DOI:
10.1039/C6RA07827C
(Paper)
RSC Adv., 2016,
6, 66233-66241
Lysozyme-stabilized Ag nanoclusters: synthesis of different compositions and fluorescent responses to sulfide ions with distinct modes†
Received
25th March 2016
, Accepted 27th June 2016
First published on 29th June 2016
Abstract
In this study, three red-emissive Ag nanoclusters capped with denatured lysozyme (dLys-AgNCs 1–3) were synthesized from different reactant molar ratios of AgNO3 and lysozyme. dLys-AgNCs 1 contained only Ag(0), whereas dLys-AgNCs 2 and 3 contained both Ag(0) and Ag(I). The maximum emission wavelengths of dLys-AgNCs 1–3 were all near 640 nm when excited at 490 nm, but had different photoluminescence intensities. The photoluminescence properties of the dLys-AgNCs in the presence of common inorganic anions were studied. dLys-AgNCs 1–3 had a distinct photoluminescence response to S2−. The photoluminescence of dLys-AgNCs 1 was quenched by S2−, because S2− destroyed the nanostructure of dLys-AgNCs 1 by forming Ag2S. The photoluminescence of dLys-AgNCs 3 was enhanced by S2−, because S2− bound to Ag(I) in dLys-AgNCs 3 and altered the ligand-to-metal–metal charge transfer. For dLys-AgNCs 2, the photoluminescence was enhanced with a small amount of S2− and quenched when more S2− was added. This was explained by S2− binding to Ag(I) at low concentrations and then to Ag(0) at high concentrations. Other anions had no obvious effects, except that I− quenched the photoluminescence of dLys-AgNCs 1–3. The response mechanisms were investigated by XPS, MS, zeta potential, DLS, and fluorescence lifetime measurements. As-prepared dLys-AgNCs 1 and 3 were used as fluorescent probes for S2− detection in tap water and lake water with satisfactory results.
1. Introduction
Metal nanoclusters (NCs) have attracted attention as fluorophores.1–4 Compared with organic dyes and semiconductor quantum dots (QDs), metal nanoclusters have superior features such as outstanding photostability, low biotoxicity and good bio-compatibility.5,6 As the bridge between single atoms and nanoparticles, metal nanoclusters have been investigated extensively in the development of nanotechnology.7 In the past decades, various metal nanoclusters have been synthesized, such as AuNCs,8–10 AgNCs,11–13 PtNCs14–16 and CuNCs.17–19 AgNCs have been commonly used because of their facile synthesis and red emission.
To satisfy the needs of biotechnology and biomedicine, the scaffolds used to synthesize AgNCs have been developed, from thiols20 and polymers21–23 to DNA,24–26 peptides27 and proteins.28,29 As a stabilizer for metal nanoclusters, proteins are more water soluble and biocompatible than macromolecular polymers like PIE or PMAA, and more stable than DNA and other small molecular thiol compounds, like Cys or GSH. Proteins are excellent templates for protecting nanoclusters because they require milder reaction conditions, provide size control, and contain many efficient binding functional groups, such as thiol, amine, hydroxyl and carboxyl groups, in their binding sites.30 Xie and coworkers31 first reported a simple, one-pot, green route for preparing red-emissive Au nanoclusters (AuNCs) using bovine serum albumin (BSA) as the template. The thiol groups (–SH) of 35 cysteine residues in the BSA monomer conjugated with Au via Au–S bonding, and the AuNCs formed in BSA were highly stable both in solution and solid form. Other proteins, including lysozyme,32 insulin,33 pepsin,34 trypsin,35 horseradish peroxidase36 and lactotransferrin,37 have also been used as scaffolds for the synthesis of metal nanoclusters. The four disulfide bonds in the secondary structure of lysozyme were broken under alkaline conditions during synthesis, and could provide binding sites and stabilization for Ag nanoclusters.28
The photoluminescence of metal nanoclusters originates from the ligand-to-metal charge transfer (LMCT)38,39 and ligand-to-metal–metal charge transfer (LMMCT).40 Wu and Jin38 reported that the charge transfer from the ligands to the metal nanoparticle core played an important role in enhancing the fluorescence of metal nanoparticles. Chen et al.40 proposed that the emission from Ag–carboxylate NCs could be attributed to ligand-to-metal–metal charge transfer of Ag(I)–carboxylate complexes, from the oxygen atom in the carboxylate ligands to the Ag(I) ions, and then to the Ag atoms, followed by radiative relaxation. Thus, metal ions, such as Ag(I), could be contained in AgNCs and play a crucial role in the photoluminescence of metal nanoclusters.
Sulfide ions (S2−), which widely exist in wastewater or natural water and tap water, are an important environmental index due to their high toxicity to aquatic organisms and humans.41,42 Increasing numbers of nanomaterials have been developed for detecting S2−.43–48 Chen's group45 used blue-emitting glutathione-stabilized Ag nanoclusters (GSH-AgNCs) as a probe for sensitive and selective detection of S2−. The fluorescence of GSH-AgNCs was quenched when S2− was added by forming Ag2S, which has low solubility. Chang's group46 used DNA-templated gold/silver nanoclusters (DNA-Au/Ag NCs) for detecting S2− with the same mechanism. Cu nanoclusters (CuNCs) function as a turn-off probe for sensing S2− by forming CuS, which has low solubility.47 Lu's group reported that S2− could enhance the photoluminescence intensity of CuNCs capped with cysteine owing to the aggregation-induced emission enhancement.48
In this work, the composition effects on the photoluminescence properties of as-prepared dLys-AgNCs were explored. As-synthesized dLys-AgNCs 1 contained only Ag(0), whereas dLys-AgNCs 2 and 3 contained both Ag(0) and Ag(I). dLys-AgNCs 1–3 had distinct photoluminescence response modes to S2− because of the different LMCT and LMMCT processes. Other common inorganic anions had no obvious effects, except for I−, which quenched the photoluminescence of the probes. As-prepared dLys-AgNCs 1 and 3 could be used for detecting S2− with turn-off and turn-on modes, respectively. S2− was detected in tap water and lake water by using dLys-AgNCs 1 and 3 as the fluorescent probes and the results were satisfactory.
