DOI:
10.1039/C6RA04443C
(Paper)
RSC Adv., 2016,
6, 54382-54393
Ferricyanide-based analysis of aqueous lignin suspension revealed sequestration of water-soluble lignin moieties†
Received
19th February 2016
, Accepted 2nd June 2016
First published on 2nd June 2016
Abstract
This study describes the application of a ferricyanide-based assay as a simple and inexpensive assay for rapid analysis of aqueous lignin samples. The assay measures the formation of Prussian blue from the redox reaction between a mixture of potassium ferricyanide and ferric chloride, and phenolic hydroxyl groups of lignin or lignin-derived phenolic moieties. This study revealed that soluble lignin moieties exhibited stronger ferricyanide reactivity than insoluble aggregates. The soluble lignin moieties exhibited higher ferricyanide reactivity because of increased access of the phenolic hydroxyl groups to the ferricyanide reagents. Ferricyanide reactivity of soluble lignin moieties correlated inversely with the molecular weight distributions of the molecules, probably due to the involvement of phenolic hydroxyl groups in bond formation. The insoluble lignin aggregates exhibited low ferricyanide reactivity due to sequestration of the phenolic hydroxyl groups within the solid matrix. The study also highlighted the sequestration of polydispersed water-soluble lignin moieties by insoluble aggregates. The sequestered moieties were released by treatment with 0.01 M NaOH at 37 °C for 180 min. The redox assay was effective on different types of lignin extracts such as Klason lignin from switchgrass, ionic-liquid derived lignin from Eucalyptus and alkali lignin extracts. The assay generated a distinct profile for each lignin sample that was highly reproducible. The assay was also used to monitor consumption of syringic acid by Sphingobium sp. SYK-6. The simplicity and reproducibility of this assay makes it an excellent and versatile tool for qualitative and semi-quantitative characterization and comparative profiling of aqueous lignin samples.
Introduction
Lignin analysis is often complicated by the amphiphilicity1–4 of its moieties that possess both hydrophilic and hydrophobic functional groups. The amphiphilic properties of lignin, a complex heterogeneous biopolymer composed of phenylpropanoids,5–8 confer varying degrees of solubility in aqueous and organic solvents. Lignin analysis has increasingly received attention because of the vast potential of the biopolymer as a renewable source of biofuels and chemicals. Lignin consists primarily of a complex array of p-hydroxyphenyl (H), guaiacyl (G) and syringyl (S) sub-units6,8,9 that are irregularly linked by C–C and C–O (ether) bonds. Despite its potential, conversion of lignin into high-value bio-products (valorization) has been impeded by its recalcitrance to chemical and biological conversion, as well as the limited availability of simple and routine tools for its analysis.
The lignin content of most plant biomass is commonly determined using gravimetric and non-gravimetric assays10–19 such as acid-insoluble (Klason) lignin, milled wood lignin (MWL) and acetyl bromide lignin assays. The properties of the lignin moieties such as chemical bonds and molecular weight distribution are characterized via FT-IR,20,21 FT-RAMAN,22 NMR,3,14,23–26 LC-MS, pyro-GC-MS. MALDI-TOF-MS22,27,28 and size exclusion chromatography (SEC) based analyses. Although, these methods are generally suitable for analysis of lignin from diverse sources, their effectiveness on lignin samples generated from biological processes decreases substantially. The presence of microbes or enzymes in these biological samples further increases the complexity of lignin, hence requiring the development of effective strategies for removing the biological agents without compromising the residual lignin and the associated by-products from the treatments. Most studies evaluate biological lignin degradation indirectly by analyzing the activities of secreted enzymes such as peroxidases6,29 and laccases30 or the release of metabolites and/or CO2 (ref. 31 and 32) generated during microbial growth on lignin substrates. The enzyme assays are mostly carried out using monomeric moieties9,33 because of the difficulty in demonstrating depolymerization of polymeric lignin moieties. A study recently described a strategy for demonstrating microbial lignin degradation by measuring the release of fluorescent adducts and nitrate groups34 from synthetically modified lignin substrate.
Current lignin analytical methods are usually low throughput, laborious, and time consuming, hence, generally unsuitable for routine application. In addition, these methods also tend to involve the use of expensive instrumentation, and often require extensive and complicated computational and statistical analyses. These challenges have led to increased demand for the development of simple assays or strategies for rapid and routine characterization of lignin before employing more sophisticated analyses. It is also very critical to develop aqueous-based analytical strategies2,35,36 for lignin analysis considering that most biological and some chemical lignin analyses are carried out under aqueous conditions. Aqueous lignin suspensions are typically a mixture of soluble and insoluble moieties1–4 that are often difficult to analyze. Therefore, it is imperative to develop analytical strategy and methods to characterize soluble and insoluble constituents of aqueous lignin suspension prior to biological treatment.
