DOI:
10.1039/C6RA11409A
(Paper)
RSC Adv., 2016,
6, 85903-85916
Biodirected synthesis of palladium nanoparticles using Phoenix dactylifera leaves extract and their size dependent biomedical and catalytic applications†
Received
3rd May 2016
, Accepted 3rd August 2016
First published on 24th August 2016
Abstract
The emerging microbial resistance and increased water pollution are of serious concern around the globe. In order to cope with these problems, new strategies are needed to develop less toxic and more effective nanomaterials that could arrest the microbial growth and eliminate unwanted organic pollutants from water samples. In the present contribution, we report the green synthesis of palladium nanoparticles using the aqueous extract of Phoenix dactylifera leaves. The appearance of a characteristic surface plasmon resonance peak at 278 nm confirmed the synthesis of palladium nanoparticles (UV-vis spectroscopy). The formation of the PdNPs was optimized at different temperatures (30 °C, 60 °C and 90 °C) and varying amounts of leaf extract (5 mL, 10 mL and 20 mL) in order to control their size, shape and dispersion. Average particle sizes of 13, 5, and 21 nm were observed by using the leaf concentrations of 5, 10, and 20 mL, respectively (HRTEM, DLS), with a fixed amount of PdCl2 (0.003 M) at 60 °C. The PdNPs synthesized under the optimized conditions (10 mL extract + 60 °C + 0.003 M PdCl2) were spherical in shape, small sized and uniformly distributed (HRTEM). The biologically synthesized nanoparticles were tested for their size dependent biomedical and catalytic applications. The PdNPs synthesized under optimized conditions exhibited strong catalytic activity with a complete reduction of 4-nitrophenol to 4-aminophenol in only 2 min. These nanoparticles were highly active in scavenging DPPH free radicals. The optimized palladium nanoparticles also exhibited strong antibacterial efficiency against Pseudomonas aeruginosa 26 (±0.8 mm). This high activity of PdNPs may be due to their small size, high dispersion and surface capping phytochemicals.
1. Introduction
There is a continuous search to prepare more effective, less toxic and economic catalysts with unique structure, small size and high dispersion. All these features could be achieved by utilizing the renewable potential of nature. The plant kingdom presents the most versatile source of phytochemicals that could be successfully utilized for the preparation of nanoscale materials. The bio-synthetic method is a very simple and convenient way to prepare nanoparticles compared to chemical and physical methods. It is well-proven that nano-sized materials have high surface-to-volume ratio that can significantly enhance the interaction between reactants and catalysts.1 Thus nanocatalysis is an important alternative to the homogeneous catalysis to produce the same products with high reaction rates and high yields.
PdNPs are usually used as potent catalysts in a variety of organic reactions including Sonogashira coupling reactions, Stille, Mizoroki–Heck and Suzuki-cross coupling.2 Furthermore, Pd nanoparticles as a homogeneous or heterogeneous catalyst3 have also been applied in various scientific fields such as hydrogen sensor,4 hydrogen storage,5 automotive catalytic converter, chemo-optical transducers, chemical modifier in ETV-ICP-MS,6 optical limiting devices, and plasmonic wave guides.7 Various protocols have been introduced for the synthesis of PdNPs which include ion exchange,8 polyol method,9 vapor deposition, thermal decomposition,10,11 chemical and electrochemical reduction.12,13 However, all those procedures are associated with several drawbacks such as the use of toxic and expensive chemicals and high energy consumption and are therefore, not friendly both from economic and environmental point of view. The current strategies are focused to prepare the nano-catalyst of desired feature with an ecofriendly procedure that could minimize the cost and hazardous effects associated with chemical based synthesis procedures. In recent years, green syntheses of noble metal nanoparticles have received much attention in order to introduce environmentally benign technology in the field of nanoparticles synthesis. Additionally, this environmentally friendly procedure include a simple reaction setup, mild reaction conditions, use of nontoxic medium such as water and plant extract, cost effectiveness as well as greater efficiency for biomedical applications.14 Fungi,15–17 bacteria18–21 and plant extracts22–32 have been widely used for the synthesis of Ag, Au etc. nanoparticles.
Environmental toxic waste is a severe universal problem because of the rapid increase in population and industries. The waste bi-products eluting from the chemical industries contain a high concentration of toxic substances which have adverse effect on the living environment.33 Among these entire toxins, organic compounds and dyes are observed to be the most dangerous water pollutants.34,35 These dyes are harmful to all human, animals and aquatic life.36
4-Nitrophenol (4-NP) is a highly toxic easily water soluble compound which infuriates the eyes, skin, and respiratory zone and cause swelling. 4-Nitrophenol is the root of numerous medical situations such as mental confusion, unconsciousness, cyanosis and brutal abdominal pain followed by nausea.37 On the contrary 4-aminophenol (4-AP) is frequently applied in making of remedies like paracetamol and phenacetin etc. It is in addition useful as a corrosion inhibitor, photographic developer, and hair coloring mediator.38,39 To surmount the poisonous character correlated with 4-NP, it was changed to a functional substance such as 4-AP. Palladium nanoparticles were used to catalyze this reaction which were prepared by an easy and environmentally friendly route using Phoenix dactylifera leaf extract as a stabilizing and reducing agent. Noble metal nanoparticles are potent antibacterial agents possessing strong antibacterial activity with minor possibility of bacterial resistance to them.40,41 It has been reported that noble metal ions destroy the bacterial DNA, damage cell membrane, inhibit critically important enzymes and destroy bacteria by a process called respiratory burst mechanism.42,43 Furthermore, metal nanoparticles have the capability of producing variety of reactive oxygen species43 which destroy pathogenic microbes by a process called respiratory burst mechanism.
In the present work, palladium nanoparticles were prepared using the leaf extract of Phoenix dactylifera as reducing and capping agents. The leaf of Phoenix dactylifera have phenolic compounds i.e. catechin and dactyl lifric acid44 etc. which are responsible for the reduction and stabilization of nanoparticles. The nanoparticles synthesized at optimized conditions i.e. 10 mL extract concentration and 60 °C were spherical in shape, small sized and uniformly distributed. The prepared nanoparticles were evaluated for their catalytic and bio-medical applications. The result illustrated that palladium nanoparticles synthesized at optimized conditions have strong efficiency towards 4-nitrophenol reduction, bacterial inhibition and DPPH scavenging (Scheme 1).
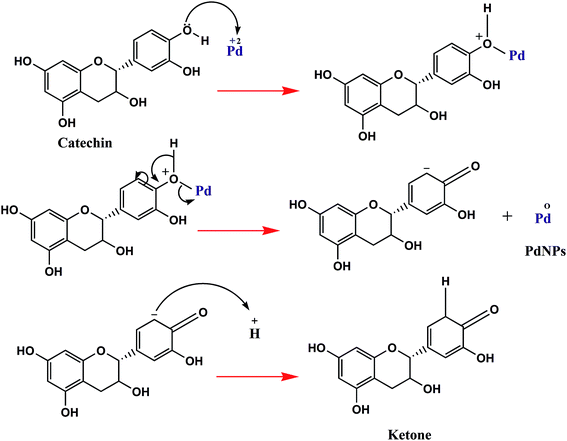 |
| Scheme 1 Reduction and stabilization of palladium nanoparticles in the presence of phenolic compounds of leaf extract. | |
2. Materials and method
2.1. Preparation of leaf extract
Leaves of Phoenix dactylifera were collected from lakki Marwat, Pakistan and thoroughly washed with distilled water to remove any dust particles. 10 g of the powdered plant material was extracted with 100 mL Milli-Q water. The suspended plant material in Milli-Q water was initially heated at 70 °C for 60 min. The as prepared aqueous extract was further stirred (500 rpm) for another 1 h. Finally, the extracted biomass was filtered through Whatman filter paper (Whatman filter paper no. 3). The clear supernatant obtained was stored at 4 °C for further use.