2. Experimental
2.1 Materials
Silver nitrate (AgNO3) was purchased from Tianjin Heowns Biochem Technologies LLC. Lysozyme was purchased from Beijing Dingguo Biotechnology Company. Sodium borohydride (NaBH4, 96%), sodium sulfide nonahydrate (Na2S·9H2O), and other reagents, including acetic acid (CH3COOH), sodium hydroxide (NaOH), sodium nitrate (NaNO3), sodium nitrite (NaNO2), sodium phosphate tribasic dodecahydrate (Na3PO4·12H2O), disodium hydrogen phosphate dodecahydrate (Na2HPO4·12H2O), sodium dihydrogen phosphate dehydrate (NaH2PO4·2H2O), sodium carbonate (NaCO3), sodium hydrogen carbonate (NaHCO3), sodium sulfate (Na2SO4), sodium sulfite (Na2SO3), sodium fluoride (NaF), sodium chloride (NaCl), sodium bromide (NaBr) and sodium iodide (NaI), were purchased from Sinopharm Chemical Reagent Co., Ltd. All reagents were of analytical grade and were used as received without further purification. All stock solutions were prepared with ultrapure water, which was obtained from a Millipore Milli-Q water purification system and had an electric resistance over 18.0 MΩ.
2.2 Instrumentation
Photoluminescence (PL) spectra were obtained on a Hitachi F-4600 fluorescence spectrophotometer. UV-visible absorption spectra were obtained on a TU-1901 diode-array spectrophotometer. Zeta potential and dynamic light scattering (DLS) measurements were performed with a Brookhaven zeta potential analyzer. X-ray photoelectron spectroscopy was conducted on a Thermo Fisher ESCALAB 250Xi X-ray photoelectron spectrometer. For MALDI-time-of-flight (TOF) mass spectrometry (MS) analysis, an AB Sciex 5800 MALDI TOF/TOF analyzer was used with 2,4,6-trihydroxyacetophenone as the matrix. Transmission electron microscopy (TEM) was performed on a TF20 (FEI) microscope. Time-resolved fluorescence measurements were obtained on an OB920 single-photon counting fluorometer. The pH was determined by a REX PHS-3C pH meter.
2.3 Preparation of dLys-AgNCs
dLys-AgNCs 1–3 were prepared according to Chen et al.28 with some modifications. The reactant molar ratios of AgNO3 to lysozyme were 1
:
1, 2
:
1 and 4
:
1 for synthesizing dLys-AgNCs 1, 2 and 3, respectively.
For the synthesis of dLys-AgNCs 1, 15 mg mL−1 lysozyme solution (5 mL) was mixed with 5 mmol L−1 AgNO3 solution (1 mL), and the mixture was vigorously stirred at room temperature for 5 min. Then, 1 mol L−1 NaOH solution (0.3 mL) was added dropwise. After 5 min of stirring, 20 mmol L−1 NaBH4 (10 μL) was injected into the mixture. The reaction was allowed to proceed for 15 min with vigorous stirring in the dark at room temperature to obtain dLys-AgNCs 1. Stable dLys-AgNCs 1 was produced by adding 1 mol L−1 acetic acid (0.5 mL) to the solution to neutralize excess NaOH, and the mixture was stirred for 5 more min.
To synthesize dLys-AgNCs 2 and 3, 10 and 20 mmol L−1 AgNO3 solutions, respectively, were used with the other conditions unchanged. The as-synthesized dLys-AgNCs were stored in the dark at 4 °C in a refrigerator.
2.4 UV-visible absorption spectra and fluorescence spectra measurements of dLys-AgNCs
For UV-visible absorption and photoluminescence spectra measurements, the stock solutions of dLys-AgNCs 1–3 were diluted 10 times. A mixture of dLys-AgNCs stock solution (80 μL) and HAc–NaAc buffer (80 μL, pH 4.6, 0.2 mol L−1) was diluted with ultrapure water to a final volume of 800 μL. The photoluminescence spectra were recorded with an excitation wavelength of 490 nm.
2.5 XPS measurements
As-synthesized dLys-AgNCs 1–3 were purified through a dialysis bag (molecular weight cut off: 8000 Da) for 24 h and then dried in a drying oven at 40 °C for 6 h, and then in a vacuum drying oven for 3 days. After tableting, the X-ray photoelectron spectra of dLys-AgNCs 1–3 were measured. The XPS spectra were deconvoluted by XPS PEAK 41.
2.6 Photoluminescence responses of dLys-AgNCs 1–3 to S2− and other anions
To measure the photoluminescence responses of dLys-AgNCs 1–3 to S2− and other anions, including NO3−, NO2−, PO43−, HPO42−, H2PO4−, CO32−, HCO3−, SO42−, SO32−, F−, Cl−, Br− and I−, the probes were diluted 20 times. The concentration of each anion was 10.0 μmol L−1 for dLys-AgNCs 1 and 3, and 5.0 and 15.0 μmol L−1 for dLys-AgNCs 2.
To examine the photoluminescence responses of dLys-AgNCs 1–3 to S2−, concentrations of S2− 0, 2.0, 4.0, 6.0, 8.0, 10.0, 15.0, and 20.0 μmol L−1 were used. The sensing properties of dLys-AgNCs 1 and 3 as fluorescent probes for detecting S2− were determined at S2− concentrations of 0, 0.5, 1, 2, 4, 6, 8, 10, 12, 14, and 16 μmol L−1 for dLys-AgNCs 1, and 0, 1, 2, 3, 4, 5, 7, 9, 11, and 12 μmol L−1 for dLys-AgNCs 3.
dLys-AgNCs (40 μL), HAc–NaAc buffer solution (80 μL, pH 4.6, 0.2 mol L−1) and the anion were added to a 1.5 mL micro-centrifuge tube and were diluted with ultrapure water to a final volume of 800 μL. The mixtures were incubated in the dark for about 15 min at room temperature. The photoluminescence intensity at 640 nm was recorded with an excitation wavelength of 490 nm.