This study evaluates the suitability of ferricyanide-based reduction–oxidation (redox) assay as a simple, rapid and inexpensive assay for routine analysis of aqueous lignin. This ferricyanide assay37–42 is a two-step reaction that oxidizes the hydroxyl groups of lignin or phenolic moieties generating Prussian blue39,41 which absorbs strongly in the visible region above 700 nm from. The first step of the reaction involves generation of ferrous (Fe2+) ion37,38 from oxidation/deprotonation of the hydroxyl groups (C–OH) of phenolic lignin moieties by ferric ion (Fe3+) from ferric chloride (FeCl3·6H2O). The ferrous (Fe2+) ion subsequently reacts with potassium ferricyanide (K3[Fe(CN)6]) generating potassium ferric/ferrocyanide (KFe3+[Fe2+(CN)6]) complex or Prussian blue. This redox assay has been widely used to evaluate the antioxidant properties of biological reducing agents39–41,43,44 such as ascorbic acid and polyphenols in fruits and vegetables. It also used in cell biology37,44–46 to study the activities of reductases against reactive oxygen species (ROS). The assay can also be used to screen for potential lignin degrading microbial species on lignin agar plates.45
Here we describe a ferricyanide assay-based strategy for comparative analysis of aqueous suspensions of lignin from Eucalyptus, switchgrass (Panicum virgatum) and technical alkali lignin. The assay highlighted distinctive profiles for soluble and insoluble components of the lignin suspensions. The soluble lignin moieties were comparatively more reactive compared to the insoluble moieties regardless of their source. Further analysis of the insoluble aggregates revealed evidence of sequestration of soluble lignin moieties of varying size.
Results and discussion
Ferricyanide reactivity of lignin phenolic hydroxyl groups is strongly enhanced by methoxylation
Aqueous solutions (0.05 mg mL−1) of p-toluic acid (TA) and four phenolic monomers (ESI Fig. S1†) were analyzed to validate the role of lignin phenolic hydroxyl groups in ferricyanide assay. The reaction with p-toluic acid did not yield Prussian blue compared the phenolic monomers that exhibited varying degrees of ferricyanide reactivity (Table 1 and ESI Fig. S1†). The ferricyanide activity (Abs750/μmol) of 4-methylsalicylic acid (MSA) and 4-hydroxybenzoic acid (HBA) were 16- and 30-fold respectively, higher than the basal activity of p-toluic acid (Table 1). The ferricyanide reactivity of MSA which differs from p-toluic acid by the presence of hydroxyl group at the 2-position validated the critical role of phenolic hydroxyl group in the assay.
Table 1 Ferricyanide reactivity of lignin derived monomers
Substrate |
Amount (μmol) |
Ferricyanide reactiona |
Activityb,c,d (Abs750) |
Normalized activity (Abs750/μmol) |
100 μL of 0.1 mg mL−1 solution of the substrate were diluted with 100 μL of DI-water prior to the reactions which was initiated by the addition of 10 μL 1% ferricyanide reagent.
The absorbance unit of each reaction was normalized by subtracting the absorbance of control reaction with DI-water and multiplied by the dilution factor (2.1).
Prior to application of the dilution factor, the direct absorbance of the DI-water control at 750 nm was 0.32 ± 0.01 compared 0.34 ± 0.08 and 11.83 ± 0.13 for p-toluic acid and syringic acid respectively.
Abs750 = absorbance at 750 nm wavelength.
|
Syringic acid |
0.050 |
24.17 ± 0.27 |
478.90 |
Vanillic acid |
0.059 |
23.28 ± 0.19 |
391.35 |
p-Toluic acid |
0.073 |
0.04 ± 0.17 |
0.56 |
4-Methylsalicylic acid |
0.066 |
0.61 ± 0.15 |
9.24 |
4-Hydroxybenzoic acid |
0.072 |
1.24 ± 0.14 |
17.12 |
Ferricyanide assay was also enhanced by the presence of methoxy group in the phenolic ring. The positive influence of methoxylation on ferricyanide assay was highlighted by 23- and 28-fold increase in activities of vanillic acid (VA) and syringic acid (SA) respectively, compared to HBA (Table 1). The appearance of Prussian blue in the reaction with VA and SA representing G- and S-lignin moieties8,46 respectively was very rapid (in seconds). The high ferricyanide reactivity of VA and SA was attributed to the influence of the electro-negative oxygen atom of the methoxy groups on ionization or deprotonation of adjacent phenolic hydroxyl groups as previously reported.47–49 The reactivity of VA and SA also correlated with the reported47 oxidation potential (Epa) of their phenolic hydroxyl groups (0.73 and 0.49 V respectively) relative to HBA (0.87).
The linearity of the assay was investigated using 0.012–0.24 mM solution (DI-water) of syringic acid. The assay was observed to be linear up to 0.12 mM concentration (Fig. 1A).
