [n]thienoacenequinodimethanes Thn-TIPS (n = 1–3) and BDTh-TIPS were successfully synthesized. Their ground-state geometry and electronic structures were systematically studied by various experimental techniques (electronic absorption spectra, X-ray crystallographic analysis, VT NMR/ESR/SQUID and Raman spectroscopy) and DFT calculations. Our studies revealed that the transformation of the electronic structure from anti-aromatic in the bisindeno-[n]thienoacenes Sn-TIPS to pro-aromatic in this new series of quinoidal thienoacenes could be achieved by simply replacing the indene units by fused benzo-thia moieties. Consequently, these pro-aromatic compounds show larger diradical character, much longer singlet excited state lifetime, larger TPA cross section values and better redox amphotericity compared with their anti-aromatic counterparts Sn-TIPS. In addition, extension of molecular length leads to an increase of the diradical character, convergence of optical/electrochemical energy gap, decrease of singlet excited state lifetime and enhancement of TPA cross section. The observed modest singlet excited state lifetime, moderate TPA cross section and amphoteric redox behavior are all related to their pro-aromaticity and small to moderate diradical character. Spectroelectrochemical studies revealed their spectroscopic features of the neutral and charge species which can be correlated to the change of their electronic structures, and a linear λ (eV)–1/n relationship was obtained. Our detailed studies provided a fundamental understanding on how the pro-aromaticity and anti-aromaticity affect the ground-state electronic structure, diradical character and consequently the physical properties of quinoidal compounds.
Acknowledgements
The work in NUS was supported by MOE Tier 1 grant (R-143-000-573-112), Tier 2 grant (MOE2014-T2-1-080) and Tier 3 programme (MOE2014-T3-1-004). We thank Dr Bruno Donnadieu and Dr Tan Geok Kheng for X-ray crystallographic analysis. K.-W. H. acknowledges the financial support from KAUST. This work at Yonsei University was supported by the Global Frontier R&D Program on Center for Multiscale Energy System funded by the National Research Foundation under the Ministry of Science, ICT & Future, Korea (NRF-2014M3A6A7060583).
Notes and references
- See review articles:
(a) Z. Sun, Q. Ye, C. Chi and J. Wu, Chem. Soc. Rev., 2012, 41, 7857 RSC;
(b) A. Shimizu, Y. Hirao, T. Kubo, M. Nakano, E. Botek and B. Champagne, AIP Conf. Proc., 2012, 1504, 399 CrossRef CAS;
(c) J. Casado, R. P. Ortiz and J. T. López Navarrete, Chem. Soc. Rev., 2012, 41, 5672 RSC;
(d) Z. Sun, Z. Zeng and J. Wu, Chem.–Asian J., 2013, 8, 2894 CrossRef CAS PubMed;
(e) M. Abe, Chem. Rev., 2013, 113, 7011 CrossRef CAS PubMed;
(f) Z. Sun, Z. Zeng and J. Wu, Acc. Chem. Res., 2014, 47, 2582 CrossRef CAS PubMed;
(g) T. Kubo, Chem. Rec., 2015, 15, 218 CrossRef CAS PubMed;
(h)
M. Nakano, Excitation Energies and Properties of Open-Shell Singlet Molecules, Springer, 2014 Search PubMed.
-
(a) V. Hernández, S. Calvo Losada, J. Casado, H. Higuchi and J. T. López Navarrete, J. Phys. Chem. A, 2000, 104, 661 CrossRef;
(b) J. Casado, T. M. Pappenfus, K. R. Mann, E. Ortí, P. M. Viruela, B. Milián, V. Hernández and J. T. López Navarrete, ChemPhysChem, 2004, 5, 529 CrossRef CAS PubMed;
(c) T. Takahashi, K. I. Matsuoka, K. Takimiya, T. Otsubo and Y. Aso, J. Am. Chem. Soc., 2005, 127, 8928 CrossRef CAS PubMed;
(d) R. P. Ortiz, J. Casado, V. Hernandez, J. T. López Navarrete, P. M. Viruela, E. Orti, K. Takimiya and T. Otsubo, Angew. Chem., Int. Ed., 2007, 46, 9057 CrossRef CAS PubMed;
(e) R. P. Ortiz, J. Casado, S. R. González, V. Hernández, J. T. López Navarrete, P. M. Viruela, E. Ortí, K. Takamiya and T. Otsubo, Chem.–Eur. J., 2010, 16, 470 CrossRef PubMed;
(f) E. V. Canesi, D. Fazzi, L. Colella, C. Bertarelli and C. Castiglioni, J. Am. Chem. Soc., 2012, 134, 19070 CrossRef CAS PubMed.