2.2. Synthesis of Pd nanoparticles using Phoenix dactylifera leaf extract
For the synthesis of palladium nanoparticles, 10 mL of Phoenix dactylifera leaf extract was added to 50 mL of 0.003 M aqueous solution of palladium chloride (PdCl2) in 100 mL beaker. The change in color took place from light brown to dark brown within 10 min. After the formation of Pd nanoparticles it was centrifuged and then dried in 6ES freeze drier. In another experiment, the PdNPs were also prepared by a chemical method using sodium borohydride as a reducing and citrate as capping agent.
2.3. Micro organisms
Bacterial specie, Pseudomonas aeruginosa was used in this assay. Strains were obtained from State Key Laboratory of Chemical Resource Engineering, Beijing University of Chemical Technology, Beijing 100029, Laboratory no. 1104, PR China, where these were identified and characterized. These strains were maintained on agar slants at 4 °C for antimicrobial test. Micro organisms were incubated overnight at 37 °C in Mueller-Hinton Broth (Oxoid) at pH 7.2.
2.3.1. Screening for antibacterial activity by agar well diffusion method. For determination of antibacterial activity of palladium nanoparticles, the agar well diffusion method was carried out.45 The bacterial strain was grown in nutrient broth at 37 °C for 24 h in an incubator. Using a sterile swab, the inocula of the respective bacteria was streaked on to the Muller Hinton agar (Oxoid) plates in order to make sure a uniform thick lawn of growth following incubation. Using sterile cork borer, wells of 6 mm in diameter were formed on to nutrient agar plates. On the other hand 1 mg palladium nanoparticles were dissolved in 1 mL distilled water and then wells were filled with 50 μL of the palladium nanoparticles and the plates were then kept to stay for 1 h at 25 °C. After that the plates were incubated at 37 °C for 24 h and the resultant diameters of zones of inhibition were measured carefully. Streptomycin was used as a standard.
2.3.2. Determination of minimum inhibitory concentration (MIC). In order to determined minimum inhibitory concentration (MIC) of the palladium nanoparticles, serial dilution method was used. With 1 mL of different concentrations of PdNPs in sterilized test tubes was mixed with 1 mL of bacterial solution (Pseudomonas aeruginosa) having turbidity of 0.5 Mcfarland turbidity standard. After mixing, these test tubes were placed in an incubator at 37 °C for 24 h. A test tube having only growth media and bacteria was used as a control. 1 mg mL−1 to 0.125 mg mL−1 concentrations of PdNPs were used in these tests. The minimum concentration of the compound, which inhibited the growth of the respective organism, was considered as MIC. The assay was carried out in triplicate.
2.3.3. Assessment of the cell constituents' release. The disruption of bacterial cell membrane and their cellular constituents release into the media was evaluated by a method as described previously.46 In short, working cultures of the tested Pseudomonas aeruginosa were collected by centrifugation (10 min, at 5000g per min), washed thrice with 0.1 M PBS (pH 7.0), and the pallet obtained was re-suspended in 50 mL 0.1 M PBS. The resulted bacterial suspension was treated with different concentrations (2 × MIC, MIC, and control) of palladium nanoparticles and incubated at 37 °C for 2 h. After 2 h of incubation, the treated bacterial cells were harvested and the supernatant was assessed for the determination of cell constituents released using spectrophotometer (260 nm).
2.4. Hemolytic activity assay
The hemolytic property of palladium nanoparticles was evaluated by measuring the hemoglobin released from the red blood cells (RBCs) with the treatment of PdNPs. The blood was collected from male Wistar albino mouse in a sterile Lithium Heparin vacutainers. The tube was followed to centrifuge at 1500 rpm for 10 minutes. The supernatant was carefully removed and the pellet was washed three times with phosphate-buffered saline (PBS) at pH 7.4. PdNPs in different concentrations (12.5, 25, 50, 75, 100 μg and 125 μg) in PBS were put into separate tubes and the cells (5% v/v) in PBS were added to each tube and the final volume was made up to 1 mL. RBCs in PBS and 1% Triton X-100 solution were taken as negative and positive controls respectively. These reaction mixtures were incubated in shaking incubator at 37 °C for 1 hour with gentle shaking. Then the tubes were centrifuged at 1500 rpm for 10 minutes and the supernatant was monitored at 540 nm against their blank. The percentage of hemolytic activity was determined as previously reported.47
2.5. DPPH free radical scavenging assay
DPPH is a stable radical and its reduction requires sever conditions. We have followed the procedure of Chang et al.48 with little modification to examine the DPPH radical scavenging activity of palladium nanoparticles. 0.5 mL of 1.0 mM solution of DPPH was stirred with different concentrations of palladium nanoparticles ranging from 31–1000 μg mL−1 and kept for 30 min in dark. UV 1100 spectro-photometer (MAPADA instruments) was used to observe the absorbance of samples at 517 nm after incubation. Methanol was used as blank. DPPH methanol reagent devoid of sample was utilized as control and vitamin C was employed as a standard. The percentage of inhibition was estimated by the following method.
Percentage of inhibition = absorbancecontrol − absorbancetest/absorbancecontrol |
The study was conducted following a protocol approved by the institutional animal care and uses committee of the Beijing University of Chemical Technology (Animal Care and Use Committee) and performed in accordance with the Guide for Care and Use of Laboratory Animals.
2.6. Chemo catalytic conversion of 4-nitrophenol (4-NP) to 4-aminophenol (4-AP)
To test the catalytic activity of PdNPs, reduction of 4-NP to 4-AP was observed at different time intervals. Briefly 1.4 mL of water, 1 mL of 0.03 M NaBH4 solution and 0.3 mL of 2 mM solution of 4-NP was added in quartz cuvette. 5 mg of biogenic PdNPs was added to this reaction amalgam and UV-vis spectra were taken by using Shimadzo UV-vis-2400 spectrophotometer.
2.7. Characterization
The biogenic Pd nanoparticles were studied initially by UV-visible spectrophotometer (Shimadzo UV-2400). The wide angle X-ray diffraction (XRD) measurements were carried out on a Rigaku D/Max 2500 VBZ+/PC diffractometer. High resolution transmission electron microscopy (HRTEM) and SAED of PdNPs was analyzed on a JEM-3010 microscope. Infra red (IR) spectrum of the extract was obtained using an ABB MB3000 spectrophotometer. The element distribution maps and EDX were obtained using a Hitachi S-4700 scanning electron microscope.
3. Result and discussion
3.1. UV-visible spectroscopy analysis
The PdNPs formation was first confirmed by UV-visible spectroscopy. Every element has free electrons, which give rise to surface plasmon resonance (SPR) peak.49 It is well known that the surface plasmon resonance peak is dependent on the size and shape of the nanoparticles formed.50 The peak appeared at 278 nm clearly indicates the formation of PdNPs. The SPR peak of PdNPs was studied at different reaction temperatures i.e. 30 °C, 60 °C and 100 °C illustrated in (Fig. 1A). Low intense and broad SPR peak for PdNPs was appeared at 30 °C. It may be due to requirement of high energy for excitation of valence electrons. As a result small amount, large sized and partially aggregated palladium nanoparticles were obtained which is in close agreement with HRTEM (Fig. 4A). The palladium nanoparticles synthesized at 60 °C have a sharp and highly intense peak which may be due to excitation of valence electrons and thus an increase in the reaction rate.51 This high intensity of peak is also due to tiny particles.52 The palladium nanoparticles prepared at 100 °C showed red shift with broad and low intense SPR peak, indicating the large size and low amount of nanoparticles. It may occur due to decomposition of organic compounds because most of the organic compounds are unstable at higher temperature.