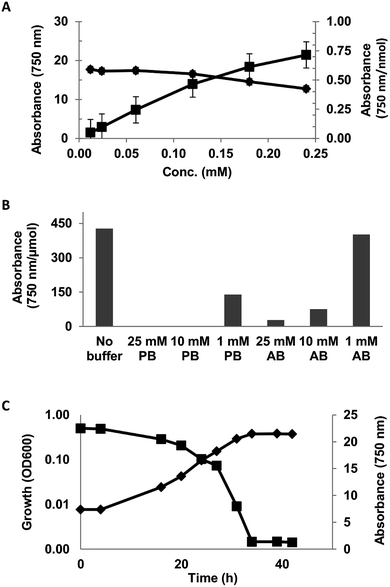 |
| Fig. 1 Ferricyanide assay characterization of syringic acid. (A) Plot of absorbance (750 nm) of ferricyanide reaction with different concentrations of syringic acid (activity) against normalized activity per nmol of substrate [absorbance (750 nm nmol−1). (B) Effect of 1, 10 and 25 mM phosphate (pH = 6.5) and acetate (pH = 5.5) buffers on ferricyanide reactivity of 0.05 mg mL−1 syringic acid and (C) ferricyanide reactivity of residual substrate during growth of Sphingobium sp. SYK-6 on 0.5 g L−1 syringic acid. OD600 = light scattering at 600 nm wavelength. | |
The effect of reaction matrix on the assay was evaluated with 0.05 mg mL−1 solution of syringic acid in 1, 10 and 25 mM phosphate (pH = 6.5) and acetate (pH = 5.5) buffers. The reactivity of syringic acid was completely inhibited in 10 and 25 mM phosphate buffer solutions and decreased by 67% in the 1 mM solution (Fig. 1B). The effect of acetate buffer was comparatively less severe, as the reactivity of SA in 1, 10 and 25 mM of the buffer decreased by 6, 82 and 93% respectively (Fig. 1B). Ferricyanide reactivity of SA in the minimal salt medium (MSM) described in ESI Table S1† exhibited an inhibitory effect that was similar to phosphate buffer (data not shown). A screen of the individual components of the medium identified mono- and dibasic potassium phosphate as the inhibitory compounds. The assay was also used to monitor the consumption of syringic acid by Sphingobium sp. SYK6 in MSM broth (Fig. 1C). The adverse effect of the inorganic phosphates in the growth medium on the assay was mitigated by making a 10-fold dilution of 3000 MWCO filtrates of the culture supernatant prior to analysis. Consumption of the syringic acid in the growth medium was highlighted by 94% decrease in the relative absorbance (Abs750) of Prussian blue produced from the reaction from inoculation to stationary growth phase (Fig. 1C). These results suggest the lignin moieties which are generally rich in S- and G-lignin (≥90%)6,50–52 would exhibit strong ferricyanide reactivity.
Soluble moieties in aqueous lignin suspension exhibit stronger ferricyanide reactivity
Ferricyanide reactivity of lignin was investigated using size fractionated (≤20 μm particles) aqueous suspensions of ionic-liquid derived lignin53 from Eucalyptus and Klason lignin12 from switchgrass (see Experimental section). The reaction was initiated by adding 10 μL of 1% ferricyanide reagent to 200 μL of total lignin suspension (TLS) from each extracts and incubated at room temperature for 30 min. The ferricyanide activity of the samples was determined by measuring the absorbance of Prussian blue produce from each reaction at 750 nm wavelength (Abs750). The contribution of soluble and insoluble lignin moieties to the reactivity of the TLS fractions was determined by analyzing the supernatant (SUP) and residual pellet (PEL) of each extract.
The results highlighted distinctive Abs750 profile for TLS, SUP and PEL fractions that distinguished the Eucalyptus from the switchgrass lignin extract (Fig. 2). The SUP fractions of lignin extracts exhibited higher ferricyanide reactivity than the corresponding PEL fractions (Fig. 2). The Eucalyptus SUP fraction accounted for 83% of the ferricyanide activity (Abs750) of the TLS, compared 51% activity contributed by the SUP from switchgrass (Fig. 2). The ferricyanide activity of the TLS fractions was fairly equal to the sum of the activities of the corresponding SUP and PEL fractions (Fig. 2). The high ferricyanide reactivity of the SUP fraction suggested that soluble lignin moieties were more reactive than insoluble moieties. The less reactive PEL fraction contained more aromatic and lignin particles based on the absorbance at 280 nm (Abs280) and 600 nm (Abs600) respectively prior to the assay. The lower reactivity of the PEL fractions correlated with the removal of soluble lignin moieties from the pellets (Fig. 3). The peaks of soluble lignin moieties in the SUP fraction from Eucalyptus were visibly absent in the size exclusion chromatography (SEC) profile of the three washes from the PEL fraction (Fig. 3). Similarly, the ferricyanide activities of the three PEL washed were relatively 2–3% of the corresponding SUP activity (ESI Fig. S2†).