2.7 Zeta potential, DLS and fluorescence lifetime
For the zeta potential, dynamic light scattering (DLS) and fluorescence lifetime measurements, dLys-AgNCs 1 and 3 were diluted 20 times from their stock solutions in the absence or presence of 5 μmol L−1 S2− or 10 μmol L−1 I−. For fluorescence lifetime measurements, the excitation wavelength was 448 nm and the fluorescence decay at 640 nm was recorded.
2.8 Detection of S2− in water samples using dLys-AgNCs 1 and 3
Tap water was taken from our lab, and lake water was taken from Taoranting Park, Beijing. The water samples were filtered through a 0.22 μm membrane and diluted 5 times. The test samples were prepared by adding different concentrations of S2− to the water sample. The photoluminescence intensity at 640 nm was measured and the detected concentration was calculated according to the linear equation for dLys-AgNCs 1 or dLys-AgNCs 3. The recovery was the ratio of the detected concentration (found) to the added concentration (added).
3. Results and discussion
3.1 Characterization of as-prepared dLys-AgNCs
The photophysical properties of dLys-AgNCs 1–3 were characterized by UV-visible absorption spectra and fluorescence spectra, which showed that dLys-AgNCs 1–3 had similar photophysical properties (Fig. 1). Fig. 1A shows that dLys-AgNCs 1–3 had a broad absorption band in the UV-visible range with a peak centered around 490 nm, which was attributed to ligand-to-metal charge transfer (LMCT)39,49 or ligand-to-metal–metal charge transfer (LMMCT).40 Fig. 1B(a) shows that dLys-AgNCs 1, which was typical of dLys-AgNCs, had two excitation wavelengths at 370 and 490 nm, respectively. When excited at 490 nm, one emission band centered at 640 nm emerged (Fig. 1B(b)). When excited at 370 nm, dLys-AgNCs 1 exhibited a weak emission band centered at 445 nm and a strong emission band centered at 640 nm (Fig. 1B(c)). Fig. 1C indicated that dLys-AgNCs 1–3 possessed similar fluorescent emission spectra with maximum emission wavelengths around 640 nm when excited at 490 nm.
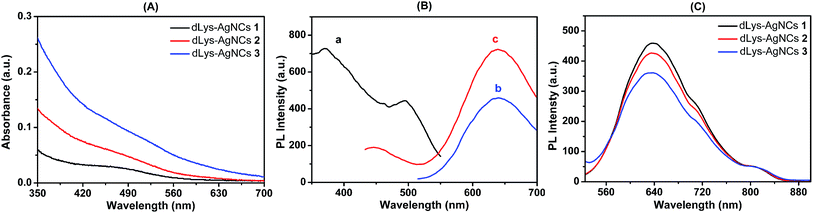 |
| Fig. 1 (A) Absorption spectra of as-prepared dLys-AgNCs 1–3. (B) Fluorescence spectra of dLys-AgNCs 1: (a) excitation spectra; (b) emission spectra when excited at 490 nm; and (c) emission spectra when excited at 370 nm. (C) Photoluminescence spectra of dLys-AgNCs 1–3 at an excitation wavelength of 490 nm. | |
The transmission electron micrograph (TEM) in Fig. 2 showed that as-prepared dLys-AgNCs 1–3 were spherical and well dispersed. The average diameters of dLys-AgNCs 1, 2 and 3 were 4.0, 3.2 and 2.7 nm, respectively.
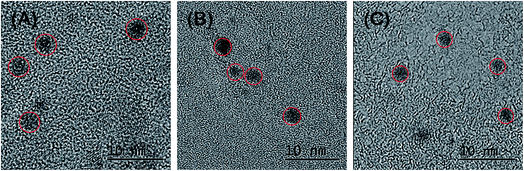 |
| Fig. 2 HRTEM images of as-prepared dLys-AgNCs 1–3. (A) dLys-AgNCs 1; (B) dLys-AgNCs 2; and (C) dLys-AgNCs 3. | |
To explore the composition of the synthesized dLys-AgNCs, X-ray photoelectron spectroscopy (XPS) measurements were performed to reveal the valence state of Ag. The XPS results showed that Ag in dLys-AgNCs 1–3 had various valence states. For dLys-AgNCs 1 (Fig. 3A), there were a group of Ag 3d peaks located at 368.18 eV (Ag 3d5/2) and another at 374.33 eV (Ag 3d3/2). The energy gap between the two peaks was nearly 6.0 eV, which confirmed the presence of Ag(0) in dLys-AgNCs 1. For dLys-AgNCs 2 and 3 (Fig. 3B and C), each of the two groups of Ag 3d peaks could be deconvoluted into two components, two of which were the intrinsic peaks for Ag(0), like dLys-AgNCs 1, and the other two peaks were located at 369.03 (Ag 3d5/2) and 375.18 eV (Ag 3d3/2), indicating the presence of Ag(I). These results showed that Ag(I) was present in dLys-AgNCs 2 and 3 after synthesis.
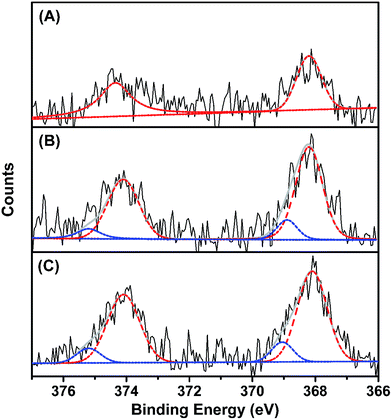 |
| Fig. 3 XPS results of the as-prepared (A) dLys-AgNCs 1; (B) dLys-AgNCs 2; and (C) dLys-AgNCs 3. The original spectra are in black, the Ag(I) 3d spectra are in blue and the Ag(0) 3d spectra are in red. | |
Moreover, as-prepared dLys-AgNCs 1–3 had outstanding stability. As shown in Fig. S1,† dLys-AgNCs 1 could be stored for nearly 4 months in the dark at 4 °C with a reduction in fluorescence intensity of less than 5%. The long period of stability was sufficient for using as-synthesized dLys-AgNCs 1–3 as convenient, stable and reliable fluorescent probes for analytical detection and optical bioimaging.