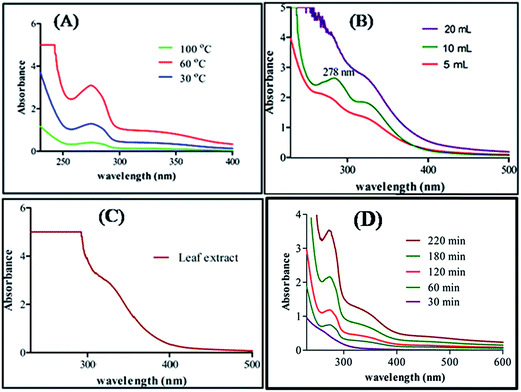 |
| Fig. 1 UV-visible spectroscopic analysis of palladium nanoparticles synthesized at (A) different temperatures i.e. 30, 60, and 100 °C (B) different leaf extract concentrations i.e. 5, 10 and 20 mL (C) Phoenix dactylifera leaf extract at 100 °C and (D) time dependent evolution of UV-vis spectra of green synthesized PdNPs at 60 °C. | |
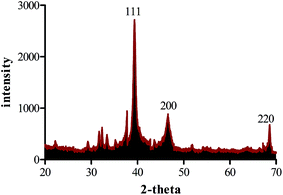 |
| Fig. 2 XRD analysis of green synthesized palladium nanoparticles at optimized conditions. | |
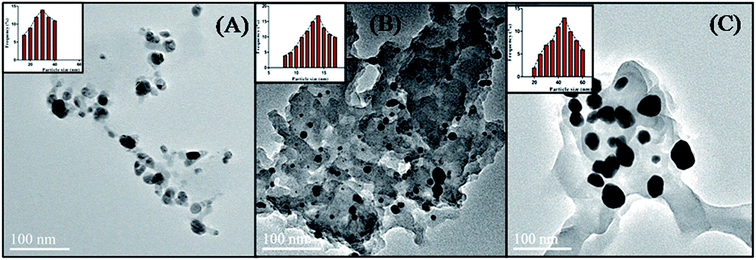 |
| Fig. 3 HRTEM images of palladium nanoparticles synthesized at different temperatures (A) 30 °C, (B) 60 °C, (C) 100 °C and their corresponding DLS. | |
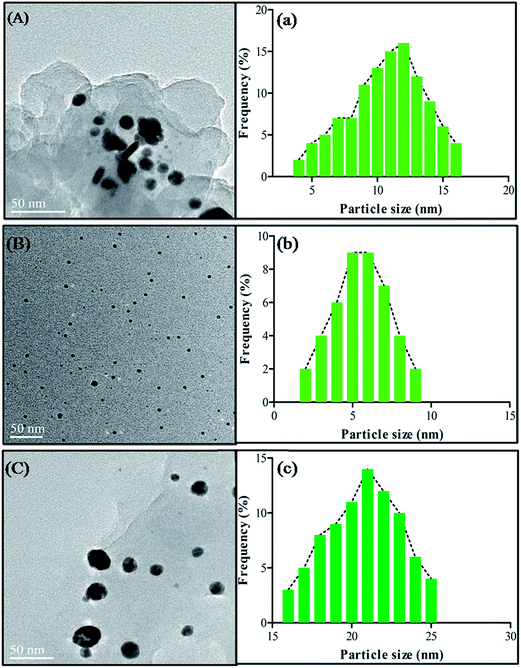 |
| Fig. 4 HRTEM images of palladium nanoparticles synthesized at different extract concentrations (A) 5 mL, (B) 10 mL, (C) 20 mL and their corresponding DLS analysis (a) 5 mL, (b) 10 mL, (c) 20 mL. | |
(Fig. 1B) shows the UV-visible spectra of aqueous solution of palladium nanoparticles synthesized at different Phoenix dactylifera leaf extract concentrations i.e. 5, 10 and 20 mL with palladium precursor (0.003 M of PdCl2). It was observed that the intensities and specific position of the SPR peaks of palladium nanoparticles changed with change in concentrations of the leaf extract. The palladium nanoparticles showed an absorption peak at 280 nm when 5 mL leaves extract concentration was used as a reducing agent. A low intensity and high broadness of the absorption peak for palladium nanoparticles was observed at this concentration of the leaf extract, representing the formation of small amount of nanoparticles. However when 10 mL of the leaf extract concentration was used as a reducing and stabilizing agent, the SPR peak for palladium nanoparticles moved towards blue shift from 280 to 278 nm with the subsequent increase in peak intensity suggesting the possible decrease in nanoparticle size. This phenomenon means that at 10 mL extract concentration; the rate of formation of palladium nanoparticles from palladium ions was increased due to availability of high content of organic moiety which works as a reducing and capping agents. Further increase in leaf extracts concentration i.e. 20 mL, the absorption peak of nanoparticles moved towards red shift which has low intensity and high broadness representing the possible increase in particle size. It may be due to additional reaction between the surface molecules and the molecules in the solution.53 These phyto-constituents play a vital role in the formation of dispersed and small sized nanoparticles as reported previously.54,55
3.2. FTIR analysis
The FTIR analysis was used to determine the possible phytochemicals present in the Phoenix dactylifera leaf extract which work as a reducing source and stabilizing agent. (ESI Fig. S1†) demonstrates the FTIR spectra of Phoenix dactylifera leaf extract and plant mediated palladium nanoparticles. The FTIR spectrum of aqueous extract of Phoenix dactylifera leaf extract shows three major peaks at 3433, 1633 and 1050 cm−1. The broad peak at 3433 cm−1 is associated with OH stretching vibration of phenolic compounds. The two intense peaks at 1633 and 1050 cm−1 are associated with conjugated C
C and C–N stretching amine respectively. The FTIR spectrum of plant extract mediated PdNPs showed a decrease in the peak intensities of the said functional groups suggesting the possible association of these groups in the synthesis of nanoparticles. On the basis of this analysis we can say that the synthesized PdNPs is stabilized by various functional groups present in the aqueous extract of plant, such as proteins, flavonoids and seponins etc.
3.3. XRD analysis
The crystalline structure of phyto-synthesized palladium nanoparticles was determined by using powder XRD technique. The XRD pattern of palladium nanoparticles is shown in (Fig. 2). The angle range was taken from 20–70°. Results showed that three prominent Bragg peaks were obtained at position 40°, 46.7° and 68° that is corresponding to (111), (200) and (220) lattice planes of f.c.c crystal structure for PdNPs. All the other peaks may be due to the organic moiety.56 Similar diffraction pattern for palladium nanoparticles have also been reported previously.57 Result illustrated that the most significant peak was obtained at 2θ = 40° which is originated due to f.c.c crystal structure of palladium nanoparticles. It is clear from the above result that the most dominant orientation of green synthesized palladium nanoparticles must be the (111) crystal plane. It is because due to its high atomic concentration, the (111) plane is considered to be the highly reactive facet. The average particle size of palladium nanoparticles synthesized at optimized condition is calculated using Debye–Scherrer eqn (1) and the particle size was found to be 5 nm. This result is in close agreement with the particle size determined by HRTEM. |
 | (1) |
where, D and β represent the particle size and full-width half maxima of the diffraction peak respectively. Theta (θ) represents the Bragg angle while K is the Scherrer constant with a value range from 0.9 to 1 respectively. The λ is the wavelength of the X-ray source.
3.4. High resolution transmission electron microscopy (HRTEM) studies
The size, shape and dispersion of bio-synthesized palladium nanoparticles were observed by HRTEM analysis at various temperatures i.e. 30 °C, 60 °C and 100 °C. (Fig. 3A–C) represents the HRTEM images of palladium nanoparticles synthesized at different temperatures using 0.003 mM PdCl2 and 10 mL extract concentration. It is clear from the HRTEM image that PdNPs synthesized at 60 °C (10 mL extract + 2 mM PdCl2) was extremely dispersed, sphere-shaped, small and uniform sized (average size 2–5 nm). On the other hand, the PdNPs prepared at 30 and 100 °C (Fig. 3) were comparatively very large in size, irregular in shape and have very low concentration. It is due to the fact that a very low temperature (30 °C) provided low energy which is insufficient for the surface plasmon resonance peak of palladium nanoparticles. The nanoparticles prepared at 100 °C also have large size and low dispersion indicating low reducing and stabilizing efficiency of leaf extract. It may be occurs due to decomposition of organic moiety of leaf extract at high temperature. The above obtained results are in close agreement with UV-visible spectroscopy.