-
(a) K. Takimiya, S. Shinamura, I. Osaka and E. Miyazaki, Adv. Mater., 2011, 23, 4347 CrossRef CAS PubMed;
(b) K. Yui, H. Ishida, Y. Aso, T. Otsubo, F. Ogura, A. Kawamoto and J. Tanaka, Bull. Chem. Soc. Jpn., 1989, 62, 1547 CrossRef CAS;
(c) Q. Wu, R. Li, W. Hong, H. Li, X. Gao and D. Zhu, Chem. Mater., 2011, 23, 3138 CrossRef CAS.
-
(a) K. Ohashi, T. Kubo, T. Masui, K. Yamamoto, K. Nakasuji, T. Takui, Y. Kai and I. Murata, J. Am. Chem. Soc., 1998, 120, 2018 CrossRef CAS;
(b) T. Kubo, M. Sakamoto, M. Akabane, Y. Fujiwara, K. Yamamoto, M. Akita, K. Inoue, T. Takui and K. Nakasuji, Angew. Chem., Int. Ed., 2004, 43, 6474 CrossRef CAS PubMed;
(c) T. Kubo, A. Shimizu, M. Sakamoto, M. Uruichi, K. Yakushi, M. Nakano, D. Shiomi, K. Sato, T. Takui, Y. Morita and K. Nakasuji, Angew. Chem., Int. Ed., 2005, 44, 6564 CrossRef CAS PubMed;
(d) A. Shimizu, M. Uruichi, K. Yakushi, H. Matsuzaki, H. Okamoto, M. Nakano, Y. Hirao, K. Matsumoto, H. Kurata and T. Kubo, Angew. Chem., Int. Ed., 2009, 48, 5482 CrossRef CAS PubMed;
(e) A. Shimizu, T. Kubo, M. Uruichi, K. Yakushi, M. Nakano, D. Shiomi, K. Sato, T. Takui, Y. Hirao, K. Matsumoto, H. Kurata, Y. Morita and K. Nakasuji, J. Am. Chem. Soc., 2010, 132, 14421 CrossRef CAS PubMed;
(f) A. Shimizu, Y. Hirao, K. Matsumoto, H. Kurata, T. Kubo, M. Uruichi and K. Yakushi, Chem. Commun., 2012, 48, 5629 RSC.
-
(a) R. Umeda, D. Hibi, K. Miki and Y. Tobe, Org. Lett., 2009, 11, 4104 CrossRef CAS PubMed;
(b) T. C. Wu, C. H. Chen, D. Hibi, A. Shimizu, Y. Tobe and Y. T. Wu, Angew. Chem., Int. Ed., 2010, 49, 7059 CrossRef CAS PubMed;
(c) Z. Sun, K.-W. Huang and J. Wu, Org. Lett., 2010, 12, 4690 CrossRef CAS PubMed;
(d) Z. Sun, K.-W. Huang and J. Wu, J. Am. Chem. Soc., 2011, 133, 11896 CrossRef CAS PubMed;
(e) Y. Li, W.-K. Heng, B. S. Lee, N. Aratani, J. L. Zafra, N. Bao, R. Lee, Y. M. Sung, Z. Sun, K.-W. Huang, R. D. Webster, J. T. López Navarrete, D. Kim, A. Osuka, J. Casado, J. Ding and J. Wu, J. Am. Chem. Soc., 2012, 134, 14913 CrossRef CAS PubMed;
(f) W. Zeng, M. Ishida, S. Lee, Y. Sung, Z. Zeng, Y. Ni, C. Chi, D.-H. Kim and J. Wu, Chem.–Eur. J., 2013, 19, 16814 CrossRef CAS PubMed;
(g) Z. Sun, S. Lee, K. Park, X. Zhu, W. Zhang, B. Zheng, P. Hu, Z. Zeng, S. Das, Y. Li, C. Chi, R. Li, K. Huang, J. Ding, D. Kim and J. Wu, J. Am. Chem. Soc., 2013, 135, 18229 CrossRef CAS PubMed;
(h) Z. Sun and J. Wu, J. Org. Chem., 2013, 78, 9032 CrossRef CAS PubMed;
(i) L. Shan, Z.-X. Liang, X.-M. Xu, Q. Tang and Q. Miao, Chem. Sci., 2013, 4, 3294 RSC;
(j) J. L. Zafra, R. C. González Cano, M. C. R. Delgado, Z. Sun, Y. Li, J. T. López Navarrete, J. Wu and J. Casado, J. Chem. Phys., 2014, 140, 054706 CrossRef PubMed;
(k) Y. Li, K.-W. Huang, Z. Sun, R. D. Webster, Z. Zeng, W. D. Zeng, C. Chi, K. Furukawa and J. Wu, Chem. Sci., 2014, 5, 1908 RSC;
(l) S. Das, S. Lee, M. Son, X. Zhu, W. Zhang, B. Zheng, P. Hu, Z. Zeng, Z. Sun, W. Zeng, R. W. Li, K. W. Huang, J. Ding, D. Kim and J. Wu, Chem.–Eur. J., 2014, 20, 11410 CrossRef CAS PubMed;
(m) Z. Sun, B. Zheng, P. Hu, K.-W. Huang and J. Wu, ChemPlusChem, 2014, 79, 1549 CrossRef CAS;
(n) X. Shi, S. Lee, M. Son, B. Zheng, J. Chang, L. Jing, K.-W. Huang, D. Kim and C. Chi, Chem. Commun., 2015, 51, 13138 RSC;
(o) P. Hu, S. Lee, T. S. Herng, N. Aratani, T. P. Gonçalves, Q. Qi, X. Shi, H. Yamada, K.-W. Huang, J. Ding, D. Kim and J. Wu, J. Am. Chem. Soc., 2016, 138, 1065–1077 CrossRef CAS PubMed.