3.2 Photoluminescence responses of as-prepared dLys-AgNCs to anions and the interaction mechanisms with S2− and I−
The photoluminescence responses of dLys-AgNCs 1–3 to common inorganic anions were investigated, and the results are shown in Fig. 4 for dLys-AgNCs 1 and 3 and Fig. S2† for dLys-AgNCs 2. Fig. 4A showed that most anions, including NO3−, NO2−, PO43−, HPO42−, H2PO4−, CO32−, HCO3−, SO42−, SO32−, F−, and Cl−, had negligible effects on the photoluminescence of dLys-AgNCs 1, and Br− had a slight quenching effect. I− quenched the photoluminescence of dLys-AgNCs 1 moderately, whereas S2− quenched the photoluminescence of dLys-AgNCs 1 strongly. For dLys-AgNCs 3 (Fig. 4B), anions such as NO2−, PO43−, HPO42−, SO42−, SO32−, F−, Cl− and Br− slightly enhanced the photoluminescence of dLys-AgNCs 3, whereas S2− enhanced the photoluminescence greatly. In contrast, I− quenched the photoluminescence of dLys-AgNCs 3 strongly. Fig. S2† showed that S2− enhanced the photoluminescence of dLys-AgNCs 2 at a low concentration of 5 μmol L−1 and quenched the photoluminescence of dLys-AgNCs 2 at a high concentration of 15 μmol L−1. Most anions, such as NO3−, NO2−, PO43−, HPO42−, H2PO4−, CO32−, HCO3−, SO42−, SO32−, F−, Cl− and Br−, had negligible effects on the photoluminescence of dLys-AgNCs 2, whereas I− quenched its photoluminescence moderately. These results indicated that as-prepared dLys-AgNCs 1–3 possessed distinct response modes toward S2−.
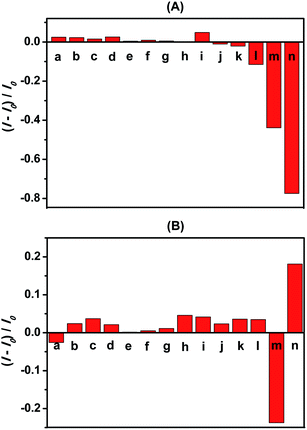 |
| Fig. 4 Responses of (A) dLys-AgNCs 1 and (B) dLys-AgNCs 3 to different anions studies ((a) NO3−, (b) NO2−, (c) PO43−, (d) HPO42−, (e) H2PO4−, (f) CO32−, (g) HCO3−, (h) SO42−, (i) SO32−, (j) F−, (k) Cl−, (l) Br−, (m) I−, (n) S2−). The concentration of each anion was 10 μmol L−1. I0 and I are the photoluminescence intensity of dLys-AgNCs at 640 nm in the absence and presence of anions, respectively. The excitation wavelength was 490 nm. | |
The photoluminescence responses of dLys-AgNCs 1–3 to different concentrations of S2− of 0, 2.0, 4.0, 6.0, 8.0, 10.0, 15.0, and 20.0 μmol L−1 were determined (Fig. 5). The photoluminescence of dLys-AgNCs 1 was quenched by S2−, whereas the photoluminescence of dLys-AgNCs 3 was enhanced in the presence of S2−. For dLys-AgNCs 2, the photoluminescence was initially enhanced with a small amount of S2− and then quenched as more S2− was added.
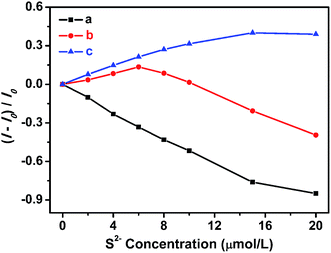 |
| Fig. 5 Relationship between the photoluminescence intensity changes of dLys-AgNCs 1–3 and different concentrations of S2−. (a) dLys-AgNCs 1; (b) dLys-AgNCs 2; and (c) dLys-AgNCs 3. I0 and I are the photoluminescence intensity of the dLys-AgNCs at 640 nm in the absence and presence of S2−, respectively. The excitation wavelength was 490 nm. | |
To clarify the interactions between the dLys-AgNCs and S2−, the MALDI-TOF mass spectrometry of dLys-AgNCs 1 and 3 in the absence and presence of S2− were measured. As shown in Fig. 6, the mass peak of denatured lysozyme was located at m/z 14
312 Da, and the peak for dLys-AgNCs was the shoulder near the denatured lysozyme peak. Fig. 6A shows that the mass peak of dLys-AgNCs 1 appeared at m/z 14
767 Da, which meant that 4.217 Ag atoms were contained in each denatured lysozyme molecule. In the presence of S2−, the mass peak at 14
562 Da showed that there were only 2.210 Ag atoms left in each denatured lysozyme molecule (Fig. 6B). Thus, the S2− reacted with the Ag atoms in dLys-AgNCs 1 by forming Ag2S, as previously reported,43,46,47 leading to the photoluminescence quenching. The formation of Ag2S was confirmed by the XPS results. Fig. S3† shows that the Ag(I) peak appeared when S2− was introduced to dLys-AgNCs 1. Fig. 6C and D show that the m/z value of dLys-AgNCs 3 increased from 14
484.7910 to 14
566.7256 after S2− was added. Therefore, S2− bound to Ag(I) in dLys-AgNCs 3 and altered the LMMCT process, increasing the photoluminescence of dLys-AgNCs 3.