Fig. 4 illustrates the HRTEM images of palladium nanoparticles synthesized using different leaf extract concentrations (5, 10, and 20 mL). It is apparent from the HRTEM image (Fig. 4A) that palladium nanoparticles prepared at low extract concentration i.e. 5 mL were irregular shapes and large sized with an average particle size of 13 nm. It is also observed from HRTEM images that palladium nanoparticles prepared at 5 mL extract concentration have slight aggregation with each other (agreement with low SPR intensity). Low amount of the leaf extract reduce the palladium ions into nanoparticles, but do not stabilized the majority of the nanoparticles from aggregation due to the lack of organic moiety to act as stabilizing agents.58 Insufficient capping of the palladium nanoparticles results in aggregation among the developing nuclei, which leads to larger size of palladium. In addition, at 5 mL extract concentration, only smaller amount of stable palladium nuclei are formed. If the production of metal nuclei is low, the resulting particles will be bigger in size.59 The palladium nanoparticles prepared at 10 mL extract concentration were small, spherical and highly dispersed without any aggregation with an average particle size of 5 nm. The nanoparticles produced at 10 mL extract concentration have sufficient reducing and stabilizing agents, which properly reduce and stabilize the palladium ions into palladium nanoparticles. Alternatively, when the concentration of leaf extract was further increased up to 20 mL, the palladium nanoparticles prepared were relatively bigger in size and of different shapes (Fig. 4C). It may be expected that the high concentration of the leaf extract, giving rise to larger particles because of an additional interaction between the surface stabilizing molecules and secondary reduction at preformed nuclei's surface.
3.5. SEM analysis of PdNPs synthesized at optimized conditions
The size, shape and dispersion of Pd nanoparticles synthesized at 10 mL extract concentration were confirmed by scanning electron microscopy (SEM) inset in (Fig. 5A). It is clear from SEM analysis that the PdNPs are present in nano form. The white spherical bodies represent the Pd nanoparticles which are highly dispersed and small sized. Most of the PdNPs are of spherical shape with uniform distribution. Fig. 5B–D shows the elemental distribution maps of carbon, Pd and oxygen respectively using optimized conditions. It is clear that each element is uniformly distributed throughout the material.
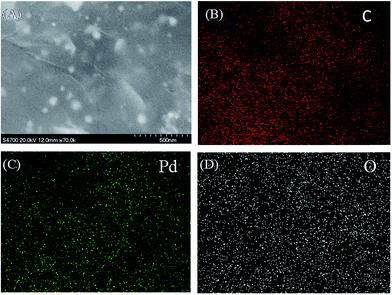 |
| Fig. 5 (A) SEM analysis of palladium nanoparticles synthesized at optimized conditions (10 mL extract concentration + 60 °C temperature + 0.003 M PdCl2) and (B, C and D) represents their corresponding mapping of carbon, palladium nanoparticles and oxygen respectively. | |
3.6. EDX and SAED analysis of PdNPs synthesized at optimized conditions
The f.c.c crystalline nature of green prepared palladium nanoparticles was also confirmed by selected area electron diffraction (SAED). Three well resolved circular rings are indicated by SAED pattern (ESI Fig. S2A†) which confirm the (111), (200), and (220) Bragg's reflection planes of XRD pattern. In addition, the circular fringes also indicated the highly crystalline structure of palladium nanoparticles.
The elemental composition of the green synthesized PdNPs was determined by EDX analysis (ESI Fig. S2B†). It is clear from EDX analysis that the strong peak appeared in the Pd region which confirmed the formation of PdNPs. The peaks for carbon and oxygen were also observed which means that they are also present on the surface of PdNPs and confirmed the capping effort of organic moiety.
3.7. Particle size distribution and stability
The particle size of biologically synthesized palladium nanoparticles (PdNPs) was determined using dispersive light scattering (DLS) analysis. The average particle size of green synthesized PdNPs at 5 mL extract concentration was found to be 13 nm as shown in (Fig. 4a), though the particle size distribution ranged between 5 to 18 nm. The maximum number of PdNPs was observed in the range of 10 to 14 nm. The PdNPs synthesized at 10 mL extract concentration have the size range in between 2 to 8 nm as clear from the size histogram (Fig. 4b). The average size of PdNPs synthesized at 10 mL extract concentration was found to be 5 nm. The PdNPs synthesized at higher concentration i.e. 20 mL have larger size with maximum number of nanoparticles in the range of 13 to 26 nm (Fig. 4c). This result is in close agreement with the UV-visible spectroscopy and HRTEM analysis. However up to some extent, larger size of nanoparticle was observed which may be due to the hydrated organic moiety and solvation effects. Additionally, the difference in nanoparticle sizes determined by other methods may also be attributed to different physical principles or detection methods.
The stability of bio-directed PdNPs was determined using zeta potential analysis. It is mostly used for the dispersion stability of nanoparticles. The zeta potential of PdNPs prepared at different leaf extract concentrations is shown in (ESI Fig. S3A and B†). The PdNPs prepared at lower extract concentration i.e. 5 mL have lower zeta potential value and at higher extract concentration i.e. 10 mL have higher zeta potential values. When we increased the leaf extract concentration from 5 to 10 mL for the synthesis of nanoparticles, the zeta potential values also increased from −25 to −30 mV indicating an increase in the stability of the synthesized PdNPs. Higher value of zeta potential show more stable dispersion of nanoparticles. The zeta potential of PdNPs show negative value which means that the nanoparticles are capped with negatively charged phytoconstituents which make repulsion among the nanoparticles (produce dispersion among the particles) and thus increasing the stability.60 This electrostatic repulsion among the negatively charged PdNPs prevents the aggregation, which might be necessary for their durable stability.
3.8. Antibacterial activity
The increase in new infectious diseases and resistance of most of the bacteria to the available drug is a serious worldwide problem. In order to overcome these problems it is the need of the day to develop new therapeutic agents which are safer and more active to inhibit these microbial pathogens. For this purpose the leaf extract of highly active medicinal plant, Phoenix dactylifera was used for the synthesis of palladium nanoparticles to enhance its bio-activities. The size dependent antibacterial activity of palladium nanoparticles were tested against Pseudomonas aeruginosa and the results showed variation with respect to the nanoparticle size (Table 1). The palladium nanoparticles prepared at optimized condition (10 mL extract concentration + 60 °C temperature + 0.003 M PdCl2) exhibited significant antibacterial activity against Pseudomonas aeruginosa with diameter of zone of inhibition 26 (±0.8 mm), which was higher than chemically synthesized PdNPs (18 ± 1.6 mm) (Fig. 6) (Table 1). However, palladium nanoparticles prepared at 5 and 20 mL extract concentrations were moderately active against Pseudomonas aeruginosa with zone of inhibition 20 (±0.3 mm) and 21 (±0.6 mm) respectively. The strong antibacterial activity of palladium nanoparticles synthesized at optimized condition may be attributed to their small size and spherical morphology. Smaller and spherical nanoparticles provide large specific surface area and are therefore more active than their larger particle size and irregular shape. The antibacterial activity of metal nanoparticles is mainly due to interaction of metal ions with the cellular contents of the bacteria. There are several proposed mechanisms has been suggested for the inactivation of bacteria through metal nanoparticles such as (i) metal ions interact with the thiol group of bacterial enzymes and denature them, (ii) inhibition of bacteria through respiratory mechanism, (iii) the metal ions attack on bacterial DNA and destroy them, (iv) cause reduction thus disrupt cellular transport system, (v) physical contact of metal nanoparticles disrupt bacterial cell membrane and (vi) production of highly reactive superoxide and hydroxide radicals from metal nanoparticles.61 Prior research indicates that control release of metals from nanoparticles induces the formation of hydroxide and superoxide radicals in pathogenic bacteria. When the concentration of these radicals rises above the scavenging capacity of bacterial cell, they cause damage to the cell. The most important outcome of this study is that palladium nanoparticles exhibited strong antibacterial activity against Pseudomonas aeruginosa which have strong defensive system against several antibiotics including streptomycin. This bacterium is generally isolated from the burn wounds, and the palladium nanoparticles could be successfully applied to inhibit this bacterium.