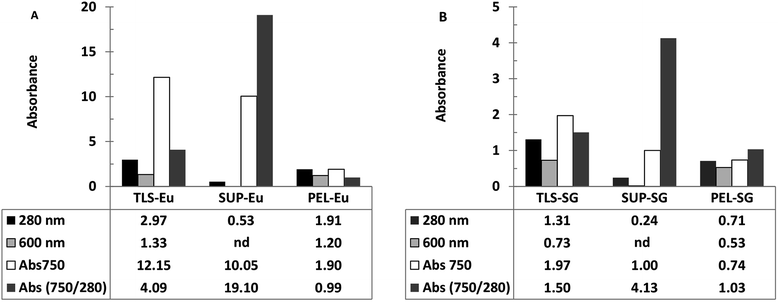 |
| Fig. 2 Ferricyanide analysis of lignin extracts from Eucalyptus (Eu) and switchgrass (SG). Profiles ferricyanide reactions with total lignin suspension (TLS), supernatant (SUP) containing soluble lignin moieties and re-suspended pellet (PEL) containing insoluble lignin aggregate from Eucalyptus (A) and switchgrass (B). The supernatant was filtered through 0.45 μm filter. The re-suspended pellet was washed three times with DI-water. The absorbance of each substrate was measured at 280 and 600 nm prior to ferricyanide analysis. Note: nd = not detected. | |
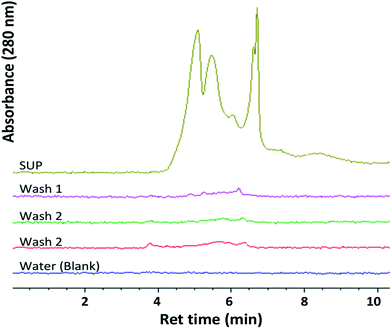 |
| Fig. 3 Size exclusion chromatography (SEC) profile of soluble lignin moieties present in the supernatant (SUP) of aqueous lignin suspension from Eucalyptus and washes (Wash 1–3) of the resulting pellet. Each sample was filtered through 0.45 μm filter. Water blank was used as reference. | |
The higher reactivity of the SUP fractions was reaffirmed by normalization of the ferricyanide activity (Abs750) with the corresponding substrate absorbance at 280 nm (Abs750/280). The normalized Abs750/280 activity of Eucalyptus SUP was ∼5-fold greater than the switchgrass equivalent (Fig. 2). In comparison, there was no observable difference in the normalized Abs750/280 activity of the insoluble PEL fractions from Eucalyptus and switchgrass (Fig. 2). The normalized Abs750/600 activity of the PEL fractions from Eucalyptus (1.56) and switchgrass (1.40) also highlighted the similarity in the ferricyanide reactivity of insoluble lignin particles. The higher ferricyanide reactivity of lignin from Eucalyptus was attributed to the reported11,52,54,55 high S/G ratio of the moieties compared to switchgrass extracts. The disparity in the ferricyanide reactivity of Eucalyptus and switchgrass lignin extracts could also be attributed to the mode of extraction of the lignin moieties.
The low reactivity of insoluble lignin aggregates (Fig. 2) potentially limit the application of the assay as a quantitative tool for comparative analysis of aqueous lignin extracts. Ferricyanide assay is comparable to non-gravimetric techniques such as acetyl-bromide10–19 that estimate lignin content in plant biomass. The acetyl-bromide method solubilizes plant biomass15,19,56,57 and estimates lignin content by directly measuring the absorbance of aromatics moieties at 280 nm. In comparison, ferricyanide assay estimates lignin content of samples by indirectly measuring the reactivity of the phenolic hydroxyl groups58,59 of lignin moieties. The assay does not solubilize or homogenize insoluble lignin aggregates as highlighted by the differential reactivity of the soluble and insoluble fractions (Fig. 2). However, a combination of the normalized ferricyanide activity (Abs750/280) profiles of the TLS, SUP and PEL fractions (Fig. 2) generates a unique and reproducible fingerprint for each lignin suspension. This distinctive fingerprint could be effectively used as a qualitative tool for screening and comparative analysis of different lignin extracts. The potential of the assay as a quantitative tool was highlighted by the linear calibration curves obtained from reactions with the TLS, SUP and PEL fractions (ESI Fig. S3†). However, the effectiveness of ferricyanide assay as a quantitative technique would be strongly influenced by availability and accessibility of phenolic hydroxyl groups51,55,60,61 of the lignin extracts. Generally, quantitative application of non-gravimetric techniques such as ferricyanide assay is undermined by the absence of lignin standards17,60,61 that are representative of all lignin extracts. Studies have shown that the use a ‘model lignin’ standard for lignin quantification17,57,60 generates results that are often not reproducible. Lignin quantification is further complicated51,60,61 by the heterogeneity of the polymer, variation in biomass and mode of extraction.
Ferricyanide reactivity soluble lignin correlates inversely with the molecular weight of the moieties
The effect of the molecular weight distribution on the ferricyanide reactivity of soluble lignin moieties was investigated by filtering the supernatant of the Eucalyptus suspension through 0.2 μm Steri-flip filter (Millipore, Billerica, MA). Aliquots (0.5 mL) of the 0.2 μm filtrate (SUP-L0.2μ) were filtered through 3, 10, 30 and 100 kDa cut-off Amicon-Ultra filters (Millipore, Billerica, MA) to remove high molecular weight (HMW) moieties. The filtrates and SUP-L0.2μ were subjected to ferricyanide analysis in triplicates as previously described. The molecular weight distribution profile and aromatic content of each sample were determined via SEC and absorbance at 280 nm respectively. The results highlighted the exclusion of HMW (≥100 kDa) moieties (Fig. 4A) from each ultra-filtered sample that corresponded to 26–40% decrease in the total aromatics (Abs280) from SUP-L0.2μ (Fig. 4B). Interestingly, the removal of ∼40% of total aromatics from the 3 kDa filtrate (SUP-L3) resulted in <6% decrease in its ferricyanide activity compared to SUP-L0.2μ (Fig. 4B). This observation suggested that the low molecular weight (LMW) lignin moieties (≤3 kDa) in SUP-L0.2μ were responsible for most of its ferricyanide reactivity (Fig. 4B). The inverse correlation between the size of lignin moieties and ferricyanide reactivity was further highlighted by Abs750/280 activity of SUP-L0.2μ and the filtrates (Fig. 4B). The Abs750/280 activity of SUP-L3 filtrate also increased by ∼52% relative to SUP-L0.2μ (Fig. 4B). The Abs750/280 activity of the soluble lignin increased with removal of HMW moieties from each substrate (Fig. 4B), as verified by the SEC profiles (Fig. 4A).