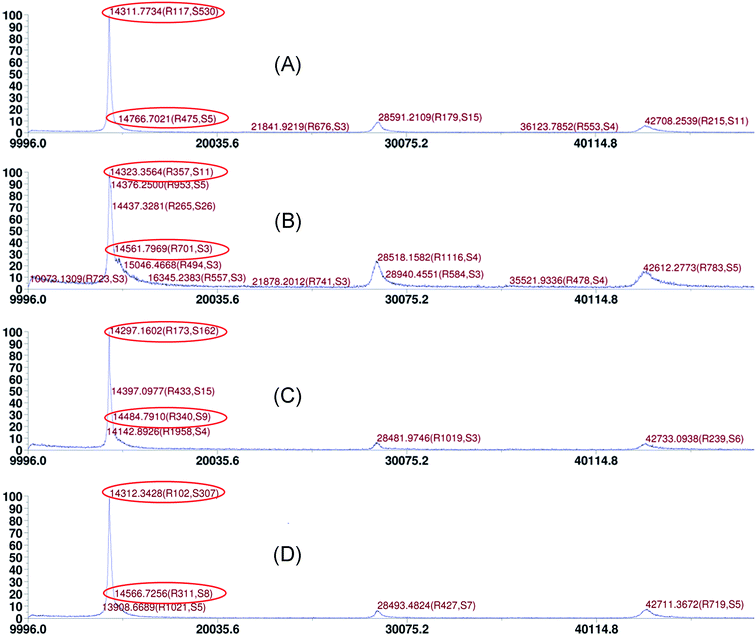 |
| Fig. 6 MALDI-TOF mass spectra of dLys-AgNCs 1 and 3 in the absence and presence of S2−: (A) dLys-AgNCs 1; (B) dLys-AgNCs 1 + 10 μmol L−1 S2−; (C) dLys-AgNCs 3; and (D) dLys-AgNCs 3 + 10 μmol L−1 S2−. | |
The zeta potential and dynamic light scattering (DLS) of dLys-AgNCs 1 and 3 in the absence and presence of S2− were measured. As-prepared dLys-AgNCs 1 was electrically neutral and S2− had no effect on its electrical properties (Fig. 7a and b). In contrast, for dLys-AgNCs 3, in addition to a big electroneutral peak, a small peak with positive potential at +27.2 mV was observed (Fig. 7c). The positive peak was attributed to Ag(I) contained in dLys-AgNCs 3,50 which was also observed in the XPS results (Fig. 3C). The peak with a positive potential shifted to +17.4 mV or disappeared when 5 or 10 μmol L−1 S2− was added (Fig. 7d and e). S2− may have reacted with Ag(I) in dLys-AgNCs 3 and neutralized the positive charge.
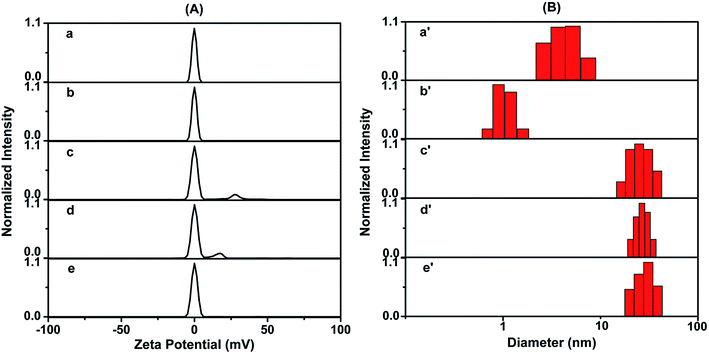 |
| Fig. 7 (A) Zeta potential and (B) DLS measurements of dLys-AgNCs 1 and 3 in the absence and presence of S2− ((a and a′) dLys-AgNCs 1, (b and b′) dLys-AgNCs 1 + 10 μmol L−1 S2−, (c and c′) dLys-AgNCs 3, (d and d′) dLys-AgNCs 3 + 5 μmol L−1 S2−, (e and e′) dLys-AgNCs 3 + 10 μmol L−1 S2−). | |
For DLS measurements, when S2− was added, the hydrodynamic diameter of dLys-AgNCs 1 decreased obviously from 4.2 ± 2.0 to 1.1 ± 0.4 nm (Fig. 7a′ and b′), whereas that of dLys-AgNCs 3 increased slightly (Fig. 7c′–e′). Therefore, S2− bound to Ag(0) in dLys-AgNCs 1, forming Ag2S, and the loss of Ag atoms decreased the size of dLys-AgNCs 1. However, S2− bound to Ag(I) in the structure of Lys-AgNCs 3 rather than destroying it.
To clarify the interactions between as-prepared dLys-AgNCs and I−, which showed a strong response, the zeta potential and hydrodynamic diameter of dLys-AgNCs 1 and 3 in the absence and presence of I− were also measured (Fig. S4†). The electrical properties of dLys-AgNCs 1 were not changed by I− (Fig. S4a and b†), and the hydrodynamic diameter of dLys-AgNCs 1 was decreased from 4.1 ± 1.3 to 1.0 ± 0.4 nm when I− was added (Fig. S4a′ and b′†), which was similar to the results for S2−. For dLys-AgNCs 3, the positive potential at +27.2 mV decreased after I− was introduced (Fig. S4c and d†), which meant that I− reacted with Ag(I) in dLys-AgNCs 3 and decreased the positive charge of dLys-AgNCs 3. In contrast to S2−, the hydrodynamic diameter of dLys-AgNCs 3 decreased slightly (Fig. S4c′ and d′†). These results indicated that I− destroyed the nanostructures of dLys-AgNCs 1 and 3 by forming AgI,51–53 which has a low Ksp, and quenching the photoluminescence.
The lifetime of dLys-AgNCs 1 and 3 before and after the addition of S2− were measured (Fig. S5†), and the results are shown in Table 1. For dLys-AgNCs 1 and 3, three lifetimes were obtained. The lifetimes of dLys-AgNCs 1 showed no significant change when S2− was introduced, which indicated that the photoluminescence quenching of dLys-AgNCs 1 by S2− was a static quenching process. S2− reacted with dLys-AgNCs 1 in the ground state by forming Ag2S and dissociating the structure, leading to photoluminescence quenching. However, for dLys-AgNCs 3, two of the three lifetimes were decreased when S2− was added. S2− bound to Ag(I) in dLys-AgNCs 3 via electrostatic interactions, altering the LMMCT process and increasing the photoluminescence even though the lifetimes were decreased.