Table 1 Zone of inhibition of palladium nanoparticles synthesized at different extract concentration against Pseudomonas aeruginosaa
Different sizes of green PdNPs |
Inhibition zone of green PdNPs |
*PdNPs (chem.) = chemical synthesized PdNPs. |
13 nm (5 mL extract) |
20 (±0.3 mm) |
5 nm (10 mL extract) |
26 (±0.8 mm) |
21 nm (20 mL extract) |
21 (±0.6 mm) |
*PdNPs (chem.) |
18 (±1.6 mm) |
Plant extract |
08 (±0.8 mm) |
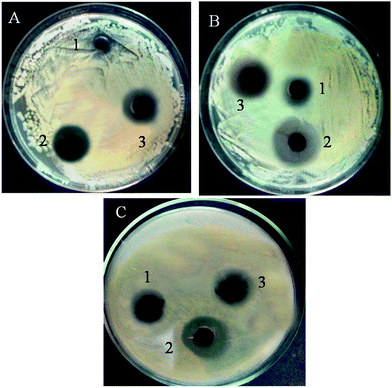 |
| Fig. 6 Antibacterial activity of green synthesized palladium nanoparticles synthesized at different leaf extract concentrations (A) 5 mL, (B) 10 mL and (C) 20 mL against Pseudomonas aeruginosa, (1), (2) and (3) represent the antibacterial activity of plant extract, palladium nanoparticles prepared by green method and palladium nanoparticles prepared by chemical method respectively. | |
3.8.1. Minimum inhibitory concentration. To determine quantitatively the antibacterial activity of PdNPs we applied the method of minimum inhibitory concentration (MIC). The MIC is the minimum concentration of antibacterial material that inhibits the growth of a pathogenic microorganism in artificial media after a specific incubation time. Similarly diluted suspensions of PdNPs were incubated with Pseudomonas aeruginosa solutions and the bacterial growth was observed. After 24 h of incubation, we found that the MIC was 0.25 mg mL−1 which is shown in (Table 2).
Table 2 Minimum inhibitory concentration (MIC) of PdNPs against Pseudomonas aeruginosa in 24 h
Bacteria |
PdNPs (mg mL−1) |
1 |
0.5 |
0.25 |
0.125 |
Pseudomonas aeruginosa |
− |
− |
− |
+ |
3.9. Cells constituents' release
Additionally, we measured the cell constituents release from the bacterium Pseudomonas aeruginosa in order to prove that the palladium nanoparticles broke the cell membrane of the bacterium and the cell contents of bacterium released into their media. The cell constituents' release from the cell was evaluated by measuring the absorbance of the supernatants of Pseudomonas aeruginosa at 260 nm. Table 3 indicates the results of cells constituents' release of the Pseudomonas aeruginosa in the presence of palladium nanoparticles at different concentrations of 0, MIC, and 2 × MIC for 2 h. The results illustrated that the cell constituents released is directly proportional to the concentration of palladium nanoparticles as compared to the control. The most efficient cell constituents' loss was observed at concentration of 2 × MIC of the palladium nanoparticles, showing an absorbance of 0.768. The result indicated that the damage of the cell membrane and cytoplasm of the bacterium in the presence of palladium nanoparticles was irreversible.
Table 3 Effect of palladium nanoparticles on the cell contents release from bacterial strain
Bacterium |
PdNPs concentrations |
Cell constituents released (OD260 nm)a |
The experiments in triplicates and the results are presented as a mean ± standard deviation. OD260 nm is optical density at 260 nm. Means within the same strain. |
Pseudomonas aeruginosa |
0 |
0.081 ± 0.003b |
MIC |
0.550 ± 0.001 |
2 × MIC |
0.768 ± 0.016 |
3.10. Antioxidant activity
Those compounds and materials which have higher reducing power have a strong antiradical activity. In this study the 1,1-diphenyl-2-picrylhydrazyl (DPPH) was taken as experimental substrate. DPPH is a stable radical and mostly resist to the majority of compounds. DPPH gives strong absorption at 517 nm due to the presence of free radical or odd electron. In order to get stability, the DPPH takes electron from electron donor compounds and the absorption maximum at 517 nm is decreased which results in decolorization of the solution. In this assay the palladium nanoparticles prepared at three different extract concentrations were applied to investigate the DPPH free radical inhibition assay. The strong reducing agent vitamin C was used as a standard. In this assay seven different concentrations of nanoparticles were used and the efficiency of nanoparticles was found to increase with increasing the dose of palladium nanoparticles. The results are correlated with the previously reported platinum, selenium, silver and gold nanoparticles.62,63
The results illustrated strong inhibition of DPPH (85%) by palladium nanoparticles synthesized at 10 mL leaf extract concentration as compared to other palladium nanoparticles prepared at 5 mL (72%) and 20 mL (75%) extract concentrations as shown in (Fig. 7). It may be due to spherical shape, high dispersion and small size of palladium nanoparticles prepared at 10 mL extract concentration as clear from HRTEM analysis. The small size and spherical shaped nanoparticles provide a large surface area and more active sites which enhanced the efficiency of nanoparticles. Antiradicals control the activity of free radicals hence they will increase the efficiency of the body's immune system which allowing the system to compete with pathogenic microbes that attack the body.
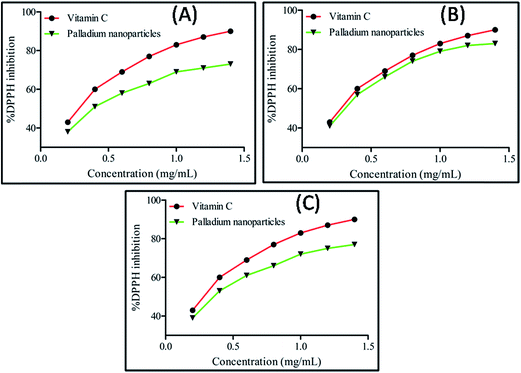 |
| Fig. 7 Antioxidant activity of palladium nanoparticles synthesized at different extract concentrations (A) 5 mL, (B) 10 mL and (C) 20 mL. | |
3.11. Hemolytic activity
Hemolytic activity of PdNPs was performed in order to evaluate the biocompatibility on normal cells, especially RBCs. The hemolytic assessment was performed using different concentrations of PdNPs (12.5, 25, 50, 75, 100 and 125 μg) on RBCs. It was found that PdNPs have no hemolytic activity against RBCs on different tested concentrations as it showed nearly equal hemolytic activity to that of negative control (Table 4). It may be due to the presence of capping agents of different phenolic compounds of leaf of Phoenix dactylifera. The presence of this active organic moiety increased the plasma antiradical efficiency and a reduction in the defenselessness of erythrocyte membranes to oxidation and thus greatly enhanced the resistance to hemolysis.64
Table 4 Hemolytic property of palladium nanoparticles synthesized at optimized condition (10 mL extract concentration + 60 °C temperature + 0.003 M PdCl2) on RBCs at different concentrationsa
Sample (n = 3) |
Hemolytic activity (%) (OD540 nm) |
Experiments are in triplicates and the results are presented as a mean ± standard deviation. OD540 nm is optical density at 540 nm. |
Control |
1.21 ± 0.18 |
1% Triton X-100 |
103 ± 0.0 |
PdNPs (12.5 μg) |
1.25 ± 0.11 |
PdNPs (25 μg) |
1.28 ± 0.15 |
PdNPs (50 μg) |
1.30 ± 0.0 |
PdNPs (75 μg) |
1.31 ± 0.12 |
PdNPs (100 μg) |
1.33 ± 0.11 |
PdNPs (125 μg) |
1.35 ± 0.13 |
3.12. Catalytic conversion of 4-NP to 4-AP