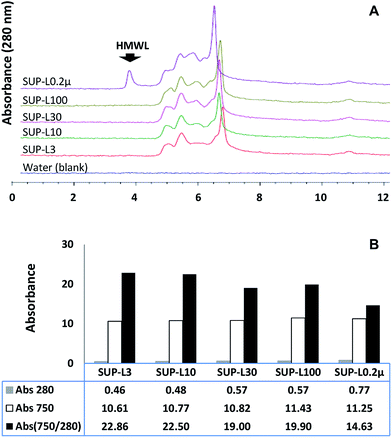 |
| Fig. 4 Characterization of size fractionated soluble lignin moieties from Eucalyptus. (A) Aqueous size exclusion chromatography profile of lignin samples containing water-soluble lignin moieties ≤3 kDa (SUP-L3), ≤10 kDa (SUP-L10), ≤30 kDa (SUP-L30), ≤100 kDa (SUP-L100) and ≤0.2 μm (SUP-L0.2μ). (B) Ferricyanide analysis of each substrate described in (A). Prussian blue produced from each reaction was estimated at 750 nm wavelength (Abs750). The absorbance of each substrate was determined at 280 nm wavelength (Abs280) prior to the analysis. Ferricyanide activity of each sample was normalized per unit substrate absorbance at 280 nm (Abs750/280). HMWL = high molecular weight lignin moieties. | |
The assay was also carried out on 1.0 mg mL−1 aqueous suspensions of alkali lignin (AL) and the low sulfonate (ALS) variant (Sigma-Aldrich, St Louis, MO). Each 1.0 mg mL−1 alkali lignin suspension was incubated at 37 °C for 60 min with occasional mixing prior to ferricyanide analysis in triplicates. The ferricyanide activity (Abs750) of the ALS suspension was observed to be ∼2-fold higher than AL equivalent (Table 2). The low Abs750 activity of AL suspension correlated with its low water solubility. The ∼6-fold higher absorbance of the ALS suspension at 280 nm wavelength (Abs280) relative to AL suspension (Table 2), suggested that it contained more water-soluble lignin aromatic moieties. The correlation between lignin solubility and ferricyanide reactivity was further investigate by filtering 0.6 mL aliquots of the AL and ALS suspensions through 0.45 μm filter (VWR Scientific, Radnor, PA) and 3, 10, 30 and 100 kDa cut-off Amicon-Ultra filters.
Table 2 Ferricyanide analysis of soluble alkali lignin extractsa
Alkali lignin |
Fraction |
Molecular weight |
Substrate aromatic content |
Ferricyanide activity (Abs750) |
Normalized ferricyanide activity (Abs750/280) |
Absorbance (280 nm) |
Relative (%) |
Approximately 0.1% of regular and low sulfonate alkali lignin samples were suspended in DI-water and filtered through 0.45 μm filters. A 1.0 mL aliquot of filtrate from each lignin sample was subsequently filtered through 10, 30 and 100 kDa membrane filters respectively and subjected to ferricyanide analysis as described in the Experimental section.
|
Regular (AL) |
AL-L3 |
≤3 kDa |
0.91 ± 0.00 |
31 |
12.58 ± 0.12 |
13.83 |
AL-L10 |
≤10 kDa |
1.32 ± 0.01 |
45 |
15.80 ± 0.17 |
11.98 |
AL-L30 |
≤30 kDa |
1.63 ± 0.00 |
56 |
19.38 ± 0.22 |
11.91 |
AL-L100 |
≤100 kDa |
1.84 ± 0.00 |
63 |
20.83 ± 0.22 |
11.32 |
AL-L0.45μ |
≤0.45 μm |
1.96 ± 0.00 |
67 |
21.19 ± 0.53 |
10.80 |
AL (1 mg mL−1) |
Total suspension |
2.93 ± 0.00 |
100 |
27.88 ± 0.74 |
9.52 |
Low sulfonate (ALS) |
ALS-L3 |
≤3 kDa |
1.82 ± 0.00 |
11 |
18.19 ± 0.30 |
10.00 |
ALS-L10 |
≤10 kDa |
2.95 ± 0.00 |
17 |
28.00 ± 0.20 |
9.49 |
ALS-L30 |
≤30 kDa |
4.94 ± 0.00 |
29 |
38.98 ± 0.53 |
7.90 |
ALS-L100 |
≤100 kDa |
8.71 ± 0.00 |
51 |
55.07 ± 0.83 |
6.32 |
ALS-L0.45μ |
≤0.45 μm |
16.00 ± 0.01 |
94 |
66.06 ± 0.44 |
4.13 |
ALS (1 mg mL−1) |
Total suspension |
17.10 ± 0.01 |
100 |
66.70 ± 1.41 |
3.90 |
Ferricyanide reactions with the filtrates revealed that the soluble lignin moieties (≤0.45 μm) in AL and ALS suspensions contributed 76 and 99% of their total ferricyanide activity respectively (Table 2). The Abs280 value of the total soluble fraction of ALS suspension (ALS-L0.45μ) was ∼8-fold higher than the AL equivalent (Table 2), suggesting that the extract was rich with lignin aromatic moieties. The normalized Abs750/280 activity of the alkali lignin extracts also revealed an inverse correlation between lignin molecular size and ferricyanide reactivity (Table 2). The effect of exclusion of HMW moieties from the soluble fraction was more severe with ALS extract. Approximately 77% of soluble lignin aromatic moieties (Abs280) in ALS extracts were ≥10 kDa compared to 22% of AL moieties (Table 2). The Abs750/280 of ALS-L0.45μ (Table 2) was ∼59% lesser than the corresponding activity of the 3 kDa filtrate (ALS-L3). The decrease in ferricyanide reactivity of HMW lignin molecules was attributed to reduction in free phenolic hydroxyl groups required for the reaction. The phenolic hydroxyl groups of lignin sub-units are expected to be involved in the formation complex array of ether bonds6,25,47,49,55 such as β-O-4 and 4-O-5 in larger moieties.