Table 1 Lifetimes of dLys-AgNCs 1 and 3 in the absence and presence of S2−
|
τ1 (ns) |
τ2 (ns) |
τ3 (ns) |
dLys-AgNCs 1 |
1.81 |
0.34 |
6.40 |
dLys-AgNCs 1 + 10 μmol L−1 S2− |
1.86 |
0.36 |
6.42 |
dLys-AgNCs 3 |
1.43 |
0.28 |
5.26 |
dLys-AgNCs 3 + 10 μmol L−1 S2− |
1.31 |
0.23 |
4.77 |
The lifetimes of dLys-AgNCs 1 and 3 in the presence of I− were also measured, and the results are shown in Table S1.† The lifetimes of both dLys-AgNCs 1 and 3 were decreased after I− was introduced, which indicated that I− quenched the photoluminescence via a dynamic quenching process. I− quenches fluorescence via the heavy atom effect and charge transfer. The DLS results in Fig. S4(B)† showed that the hydrodynamic diameter of dLys-AgNCs 1 and 3 were decreased when I− was added, indicating that AgI was formed in the ground state. Thus, the photoluminescence quenching of dLys-AgNCs 1 and 3 by I− was caused by dynamic quenching and static quenching.
Based on these results, the response mechanisms of dLys-AgNCs 1 and 3 to S2− were proposed as shown in Scheme 1. The photoluminescence of dLys-AgNCs 1 is quenched by S2− due to the breakdown of the nanostructure and the formation of poorly soluble Ag2S. The photoluminescence of dLys-AgNCs 3 is enhanced by S2−, because S2− reacts with Ag(I) in dLys-AgNCs 3 via electrostatic interactions, altering the LMMCT process without destroying the structure of dLys-AgNCs 3. The interaction mechanism of dLys-AgNCs 2 with S2− is the same as that of dLys-AgNCs 3 at low S2− concentrations and the same as that of dLys-AgNCs 1 at high S2− concentrations.
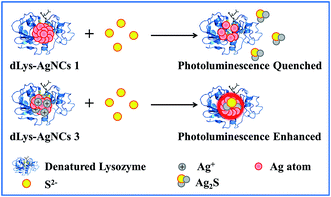 |
| Scheme 1 Schematic illustration of the interaction mechanisms of dLys-AgNCs 1 and 3 with S2−. | |
3.3 Photoluminescence sensing of S2− with dLys-AgNCs 1 based on turn-off mode and dLys-AgNCs 3 based on turn-on mode
Based on the photoluminescence responses of dLys-AgNCs 1 and 3 to S2−, these two fluorescent compounds were used for S2− detection. Fig. 8A shows that the photoluminescence of dLys-AgNCs 1 was gradually quenched when the concentration of S2− increased (0, 0.5, 1, 2, 4, 6, 8, 10, 12, 14, and 16 μmol L−1). The inset figure shows the relationship between the changes in the photoluminescence intensity of dLys-AgNCs 1 and the concentration of S2−. A good linear relationship was obtained between (I − I0)/I0 and the S2− concentration, and the linear regression equation was expressed as (I − I0)/I0 = −0.05375CS2−, with a correlation coefficient of 0.9982 and a limit of detection (LOD) of 0.2 μmol L−1. For dLys-AgNCs 3 (Fig. 8B), the photoluminescence of dLys-AgNCs 3 was enhanced when the concentration of S2− increased (0, 1, 2, 3, 4, 5, 7, 9, 11, and 12 μmol L−1). The resulting relationship between the photoluminescence intensity changes of dLys-AgNCs 3 and the concentration of S2− was expressed as (I − I0)/I0 = 0.02140CS2−, with correlation coefficient of 0.9985 and a LOD of 0.6 μmol L−1.
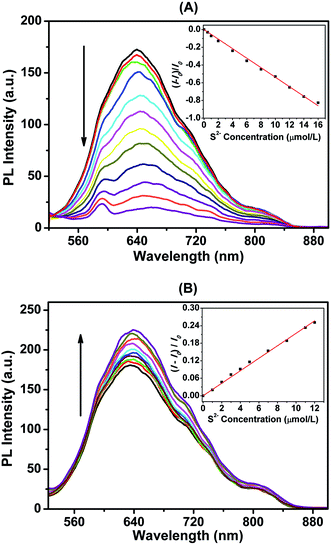 |
| Fig. 8 Photoluminescence spectra of (A) dLys-AgNCs 1 and (B) dLys-AgNCs 3 in the presence of different concentrations of S2−. Inset graph: linear relationship between photoluminescence intensity changes of (A) dLys-AgNCs 1 or (B) dLys-AgNCs 3 and different concentrations of S2−. I0 and I are the photoluminescence intensity of dLys-AgNCs 1 or dLys-AgNCs 3 in the absence and presence of S2−, respectively. The arrow indicates the signal changes as the concentration of S2− increases. | |
To demonstrate the potential applications of the proposed method for S2− detection, assays of S2− in tap water and lake water were conducted. Using dLys-AgNCs 1 as a fluorescent probe (Table 2) showed that there was no detectable S2− in the two water samples, and the recovery rates of standard addition for S2− were in the range of 97.0% to 107%. Moreover, the relative standard deviations were between 2.34% and 6.86%. For dLys-AgNCs 3, the added recovery rates of S2− were in the range of 93.8% to 105%, and the relative standard deviations were between 2.22% and 6.48% (Table 3). These results suggested that dLys-AgNCs 1 and 3 could be used to detect S2− in real samples.