In this reaction sodium borohydride (NaBH4) was used as reducing agent to monitor the conversion of 4-NP and the competence of Pd nanoparticles. Due to kinetic barrier there is huge potential difference between acceptor and donor molecules which reduces the opportunity of the supposed reaction. In order to overcome the kinetic barrier, Pd nanoparticles performed a precise task by assisting electron conduction from the electron donator BH4− to electron acceptor 4-nitrophenolate ion. 4-Nitrophenolate ion was observed as an intermediate during the conversion of 4-nitrophenol to 4-aminophenol (Scheme 2). The transport of electron from BH4− to 4-nitrophenolate ion happens when they make contact with the Pd nanoparticles. The activation energy of the reaction was lowered by using Pd nanoparticles as a catalyst. In alkaline medium UV-visible spectroscopic results shown the peak at 317 nm for 4-nitrophenol which shifted towards red shift at 400 nm in the incidence of NaBH4 due to creation of 4-nitrophenolate ion.65 By tracking the difference in the spectra of 4-nitrophenolate ion at 400 nm the advancement of reaction can be observed by UV-visible spectroscopy at several time zones. On addition of Pd nanoparticles there was a quick decrease of adsorption peak at 400 nm, while the formation of adsorption peak at 292 nm shows the confirmation of 4-AP which is obvious from (Fig. 8). In start rapid decrease was observed in the peak at 400 nm due to accessibility of whole surface region of Pd nanoparticles but with the passage of time the reaction was slow down because nearly the entire available region of Pd nanoparticles was covered by reactants. The speed of this reaction entirely depends upon the available surface area of Pd nanoparticles. We examined the effect of particles size and morphology of Pd nanoparticles on the chemocatalytic reduction of 4-NP to 4-AP. The chemocatalytic activity of Pd nanoparticles synthesized at different extract concentrations i.e. 5 mL, 10 mL and 20 mL was studied. The results showed that Pd nanoparticles synthesized at 10 mL have strong chemocatalytic activity and reduced 4-NP only in 2 min. On the other hand the Pd nanoparticles synthesized at lower extract concentration have weak chemocatalytic activity. It is due to the fact that Pd nanoparticles prepared at 10 mL extract concentration have spherical shape and small size with high dispersion. Commonly with decreasing the size of nanoparticles, the chemocatalytic reduction of 4-NP to 4-AP is increased because small spherical particles provide large specific surface area which enhances the chemocatalytic reduction ability of nanoparticles. In other words, we can say that the chemocatalytic reduction of 4-NP to 4-AP is directly proportional to the spherical and smaller size of nanoparticles.
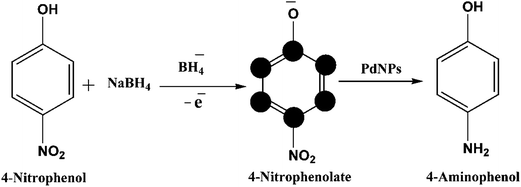 |
| Scheme 2 Mechanism of the formation of 4-AP from 4-NP through intermediate 4-nitrophenolate ion in the presence of NaBH4 as a reducing agent and PdNPs as a catalyst. | |
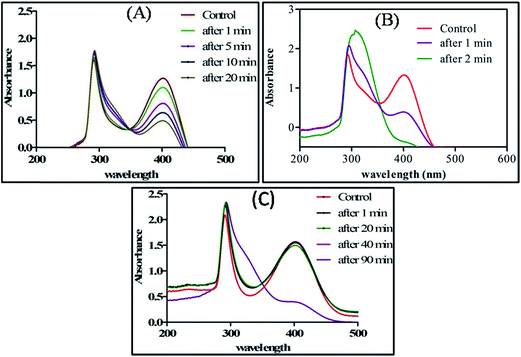 |
| Fig. 8 Chemocatalytic conversion of 4-NP to 4-AP in the presence of palladium nanoparticles synthesized at different extract concentrations (A) 5 mL, (B) 10 mL and (C) 20 mL. | |
4. Conclusion
Water purification and microbial contamination is a severe worldwide problem and most of the usual used methods have a number of limitations i.e. costly and environmentally unsafe. Therefore, there is an intense need to develop new techniques and materials to overcome the problem of water purification. In this work, a green procedure was introduced to modify the conventional methods of synthesis as this method is cost-effective and non-hazardous. The leaf extract of Phoenix dactylifera was used as a reducing and stabilizing agent for the synthesis of PdNPs. The synthesized PdNPs were optimized at different temperatures (30 °C, 60 °C and 90 °C) and variant leaf extract concentrations (5 mL, 10 mL and 20 mL) and it was observed that 10 mL extract concentration and 60 °C temperature are more favorable conditions to obtain small and uniform size of PdNPs. The synthesized nanoparticles at different extract concentrations were evaluated for catalytic reduction of 4-NP to 4-AP and inactivation of Pseudomonas aeruginosa. The results demonstrated that the nanoparticles synthesized under optimized conditions have high catalytic and biological activities. These high activities of PdNPs may be attributed to their small size and uniform distribution. Further research is required to examine the other applications of PdNPs.
Acknowledgements
The authors are thankful to China Scholarship Council (No. 2013GXZ031), National Natural Science of Foundation of China (Grant No. 21271017) and the National Science and Technology Supporting Plan of the Twelth five-year (No. 2014BAE12B0101), the Fundamental Research Funds for the Central Universities (No. YS1406).
References
- S. Wei, Z. Dong, Z. Ma, J. Sun and J. Ma, Palladium supported on magnetic nanoparticles as recoverable catalyst for one-pot reductive amination of aldehydes with nitroarenes under ambient conditions, Catal. Commun., 2013, 30, 40–44 CrossRef CAS.
- C. R. Rao, V. Lakshminarayanan and D. Trivedi, Synthesis and characterization of lower size, laurylamine protected palladium nanoparticles, Mater. Lett., 2006, 60, 3165–3169 CrossRef CAS.
- S.-W. Kim, M. Kim, W. Y. Lee and T. Hyeon, Fabrication of hollow palladium spheres and their successful application to the recyclable heterogeneous catalyst for Suzuki coupling reactions, J. Am. Chem. Soc., 2002, 124, 7642–7643 CrossRef CAS PubMed.
- S. Mubeen, T. Zhang, B. Yoo, M. A. Deshusses and N. V. Myung, Palladium nanoparticles decorated single-walled carbon nanotube hydrogen sensor, J. Phys. Chem. C, 2007, 111, 6321–6327 CAS.
- M. Hakamada, T. Furukawa, T. Yamamoto, M. Takahashi and M. Mabuchi, Abnormal hydrogen absorption/desorption properties of nanoporous Pt with large lattice strains, Mater. Trans., 2011, 52, 806–809 CrossRef CAS.
- W.-H. Hsu, S.-J. Jiang and A. Sahayam, Determination of Cu, As, Hg and Pb in vegetable oils by electrothermal vaporization inductively coupled plasma mass spectrometry with palladium nanoparticles as modifier, Talanta, 2013, 117, 268–272 CrossRef CAS PubMed.
- M. M. Kumari, S. A. Aromal and D. Philip, Synthesis of monodispersed palladium nanoparticles using tannic acid and its optical non-linearity, Spectrochim. Acta, Part A, 2013, 103, 130–133 CrossRef PubMed.
- A. Sartre, M. Phaner, L. Porte and G. Sauvion, STM and ESCA studies of palladium particles deposited on a HOPG surface, Appl. Surf. Sci., 1993, 70, 402–406 CrossRef.
- Y. Xiong, J. Chen, B. Wiley, Y. Xia, S. Aloni and Y. Yin, Understanding the role of oxidative etching in the polyol synthesis of Pd nanoparticles with uniform shape and size, J. Am. Chem. Soc., 2005, 127, 7332–7333 CrossRef CAS PubMed.