-
(a) D. T. Chase, B. D. Rose, S. P. McClintock, L. N. Zakharov and M. M. Haley, Angew. Chem., Int. Ed., 2011, 50, 1127 CrossRef CAS PubMed;
(b) A. Shimizu and Y. Tobe, Angew. Chem., Int. Ed., 2011, 50, 6906 CrossRef CAS PubMed;
(c) A. G. Fix, P. E. Deal, C. L. Vonnegut, B. D. Rose, L. N. Zakharov and M. M. Haley, Org. Lett., 2013, 15, 1362 CrossRef CAS PubMed;
(d) B. D. Rose, C. L. Vonnegut, L. N. Zakharov and M. M. Haley, Org. Lett., 2013, 14, 2426 CrossRef PubMed;
(e) A. Shimizu, R. Kishi, M. Nakano, D. Shiomi, K. Sato, T. Takui, I. Hisaki, M. Miyata and Y. Tobe, Angew. Chem., Int. Ed., 2013, 52, 6076 CrossRef CAS PubMed;
(f) A. G. Fix, D. T. Chase and M. M. Haley, Top. Curr. Chem., 2014, 349, 159 CrossRef CAS PubMed;
(g) H. Miyoshi, S. Nobusue, A. Shimizu, I. Hisaki, M. Miyatab and Y. Tobe, Chem. Sci., 2014, 5, 163 RSC;
(h) B. S. Young, D. T. Chase, J. L. Marshall, C. L. Vonnegut, L. N. Zakharov and M. M. Haley, Chem. Sci., 2014, 5, 1008 RSC;
(i) D. Luo, S. Lee, B. Zheng, Z. Sun, W. Zeng, K.-W. Huang, K. Furukawa, D. Kim, R. D. Webster and J. Wu, Chem. Sci., 2014, 5, 4944 RSC.
-
(a) X. Zhu, H. Tsuji, H. Nakabayashi, S. Ohkoshi and E. Nakamura, J. Am. Chem. Soc., 2011, 133, 16342 CrossRef CAS PubMed;
(b) Z. Zeng, Y. M. Sung, N. Bao, D. Tan, R. Lee, J. L. Zafra, B. S. Lee, M. Ishida, J. Ding, J. T. López Navarrete, Y. Li, W. Zeng, D. Kim, K.-W. Huang, R. D. Webster, J. Casado and J. Wu, J. Am. Chem. Soc., 2012, 134, 14513 CrossRef CAS PubMed;
(c) Z. Zeng, M. Ishida, J. L. Zafra, X. Zhu, Y. M. Sung, N. Bao, R. D. Webster, B. S. Lee, R.-W. Li, W. Zeng, Y. Li, C. Chi, J. T. López Navarrete, J. Ding, J. Casado, D. Kim and J. Wu, J. Am. Chem. Soc., 2013, 135, 6363 CrossRef CAS PubMed;
(d) Z. Zeng, S. Lee, J. L. Zafra, M. Ishida, X. Zhu, Z. Sun, Y. Ni, R. D. Webster, R.-W. Li, J. T. López Navarrete, C. Chi, J. Ding, J. Casado, D. Kim and J. Wu, Angew. Chem., Int. Ed., 2013, 52, 8561 CrossRef CAS PubMed;
(e) Z. Zeng, S. Lee, J. L. Zafra, M. Ishida, N. Bao, R. D. Webster, J. T. López Navarrete, J. Ding, J. Casado, D. Kim and J. Wu, Chem. Sci., 2014, 5, 3072 RSC;
(f) Z. Zeng and J. Wu, Chem. Rec., 2015, 15, 322 CrossRef CAS PubMed;
(g) Z. Zeng, S. Lee, M. Son, K. Fukuda, P. M. Burrezo, X. Zhu, Q. Qi, R.-W. Li, J. T. López Navarrete, J. Ding, J. Casado, M. Nakano, D. Kim and J. Wu, J. Am. Chem. Soc., 2015, 137, 8572 CrossRef CAS PubMed.