In addition, the differences in the ferricyanide reactivity of equal concentrations of AL and ALS alkali lignin suspensions suggested that absolute concentration of lignin was not important for comparative analysis of lignin. The results from this study indicated that molecular weight distribution and physico-chemical properties of the lignin molecules were critical parameters to be considered for effective lignin analysis. The ability of the assay to differential between two technical alkali lignin samples (Table 2) for highlighted the potential of assay as a qualitative tool for lignin analysis. The differential reactivity of the alkali lignin samples also revealed a potential pitfall to the use of model lignin such as dehydrogenative polymers (DHP)62,63 or a single source of lignin extract17 as standard for comparative analysis.
Ferricyanide assay revealed sequestration of water-soluble lignin moieties within insoluble aggregates
The low ferricyanide reactivity of the PEL fractions (Fig. 2) was attributed to limited access to phenolic hydroxyl groups that are sequestered within insoluble lignin aggregates. The hypothesis was investigated by treating PEL fraction from Eucalyptus with NaOH as previously reported,1,2 to solubilize the lignin aggregates. Three PEL fractions were generated from 1.0 mL aliquots of Eucalyptus TLS as described in the Experimental section. The three SUP fractions were filtered through 0.45 μm membrane filter to remove insoluble lignin moieties. The PEL fractions were treated with 300 μL DI-water (PEL-W), 0.01 M NaCl (PEL-NaCl) and 0.01 M NaOH (PEL-NaOH) respectively and incubated at 37 °C for 180 min. The PEL-NaOH reaction was neutralized with 300 μL of 0.01 M HCl and adjusted to 1.0 mL with 400 μL of water (Fig. 5). The PEL-W and PEL-NaCl reactions were each adjusted to 1.0 mL with 700 μL of water after incubation (Fig. 5). The treated PEL, the corresponding SUP and the TLS fractions were subjected to ferricyanide assay as previously described. The results showed that the Abs750/280 and Abs750/600 activities of alkali-treated PEL-NaOH increased by ∼3- and 6-fold relative to the water-treated PEL-W (Table 3). There was relatively no observable difference in the ferricyanide reactivity or absorbance of PEL-NaCl and PEL-W substrates at 280 nm or 600 nm (Table 3). In addition to increase ferricyanide reactivity, the absorbance of PEL-NaOH at 280 nm (Abs280) increased by ∼20% and decreased by ∼33% at 600 nm (Abs600) relative to PEL-W (Table 3). The inverse correlation between the absorbance of PEL-NaOH at 280 and 600 nm (Table 3) was attributed to dissolution of insoluble lignin aggregates by alkaline solution as previously reports.2,4,64–70
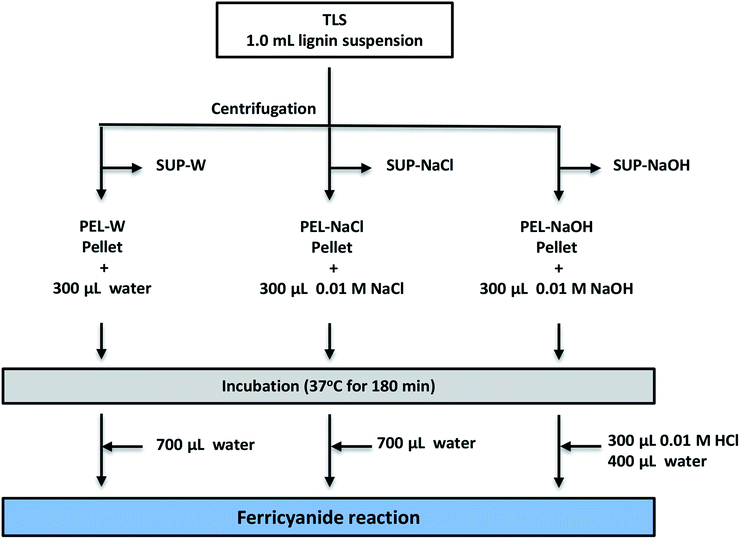 |