Table 2 Determination of S2− in water samples using dLys-AgNCs 1
Sample |
Detected (μmol L−1) |
Added (μmol L−1) |
Found (μmol L−1) |
Recovery (%) |
RSD, n = 3 (%) |
Not detected. |
Tap water |
—a |
2.0 |
2.03 |
102 |
2.34 |
—a |
6.0 |
5.87 |
97.8 |
5.95 |
—a |
10.0 |
10.3 |
103 |
6.86 |
Lake water |
—a |
2.0 |
2.13 |
107 |
4.61 |
—a |
6.0 |
5.82 |
97.0 |
5.63 |
—a |
10.0 |
9.81 |
98.1 |
2.60 |
Table 3 Determination of S2− in water samples using dLys-AgNCs 3
Sample |
Detected (μmol L−1) |
Added (μmol L−1) |
Found (μmol L−1) |
Recovery (%) |
RSD, n = 3 (%) |
Not detected. |
Tap water |
—a |
2.0 |
2.09 |
104 |
2.22 |
—a |
6.0 |
5.74 |
95.6 |
4.80 |
—a |
10.0 |
9.69 |
96.9 |
4.65 |
Lake water |
—a |
2.0 |
2.11 |
105 |
3.25 |
—a |
6.0 |
5.91 |
98.5 |
6.48 |
—a |
10.0 |
9.38 |
93.8 |
2.57 |
4. Conclusions
Three red-emissive water-soluble lysozyme-stabilized Ag nanoclusters were synthesized, of which dLys-AgNCs 1 contained only Ag(0), whereas dLys-AgNCs 2 and 3 contained both Ag(0) and Ag(I). The photoluminescence properties of dLys-AgNCs 1–3 and their photoluminescence responses to common inorganic anions were studied. dLys-AgNCs 1–3 had a distinct response mode to S2−. S2− quenched the photoluminescence of dLys-AgNCs 1 by forming Ag2S and destroying the nanostructure of dLys-AgNCs 1. In contrast, S2− enhanced the photoluminescence of dLys-AgNCs 3 by binding to Ag(I) contained in dLys-AgNCs 3 and altering the LMMCT process. For dLys-AgNCs 2, the photoluminescence was enhanced at low S2− concentrations and quenched at high S2− concentrations, because S2− bound to Ag(I) at low concentrations and then reacted with Ag(0) at high concentrations. I− quenched the photoluminescence of dLys-AgNCs 1–3 moderately and other anions had no obvious effects. The interaction mechanisms were confirmed by MS, zeta potential, DLS and fluorescence lifetime measurements. As-synthesized dLys-AgNCs 1 and 3 could be used as fluorescent turn-off and turn-on probes for S2− sensing, respectively. This work showed that the composition of the synthesized nanoclusters was affected by the ratio of reactants during synthesis and had a distinct effect on their response mode. These results provide insights into the synthesis strategy and guidance on the design of fluorescent probes for different response modes.
Acknowledgements
This project was supported by the National Natural Science Foundation of China (No. 20905008), and the Fundamental Research Funds for the Central Universities.
References
- I. Diez and R. H. A. Ras, Nanoscale, 2011, 3, 1963–1970 RSC.
- X. Jia, J. Li, L. Han, J. Ren, X. Yang and E. Wang, ACS Nano, 2012, 6, 3311–3317 CrossRef CAS PubMed.
- N. Zhang, Y. Si, Z. Sun, L. Chen, R. Li, Y. Qiao and H. Wang, Anal. Chem., 2014, 86, 11714–11721 CrossRef CAS PubMed.
- L. Lai, C. Zhao, X. Li, X. Liu, H. Jiang, M. Selke and X. Wang, RSC Adv., 2016, 6, 30081–30088 RSC.
- Y. Tao, M. Li, J. Ren and X. Qu, Chem. Soc. Rev., 2015, 44, 8636–8663 RSC.
- J. Wang, J. Ye, H. Jiang, S. Gao, W. Ge, Y. Chen, C. Liu, C. Amatore and X. Wang, RSC Adv., 2014, 4, 37790–37795 RSC.
- L. Zhang and E. Wang, Nano Today, 2014, 9, 132–157 CrossRef CAS.
- J. Sun, J. Zhang and Y. Jin, J. Mater. Chem. C, 2013, 1, 138–143 RSC.
- J. Zhang, Y. Yuan, G. Liang, M. N. Arshad, H. A. Albar, T. R. Sobahi and S. H. Yu, Chem. Commun., 2015, 51, 10539–10542 RSC.
- J. P. Vanegas, E. Zaballos-Garcia, M. Gonzalez-Bejar, P. Londono-Larrea and J. Perez-Prieto, RSC Adv., 2016, 6, 17678–17682 RSC.
- N. Cathcart, P. Mistry, C. Makra, B. Pietrobon, N. Coombs, M. Jelokhani-Niaraki and V. Kitaev, Langmuir, 2009, 25, 5840–5846 CrossRef CAS PubMed.
- Y. W. Zhou, C. M. Li, Y. Liu and C. Z. Huang, Analyst, 2013, 138, 873–878 RSC.
- K. Ma, Y. Shao, Q. Cui, F. Wu, S. Xu and G. Liu, Langmuir, 2012, 28, 15313–15322 CrossRef CAS PubMed.
- X. Yuan, Z. Luo, Q. Zhang, X. Zhang, Y. Zheng, J. Y. Lee and J. Xie, ACS Nano, 2011, 5, 8800–8808 CrossRef CAS PubMed.
- X. Le Guével, V. Trouillet, C. Spies, G. Jung and M. Schneider, J. Phys. Chem. C, 2012, 116, 6047–6051 Search PubMed.
- C. J. Yu, T. H. Chen, J. Y. Jiang and W. L. Tseng, Nanoscale, 2014, 6, 9618–9624 RSC.
- H. Cao, Z. Chen, H. Zheng and Y. Huang, Biosens. Bioelectron., 2014, 62, 189–195 CrossRef CAS PubMed.
- C. Wang, C. Wang, L. Xu, H. Cheng, Q. Lin and C. Zhang, Nanoscale, 2014, 6, 1775–1781 RSC.
- C. A. Chen, C. C. Wang, Y. J. Jong and S. M. Wu, Anal. Chem., 2015, 87, 6228–6232 CrossRef CAS PubMed.
- D. E. Cliffel, F. P. Zamborini, S. M. Gross and R. W. Murray, Langmuir, 2000, 16, 9699–9702 CrossRef CAS.