- Z. Zeng, X. Shi, C. Chi, J. T. López Navarrete, J. Casado and J. Wu, Chem. Soc. Rev., 2015, 44, 6578 RSC.
-
(a) G. E. Rudebusch, A. G. Fix, H. A. Henthorn, C. L. Vonnegut, L. N. Zakharov and M. M. Haley, Chem. Sci., 2014, 5, 3627 RSC;
(b) X. Shi, P. M. Burrezo, S. Lee, W. Zhang, B. Zheng, G. Dai, J. Chang, J. T. López Navarrete, K.-W. Huang, D. Kim, J. Casado and C. Chi, Chem. Sci., 2014, 5, 4490 RSC.
-
(a) L. S. Fuller, B. Iddon and K. A. Smith, J. Chem. Soc., Perkin Trans. 1, 1997, 3465 RSC;
(b) D. T. Túng, D. T. Tuân, N. Rasool, A. Villinger, H. Reinke, C. Fischer and P. Langer, Adv. Synth. Catal., 2009, 351, 1595 CrossRef;
(c) T. H. Kwon, V. Armel, A. Nattestad, D. R. MacFarlane, U. Bach, S. J. Lind, K. C. Gordon, W. Tang, D. J. Jones and A. B. Holmes, J. Org. Chem., 2011, 76, 4088 CrossRef CAS PubMed.
- T. Okauchi, K. Kuramoto and M. Kitamura, Synlett, 2010, 19, 2891 CrossRef.
- S. Ota, S. Minami, K. Hirano, T. Satoh, Y. Ie, S. Seki, Y. Aso and M. Miura, RSC Adv., 2013, 3, 12356 RSC.
- S. D. Motta, F. Negri, D. Fazzi, C. Castiglioni and E. V. Canesi, J. Phys. Chem. Lett., 2010, 1, 3334 CrossRef.
-
(a) J. Anthony, Angew. Chem., Int. Ed., 2008, 47, 452 CrossRef CAS PubMed;
(b) T. Weil, T. Vosch, J. Hofkens, K. Peneva and K. Müllen, Angew. Chem., Int. Ed., 2010, 49, 9068 CrossRef CAS PubMed.
- B. Bleaney and K. D. Bowers, Proc. R. Soc. London, Ser. A, 1952, 214, 451 CrossRef CAS.
-
(a) Crystallographic data for Th1-TIPS: C48H66S3Si2. Mw: 795.36; triclinic; space group P
; a = 9.8336(6) Å, b = 14.2701(9) Å, c = 16.3902(10) Å, α = 83.637(2)°, β = 85.505(2)°, γ = 83.306(2)°; V = 2265.2(2) Å3; Z = 2; ρcalcd = 1.166 Mg m−3; R1 = 0.0591, wR2 = 0.1306 (I > 2σ(I)); R1 = 0.0888, wR2 = 0.1444 (all data). CCDC no. 1419444.†;
(b) Crystallographic
data for Th2-TIPS: C50H66S4Si2. Mw: 851.44; triclinic; space group P
; a = 7.4217(4) Å, b = 19.0953(11) Å, c = 19.1877(16) Å, α = 63.238(2)°, β = 89.851(3)°, γ = 79.162(2)°; V = 2374.2(3) Å3; Z = 2; ρcalcd = 1.191 Mg m−3; R1 = 0.0922, wR2 = 0.2629 (I > 2σ(I)); R1 = 0.1101, wR2 = 0.3472 (all data). CCDC no. 1419445.†;
(c) Crystallographic data for Th3-TIPS: C104H132S10Si4. Mw: 1815.05; triclinic; space group P
; a = 14.9964(4) Å, b = 18.3543(5) Å, c = 19.3746(5) Å, α = 96.6629(12)°, β = 99.0302(12)°, γ = 98.7364(11)°; V = 5151.9(2) Å3; Z = 2; ρcalcd = 1.170 Mg m−3; R1 = 0.0442, wR2 = 0.1035 (I > 2σ(I)); R1 = 0.0642, wR2 = 0.1145 (all data). CCDC no. 1419446.†;
(d) Crystallographic data for BDTh-TIPS: C54H68S4Si2. Mw: 901.50; monoclinic; space group P21/n; a = 6.9436(2) Å, b = 21.6735(6) Å, c = 33.9795(8) Å, α = 90°, β = 94.3960(10)°, γ = 90°; V = 5098.6(2) Å3; Z = 4; ρcalcd = 1.174 Mg m−3; R1 = 0.0495, wR2 = 0.1206 (I > 2σ(I)); R1 = 0.0680, wR2 = 0.1327 (all data). CCDC no. 1419447.†.