- S. U. Son, Y. Jang, K. Y. Yoon, E. Kang and T. Hyeon, Facile synthesis of various phosphine-stabilized monodisperse palladium nanoparticles through the understanding of coordination chemistry of the nanoparticles, Nano Lett., 2004, 4, 1147–1151 CrossRef CAS.
- S.-W. Kim, J. Park, Y. Jang, Y. Chung, S. Hwang, T. Hyeon and Y. W. Kim, Synthesis of monodisperse palladium nanoparticles, Nano Lett., 2003, 3, 1289–1291 CrossRef CAS.
- M. Tristany, J. Courmarcel, P. Dieudonné, M. Moreno-Manas, R. Pleixats, A. Rimola, M. Sodupe and S. Villarroya, Palladium nanoparticles entrapped in heavily fluorinated compounds, Chem. Mater., 2006, 18, 716–722 CrossRef CAS.
- Y. Xiong, J. Chen, B. Wiley, Y. Xia, Y. Yin and Z.-Y. Li, Size-dependence of surface plasmon resonance and oxidation for Pd nanocubes synthesized via a seed etching process, Nano Lett., 2005, 5, 1237–1242 CrossRef CAS PubMed.
- E. Abdel-Halim, M. El-Rafie and S. S. Al-Deyab, Polyacrylamide/guar gum graft copolymer for preparation of silver nanoparticles, Carbohydr. Polym., 2011, 85, 692–697 CrossRef CAS.
- D. Balaji, S. Basavaraja, R. Deshpande, D. B. Mahesh, B. Prabhakar and A. Venkataraman, Extracellular biosynthesis of functionalized silver nanoparticles by strains of Cladosporium cladosporioides fungus, Colloids Surf., B, 2009, 68, 88–92 CrossRef CAS PubMed.
- A. M. Fayaz, K. Balaji, P. Kalaichelvan and R. Venkatesan, Fungal based synthesis of silver nanoparticles—an effect of temperature on the size of particles, Colloids Surf., B, 2009, 74, 123–126 CrossRef PubMed.
- W. Huang, J. Yan, Y. Wang, C. Hou, T. Dai and Z. Wang, Biosynthesis of Silver Nanoparticles by Septoria apii and Trichoderma koningii, Chin. J. Chem., 2013, 31, 529–533 CrossRef CAS.
- A. Ahmad, S. Senapati, M. I. Khan, R. Kumar and M. Sastry, Extracellular biosynthesis of monodisperse gold nanoparticles by a novel extremophilic actinomycete, Thermomonospora sp, Langmuir, 2003, 19, 3550–3553 CrossRef CAS.
- A. Ahmad, S. Senapati, M. I. Khan, R. Kumar, R. Ramani, V. Srinivas and M. Sastry, Intracellular synthesis of gold nanoparticles by a novel alkalotolerant actinomycete, Rhodococcus species, Nanotechnology, 2003, 14, 824 CrossRef CAS.
- H. Lee, A. M. Purdon, V. Chu and R. M. Westervelt, Controlled assembly of magnetic nanoparticles from magnetotactic bacteria using microelectromagnets arrays, Nano Lett., 2004, 4, 995–998 CrossRef CAS.
- Z. Sadowski, Biosynthesis and application of silver and gold nanoparticles, 2010 Search PubMed.
- S. S. Shankar, A. Rai, A. Ahmad and M. Sastry, Rapid synthesis of Au, Ag, and bimetallic Au core–Ag shell nanoparticles using Neem (Azadirachta indica) leaf broth, J. Colloid Interface Sci., 2004, 275, 496–502 CrossRef CAS PubMed.
- K. Pooriya and M. Javad, Green synthesis, characterization and physiological stability of gold nanoparticles from Stachys lavandulifolia Vahl extract, Particuology, 2015, 19, 22–26 CrossRef.
- A. Aftab, W. Yun, S. Fatima, I. Muhammad, U. H. K. Zia, T. Kamran, U. K. Arif, R. Muslim, K. Qudratullah and Y. Qipeng, Size dependent catalytic activities of green synthesized gold nanoparticles and electrocatalytic oxidation of catechol on gold nanoparticles modified electrode, RSC Adv., 2015, 5, 99364 RSC.
- S. S. Shankar, A. Absar and S. Murali, Geranium Leaf Assisted Biosynthesis of Silver Nanoparticles, Biotechnol. Prog., 2003, 19, 1627–1631 CrossRef CAS PubMed.
- A. U. Khan, Y. Wei, Z. U. H. Khan, K. Tahir, S. U. Khan, A. Ahmad, F. U. Khan, L. Cheng and Q. Yuan, Electrochemical and Antioxidant Properties of Biogenic Silver Nanoparticles, Int. J. Electrochem. Sci., 2015, 10, 7905–7916 CAS.
- K. Tahir, B. Li, S. Khan, S. Nazir, Z. U. H. Khan, A. U. Khan and R. U. Islam, Enhanced chemocatalytic reduction of aromatic nitro compounds by biosynthesized gold nanoparticles, J. Alloys Compd., 2015, 651, 322–327 CrossRef CAS.
- B. Ankamwar, M. Chaudhary and M. Sastry, Gold nanotriangles biologically synthesized using tamarind leaf extract and potential application in vapor sensing, Synth. React. Inorg., Met.-Org., Nano-Met. Chem., 2005, 35, 19–26 CrossRef CAS.
- K. Tahir, S. Nazir, B. Li, A. U. Khan, Z. U. H. Khan, A. Ahmad and F. U. Khan, An efficient photo catalytic activity of green synthesized silver nanoparticles using Salvadora persica stem extract, Sep. Purif. Technol., 2015, 150, 316–324 CrossRef CAS.
- S. Li, Y. Shen, A. Xie, X. Yu, L. Qiu, L. Zhang and Q. Zhang, Green synthesis of silver nanoparticles using Capsicum annuum L. extract, Green Chem., 2007, 9, 852–858 RSC.
- K. Tahir, S. Nazir, B. Li, A. U. Khan, Z. U. H. Khan, A. Ahmad, Q. U. Khan and Y. Zhao, Enhanced visible light photocatalytic inactivation of Escherichia coli using silver nanoparticles as photocatalyst, J. Photochem. Photobiol., B, 2015, 153, 261–266 CrossRef CAS PubMed.
- N. Kannan and M. M. Sundaram, Kinetics and mechanism of removal of methylene blue by adsorption on various carbons—a comparative study, Dyes Pigm., 2001, 51, 25–40 CrossRef CAS.
- N. A. Fathy, O. I. El-Shafey and L. B. Khalil, Effectiveness of Alkali-Acid Treatment in Enhancement the Adsorption Capacity for Rice Straw: The Removal of Methylene
Blue Dye, ISRN Phys. Chem., 2013, 2013, 1–15 CrossRef.
- L. Núñez, J. A. García-Hortal and F. Torrades, Study of kinetic parameters related to the decolourization and mineralization of reactive dyes from textile dyeing using Fenton and photo-Fenton processes, Dyes Pigm., 2007, 75, 647–652 CrossRef.
- M. A. Ahmad and R. Alrozi, Removal of malachite green dye from aqueous solution using rambutan peel-based activated carbon: Equilibrium, kinetic and thermodynamic studies, Chem. Eng. J., 2011, 171, 510–516 CrossRef CAS.
- W. Zou, K. Li, H. Bai, X. Shi and R. Han, Enhanced cationic dyes removal from aqueous solution by oxalic acid modified rice husk, J. Chem. Eng. Data, 2011, 56, 1882–1891 CrossRef CAS.
- J. L. Hughart and M. M. Bashor, Substances, A. f. T.; Registry, D.; America, U. S. o. Industrial chemicals and terrorism: human health threat analysis, mitigation and prevention, US Department of Health and Human Services, Agency for Toxic Substances and Disease Registry, 2000 Search PubMed.
- J. F. Corbett, An historical review of the use of dye precursors in the formulation of commercial oxidation hair dyes, Dyes Pigm., 1999, 41, 127–136 CrossRef CAS.