| Fig. 5 Schematic diagram of protocol for dissolution of insoluble lignin aggregates from Eucalyptus. Three 1.0 mL aliquots of aqueous total lignin suspension (TLS) were centrifuged to separate soluble lignin moieties (SUP) from insoluble aggregates (PEL). The SUP fractions were filtered through 0.45 μm membrane filter. The PEL extracts were washed three times by re-suspending in 1.0 mL of DI-water and centrifuging to remove residual soluble lignin moieties. The washed PEL extracts were re-suspended in 300 μL of DI-water (PEL-W), 300 μL of 0.01 M NaCl (PEL-NaCl) and 300 μL of 0.01 M NaOH (PEL-NaOH) respectively, and incubated in a water-bath at 37 °C for 180 min with intermittent agitation. PEL-NaOH suspension was neutralized with 0.01 M HCl after incubation. The volume of each treated PEL suspensions were subsequent adjusted to 1.0 mL with DI-water. The soluble fraction of the treated PEL suspensions were also obtained from 0.5 mL aliquot of each sample and filtered through 0.45 μm membrane filter. The treated PEL extracts, the corresponding SUP (SUP-W, SUP-NaCl and SUP-NaOH) and the TLS were subjected to ferricyanide assay and size exclusion chromatography. | |
Table 3 Characterization of insoluble lignin aggregates from Eucalyptusa
Substrate |
Description |
Substrate absorbance |
Ferricyanide reactivity Abs750 |
Normalized ferricyanide activity |
Abs280 |
Abs600 |
Abs750/280 |
Abs750/600 |
Note: nd = not detected, na = not applicable. Each supernatant was filtered through 0.45 μm filter.
|
TLS |
Total lignin suspension from Eucalyptus (≤20 μm) |
3.15 ± 0.00 |
1.40 ± 0.00 |
12.65 ± 0.12 |
4.01 |
9.05 |
SUP-W |
Residual supernatant from pellet used for PEL-W |
0.62 ± 0.00 |
na |
10.29 ± 0.10 |
16.58 |
na |
SUP-NaCl |
Residual supernatant from pellet used for PEL-NaCl |
0.62 ± 0.00 |
na |
10.30 ± 0.04 |
16.52 |
na |
SUP-NaOH |
Residual supernatant from pellet used for PEL-NaOH |
0.61 ± 0.00 |
na |
10.16 ± 0.11 |
16.72 |
na |
PEL-W |
Water-treated residual pellet from TLS |
2.02 ± 0.00 |
1.20 ± 0.00 |
2.14 ± 0.01 |
1.06 |
1.79 |
PEL-NaCl |
NaCl-treated residual pellet from TLS |
1.94 ± 0.00 |
1.16 ± 0.00 |
2.18 ± 0.02 |
1.12 |
1.87 |
PEL-NaOH |
NaOH-treated residual pellet from TLS |
2.43 ± 0.00 |
0.80 ± 0.00 |
7.87 ± 0.14 |
3.24 |
9.88 |
PEL-W-S |
Supernatant from PEL-W after water treatment |
nd |
na |
0.45 ± 0.01 |
na |
na |
PEL-NaCl-S |
Supernatant from PEL-NaCl after NaCl treatment |
nd |
na |
0.51 ± 0.04 |
na |
na |
PEL-NaOH-S |
Supernatant from PEL-NaOH after NaOH treatment |
0.74 ± 0.00 |
na |
5.69 ± 0.04 |
7.66 |
na |
The release of soluble lignin moieties in PEL-NaOH were verified via aqueous SEC analysis (Fig. 6) of its supernatant (PEL-NaOH-S) which contributed ∼72% of its total ferricyanide activity (Table 3). The SEC profile of PEL-NaOH-S highlighted the presence of HMW lignin moieties that were absent in the supernatants of PEL-W and PEL-NaCl (Fig. 6A). The alkaline solubilization of insoluble lignin aggregates was further validated by the absence of the HMW lignin peaks in the SUP associated with each PEL fraction (Fig. 6B).