- Z. Yuan, N. Cai, Y. Du, Y. He and E. S. Yeung, Anal. Chem., 2014, 86, 419–426 CrossRef CAS PubMed.
- F. Qu, N. B. Li and H. Q. Luo, Langmuir, 2013, 29, 1199–1205 CrossRef CAS PubMed.
- J. X. Dong, F. Qu, N. B. Li and H. Q. Luo, RSC Adv., 2015, 5, 6043–6050 RSC.
- W. Guo, J. Yuan, Q. Dong and E. Wang, J. Am. Chem. Soc., 2010, 132, 932–934 CrossRef CAS PubMed.
- C. M. Ritchie, K. R. Johnsen, J. R. Kiser, Y. Antoku, R. M. Dickson and J. T. Petty, J. Phys. Chem. C, 2007, 111, 175–181 CAS.
- L. Zhang, J. Zhu, S. Guo, T. Li, J. Li and E. Wang, J. Am. Chem. Soc., 2013, 135, 2403–2406 CrossRef CAS PubMed.
- X. Yuan, Y. Tay, X. Dou, Z. Luo, D. T. Leong and J. Xie, Anal. Chem., 2013, 85, 1913–1919 CrossRef CAS PubMed.
- T. Zhou, Y. Huang, W. Li, Z. Cai, F. Luo, C. J. Yang and X. Chen, Nanoscale, 2012, 4, 5312–5315 RSC.
- A. Patel and T. Mohanty, J. Mater. Sci., 2014, 49, 2136–2143 CrossRef CAS.
- C. Guo and J. Irudayaraj, Anal. Chem., 2011, 83, 2883–2889 CrossRef CAS PubMed.
- J. Xie, Y. Zheng and J. Y. Ying, J. Am. Chem. Soc., 2009, 131, 888–889 CrossRef CAS PubMed.
- Y. H. Lin and W. L. Tseng, Anal. Chem., 2010, 82, 9194–9200 CrossRef CAS PubMed.
- A. R. Garcia, I. Rahn, S. Johnson, R. Patel, J. Guo, J. Orbulescu, M. Micic, J. D. Whyte, P. Blackwelder and R. M. Leblanc, Colloids Surf., B, 2013, 105, 167–172 CrossRef CAS PubMed.
- H. Kawasaki, K. Hamaguchi, I. Osaka and R. Arakawa, Adv. Funct. Mater., 2011, 21, 3508–3515 CrossRef CAS.
- L. Zou, W. Qi, R. Huang, R. Su, M. Wang and Z. He, ACS Sustainable Chem. Eng., 2013, 1, 1398–1404 CrossRef CAS.
- F. Wen, Y. Dong, L. Feng, S. Wang, S. Zhang and X. Zhang, Anal. Chem., 2011, 83, 1193–1196 CrossRef CAS PubMed.
- P. L. Xavier, K. Chaudhari, P. K. Verma, S. K. Pal and T. Pradeep, Nanoscale, 2010, 2, 2769–2776 RSC.
- Z. Wu and R. Jin, Nano Lett., 2010, 10, 2568–2573 CrossRef CAS PubMed.
- I. Diez, R. H. A. Ras, M. I. Kanyuk and A. P. Demchenko, Phys. Chem. Chem. Phys., 2013, 15, 979–985 RSC.
- Y. Chen, T. Yang, H. Pan, Y. Yuan, L. Chen, M. Liu, K. Zhang, S. Zhang, P. Wu and J. Xu, J. Am. Chem. Soc., 2014, 136, 1686–1689 CrossRef CAS PubMed.
- G. Yang, L. Wu, B. Jiang, W. Yang, J. Qi, K. Cao, Q. Meng, A. K. Mustafa, W. Mu, S. Zhang, S. H. Snyder and R. Wang, Science, 2008, 322, 587–590 CrossRef CAS PubMed.
- L. Ferrer, G. de Armas, M. Miro, J. M. Estela and V. Cerda, Analyst, 2005, 130, 644–651 RSC.
- M. Liang, Y. Chen, H. Zhang, X. Niu, L. Xu, C. Ren and X. Chen, Analyst, 2015, 140, 6711–6719 RSC.
- L. Jin, Z. Zhang, A. Tang, C. Li and Y. Shen, Biosens. Bioelectron., 2016, 79, 108–113 CrossRef CAS PubMed.
- T. Zhou, M. Rong, Z. Cai, C. J. Yang and X. Chen, Nanoscale, 2012, 4, 4103–4106 RSC.
- W. Y. Chen, G. Y. Lan and H. T. Chang, Anal. Chem., 2011, 83, 9450–9455 CrossRef CAS PubMed.
- M. J. Ying, C. P. Cheng and C. H. Tsung, Nanotechnology, 2014, 25, 195502 CrossRef PubMed.
- Z. Li, S. Guo and C. Lu, Analyst, 2015, 140, 2719–2725 RSC.
- X. Liu, R. Hu, Z. Gao and N. Shao, Langmuir, 2015, 31, 5859–5867 CrossRef CAS PubMed.
- M. Esmaeili, S. S. Madaeni, J. Barzin and N. Yousefimehr, Sep. Purif. Technol., 2011, 81, 371–383 CrossRef CAS.
- F. Qu, N. B. Li and H. Q. Luo, Anal. Chem., 2012, 84, 10373–10379 CrossRef CAS PubMed.
- J. Zeng, Y. Cao, C. H. Lu, X. D. Wang, Q. Wang, C. Y. Wen, J. B. Qu, C. Yuan, Z. F. Yan and X. Chen, Anal. Chim. Acta, 2015, 891, 269–276 CrossRef CAS PubMed.
- W. Ding, S. Huang, L. Guan, X. Liu and Z. Luo, RSC Adv., 2015, 5, 64138–64145 RSC.
Footnote |
† Electronic supplementary information (ESI) available. See DOI: 10.1039/c6ra07827c |
|
This journal is © The Royal Society of Chemistry 2016 |