- H. W. Murray, J. D. Berman, C. R. Davies and N. G. Sara via, Advances in leishmaniasis, Lancet, 2005, 366, 1561–1577 CrossRef CAS.
- A. U. Khan, Q. Yuan, Y. Wei, K. Tahir, S. U. Khan, A. Ahmad, S. Khan, S. Nazir and F. U. Khan, Ultra-efficient photocatalytic deprivation of methylene blue and biological activities of biogenic silver nanoparticles, J. Photochem. Photobiol., B, 2016, 159, 49–58 CrossRef PubMed.
- A. Ahmad, F. Syed, A. Shah, Z. Khan, K. Tahir, A. U. Khan and Q. Yuan, Silver and gold nanoparticles from Sargentodoxa cuneata: synthesis, characterization and antileishmanial activity, RSC Adv., 2015, 5, 73793–73806 RSC.
- K. Chamakura, R. Perez-Ballestero, Z. Luo, S. Bashir and J. Liu, Comparison of bactericidal activities of silver nanoparticles with common chemical disinfectants, Colloids Surf., B, 2011, 84, 88–96 CrossRef CAS PubMed.
- J. R. Morones, J. L. Elechiguerra, A. Camacho, K. Holt, J. B. Kouri, J. T. Ramírez and M. J. Yacaman, The bactericidal effect of silver nanoparticles, Nanotechnology, 2005, 16, 2346 CrossRef CAS PubMed.
- A. Ziouti, C. E. Modafar, A. Fleuriet, S. E. Boustani and J. J. Macheix, Phenolic compounds in date palm cultivars sensitive and resistant to Fusarium oxysporum, Biol. Plant., 1996, 38, 451–457 CrossRef CAS.
- S. Roy, S. Roy, B. Neuenswander, D. Hill and R. C. Larock, Solution-phase synthesis of a diverse isocoumarin library, J. Comb. Chem., 2009, 11, 1128–1135 CrossRef CAS PubMed.
- K. Rhayour, T. Bouchikhi, A. Tantaoui-Elaraki, K. Sendide and A. Remmal, The mechanism of bactericidal action of oregano and clove essential oils and of their phenolic major components on Escherichia coli and Bacillus subtilis, J. Essent. Oil Res., 2003, 15, 286–292 CrossRef CAS.
- W. S. Vedakumari, P. Prabu, S. C. Babu and T. P. Sastry, Fibrin nanoparticles as possible vehicles for drug delivery, Biochim. Biophys. Acta, Gen. Subj., 2013, 1830, 4244–4253 CrossRef CAS PubMed.
- C. W. Choi, S. C. Kim, S. S. Hwang, B. K. Choi, H. J. Ahn, M. Y. Lee, S. H. Park and S. K. Kim, Antioxidant activity and free radical scavenging capacity between Korean medicinal plants and flavonoids by assay-guided comparison, Plant Sci., 2002, 163, 1161–1168 CrossRef CAS.
- M. Noginov, G. Zhu, M. Bahoura, J. Adegoke, C. Small, B. Ritzo, V. Drachev and V. Shalaev, Enhancement of surface plasmons in an Ag aggregate by optical gain in a dielectric medium, Opt. Lett., 2006, 31, 3022–3024 CrossRef CAS PubMed.
- K. L. Kelly, E. Coronado, L. L. Zhao and G. C. Schatz, The optical properties of metal nanoparticles: the influence of size, shape, and dielectric environment, J. Phys. Chem. B, 2003, 107, 668–677 CrossRef CAS.
- D. Philip, Biosynthesis of Au, Ag and Au–Ag nanoparticles using edible mushroom extract, Spectrochim. Acta, Part A, 2009, 73, 374–381 CrossRef PubMed.
- J. Mock, M. Barbic, D. Smith, D. Schultz and S. Schultz, Shape effects in plasmon resonance of individual colloidal silver nanoparticles, J. Chem. Phys., 2002, 116, 6755–6759 CrossRef CAS.
- S. Link and M. A. El-Sayed, Spectral properties and relaxation dynamics of surface plasmon electronic oscillations in gold and silver nanodots and nanorods, J. Phys. Chem. B, 1999, 103, 8410–8426 CrossRef CAS.
- Z. U. H. Khan, A. Khan, A. Shah, Y. Chen, P. Wan, A. u. Khan, K. Tahir, N. Muhamma, F. U. Khan and H. U. Shah, Photocatalytic, antimicrobial activities of biogenic silver nanoparticles and electrochemical degradation of water soluble dyes at glassy carbon/silver modified past electrode using buffer solution, J. Photochem. Photobiol., B, 2016, 156, 100–107 CrossRef PubMed.
- V. Kumar, S. C. Yadav and S. K. Yadav, Syzygium cumini leaf and seed extract mediated biosynthesis of silver nanoparticles and their characterization, J. Chem. Technol. Biotechnol., 2010, 85, 1301–1309 CrossRef CAS.
- D. Philip, C. Unni, S. A. Aromal and V. Vidhu, Murraya koenigii leaf-assisted rapid green synthesis of silver and gold nanoparticles, Spectrochim. Acta, Part A, 2011, 78, 899–904 CrossRef PubMed.
- L. Jia, Q. Zhang, Q. Li and H. Song, The biosynthesis of palladium nanoparticles by antioxidants in Gardenia jasminoides Ellis: long lifetime nanocatalysts for p-nitrotoluene hydrogenation, Nanotechnology, 2009, 20, 385601 CrossRef PubMed.
- R. Emmanuel, C. Karuppiah, S.-M. Chen, S. Palanisamy, S. Padmavathy and P. Prakash, Green synthesis of gold nanoparticles for trace level detection of a hazardous pollutant (nitrobenzene) causing Methemoglobinaemia, J. Hazard. Mater., 2014, 279, 117–124 CrossRef CAS PubMed.
- A. Panáček, L. Kvitek, R. Prucek, M. Kolar, R. Vecerova, N. Pizurova, V. K. Sharma, T. j. Nevečná and R. Zboril, Silver colloid nanoparticles: synthesis, characterization, and their antibacterial activity, J. Phys. Chem. B, 2006, 110, 16248–16253 CrossRef PubMed.
- A. K. Suresh, M. J. Doktycz, W. Wang, J. W. Moon, B. Gu, H. M. Meyer III, D. K. Hensley, S. T. Retterer, D. P. Allison and T. J. Phelps, Monodispersed biocompatible Ag2S nanoparticles: Facile extracellular bio-fabrication using the gamma-proteobacterium, S. oneidensis, 2011 Search PubMed.
- K. B. Holt and A. J. Bard, Interaction of silver(I) ions with the respiratory chain of Escherichia coli: an electrochemical and scanning electrochemical microscopy study of the antimicrobial mechanism of micromolar Ag+, Biochemistry, 2005, 44, 13214–13223 CrossRef CAS PubMed.
- X. Gao, J. Zhang and L. Zhang, Hollow sphere selenium nanoparticles: their in vitro anti hydroxyl radical effect, Adv. Mater., 2002, 14, 290 CrossRef CAS.
- K. Tahir, S. Nazir, B. Li, A. U. Khan, Z. U. H. Khan, P. Y. Gong, S. U. Khan and A. Ahmad, Nerium oleander leaves extract mediated synthesis of gold nanoparticles and its antioxidant activity, Mater. Lett., 2015, 156, 198–201 CrossRef CAS.
- Q. Y. Zhu, R. R. Holt, S. A. Lazarus, T. J. Orozco and C. L. Keen, Inhibitory effects of cocoa flavanols and procyanidin oligomers on free radical-induced erythrocyte hemolysis, Exp. Biol. Med., 2002, 227, 321–329 CAS.
- N. Pradhan, A. Pal and T. Pal, Silver nanoparticle catalyzed reduction of aromatic nitro compounds, Colloids Surf., A, 2002, 196, 247–257 CrossRef CAS.
Footnote |
† Electronic supplementary information (ESI) available. See DOI: 10.1039/c6ra11409a |
|
This journal is © The Royal Society of Chemistry 2016 |