- J. Casado and J. T. López Navarrete, Chem. Rec., 2011, 11, 45 CrossRef CAS PubMed.
- R. Malavé Osuna, V. Hernández, J. T. López Navarrete, J. Aragó, P. Viruela, E. Ortí, Y. Suzuki, S. Yamaguchi, J. T. Henssler and A. Matzger, ChemPhysChem, 2009, 10, 3069 CrossRef PubMed.
-
(a) K. Ishii, Y. Hirose, H. Fujitsuka, O. Ito and N. Kobayashi, J. Am. Chem. Soc., 2001, 123, 702 CrossRef CAS PubMed;
(b) E. M. Giacobbe, G. Q. Mi, M. T. Colvin, B. Cohen, A. Ramana, A. M. Scott, S. Yeganeh, T. J. Marks, M. A. Ratner and M. R. Wasielewski, J. Am. Chem. Soc., 2009, 131, 3700 CrossRef CAS PubMed.
-
(a) M. Nakano, H. Nagao and K. Yamaguchi, Phys. Rev. A, 1997, 55, 1503 CrossRef CAS;
(b) M. Nakano, R. Kishi, T. Nitta, T. Kubo, K. Nakasuji, K. Kamada, K. Ohta, B. Champagne, E. Botek and K. Yamaguchi, J. Phys. Chem. A, 2005, 109, 885 CrossRef CAS PubMed;
(c) M. Nakano, T. Kubo, K. Kamada, K. Ohta, R. Kishi, S. Ohta, N. Nakagawa, H. Takahashi, S. Furukawa, Y. Morita, K. Nakasuji and K. Yamaguchi, Chem. Phys. Lett., 2006, 418, 142 CrossRef CAS;
(d) S. Ohta, M. Nakano, K. Kubo, K. Kamada, K. Ohta, R. Kishi, N. Nakagawa, B. Champagne, E. Botek, A. Takebe, S. Umezaki, M. Nate, H. Takahashi, S. Furukawa, Y. Morita, K. Nakasuji and K. Yamaguchi, J. Phys. Chem. A, 2007, 111, 3633 CrossRef CAS PubMed;
(e) M. Nakano, R. Kishi, A. Takebe, M. Nate, H. Takahashi, T. Kubo, K. Kamada, K. Ohta, B. Champagne and E. Botek, Comput. Lett., 2007, 3, 333 CrossRef CAS;
(f) M. Nakano, R. Kishi, S. Ohta, H. Takahashi, T. Kubo, K. Kamada, K. Ohta, E. Botek and B. Champagne, Phys. Rev. Lett., 2007, 99, 033001 CrossRef PubMed;
(g) M. Nakano, H. Nagai, H. Fukui, K. Yoneda, R. Kishi, H. Takahashi, A. Shimizu, T. Kubo, K. Kamada, K. Ohta, B. Champagne and E. Botek, Chem. Phys. Lett., 2008, 467, 120 CrossRef CAS;
(h) K. Yoneda, M. Nakano, Y. Inoue, T. Inui, K. Fukuda, Y. Shigeta, T. Kubo and B. Champagne, J. Phys. Chem. C, 2012, 116, 17787 CrossRef CAS;
(i) M. Nakano and B. Champagne, Theor. Chem. Acc., 2015, 134, 23 CrossRef.
- X. Zhang, A. Côté and A. J. Matzger, J. Am. Chem. Soc., 2005, 127, 10502 CrossRef CAS PubMed.
Footnote |
† Electronic supplementary information (ESI) available: Synthetic procedures and characterization data for all new compounds, general experimental method, additional spectroscopic data, DFT calculation details, crystallographic data. CCDC 1419444–1419447. For ESI and crystallographic data in CIF or other electronic format see DOI: 10.1039/c5sc04706d |
|
This journal is © The Royal Society of Chemistry 2016 |
Click here to see how this site uses Cookies. View our privacy policy here.