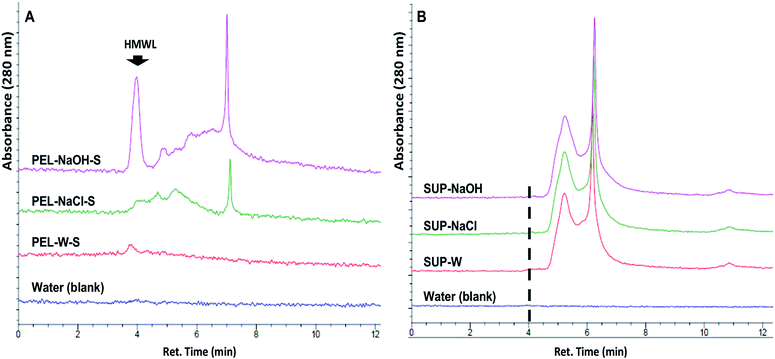 |
| Fig. 6 Size exclusion chromatography (SEC) analysis of alkaline solubilized sequestered lignin moieties from Eucalyptus. (A) SEC profile of 0.45 μm filtered supernatants of insoluble lignin aggregates (PEL) treated with DI-water (PEL-W-S), 0.01 M NaCl (PEL-NaCl-S) and 0.01 M NaOH (PEL-NaOH-S). Each PEL suspension was incubated at 37 °C for 180 min. After incubation PEL-NaOH was neutralized with 0.01 M HCl. Sequestered soluble high molecular weight lignin (HMWL) moieties (ret. time = 3.5–4.5 min) were released from NaOH-treated aggregates. (B) SEC profile of the soluble lignin fractions (SUP) of the total lignin suspension (TLS) that was used for the preparation of the corresponding PEL fraction in (A). The broken line corresponded to the retention time of alkali solubilized high molecular weight lignin moieties released by NaOH treatment. | |
The released soluble lignin moieties by mild alkali treatment of PEL-NaOH suggested that the molecules were sequestered within the insoluble aggregates (Table 3 and Fig. 6). Lignin moieties have been reported to self-assemble into metastable aggregates1,2,35,64,65,70 that potentially limit access to the phenolic hydroxyl groups47,54 of the molecules. The metastable insoluble lignin aggregates are believed to be bound by a combination of hydrophilic and hydrophobic interactions2,70 between the amphiphilic lignin molecules. Solubilization of lignin aggregates was proposed to result from ionization of the lignin molecules by deprotonation of the carboxyl and hydroxyl groups2,47,49 in mild alkaline environment (Fig. 7). The dissociation of the lignin aggregates by mild alkali treatment (Fig. 6A), despite withstanding three autoclaving cycles at 121 °C (15 psi) for 30–35 min (see Experimental section) suggested that the aggregates were not covalently linked.
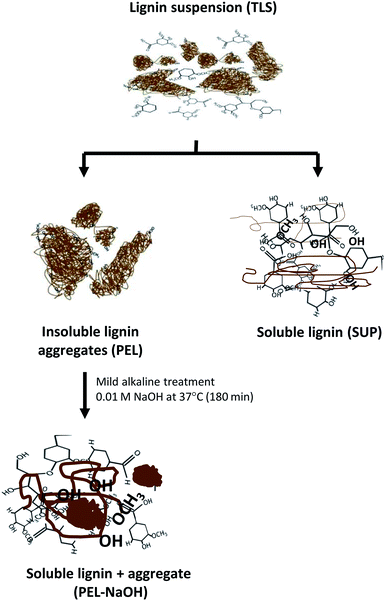 |
| Fig. 7 Schematic representation of proposed mechanism of alkali solubilization of insoluble lignin aggregates. Aqueous lignin suspension (TLS) consists of a mixture of soluble (SUP) and insoluble (PEL) moieties. The PEL fraction sequesters soluble lignin moieties that are released by mild alkali treatment at ambient temperature and pressure. Solubilization of the sequestered lignin moieties was proposed to result from ionization of the hydrophilic functional groups of the lignin moieties. | |
Therefore, the low reactivity of insoluble aggregates in the PEL fractions was concluded to result from limited access to sequestered phenolic hydroxyl groups of the lignin molecules. The results also highlighted the release of substantial amount of sequestered low molecular weight lignin moieties (Fig. 6A). The abiotic release of sequestered soluble lignin moieties observed in this study complicates the interpretation data from biological lignin degradation studies. Most microbial lignin degradation studies often ascribed the release of soluble lignin moieties during growth on insoluble lignin71,72 to degradative activities of the microbes. This study highlights the need re-evaluate the use of microbial growth and release of soluble lignin or CO2 as a proxy for biological lignin depolymerization.
The vast potential of lignin as a renewable alternative to petroleum for production of high-value fuels and chemicals73 has increased the demand to improve our understanding of the complex polymer. The primary impediment to advancing our knowledge of lignin is the inadequate availability of reproducible6,55 analytical tools. Lignin analysis61,74 often involve the use of multiple techniques that estimate total lignin content of biomass10–19 or evaluate physico-chemical characteristics3,14,20–28 of the heterogeneous polymer.
The strategy described in the study provided a simple and reproducible qualitative technique for rapid comparative analysis of lignin content of aqueous samples. The ferricyanide assay and SEC based strategy provided information on solubility, size and reactivity of the moieties in each extracts. The differential reactivity of soluble and insoluble fractions (Fig. 2) highlighted a potential limitation to quantitative application of the assay and other lignin analytical techniques. The normalized ferricyanide activity of (Abs750/280) of the TLS, SUP and PEL aqueous lignin samples highlighted by this study could serve as a useful tool for qualitative comparative analysis.
The assay reproducibly differentiated between lignin extracts from the Eucalyptus and switchgrass (Fig. 2). The assay could also be useful for screening of biotic and abiotically treated lignin samples prior to analysis with sophisticated techniques such as NMR spectroscopy. The throughput and potential automation of the assay was enhanced with the development of a 96-well assay (ESI Fig. S3†).
Conclusion
This study reports the application of a ferricyanide-based redox assay as a simple and reproducible assay for routine analysis of aqueous lignin. The inexpensive redox assay was based on the reaction between lignin phenolic hydroxyl groups and 1% solution of potassium ferricyanide and ferric chloride mixture. The redox assay was effective on different types of lignin extracts such as alkali, Klason and organosolv lignin. The assay generated distinctive profiles for soluble and insoluble fractions of lignin sample evaluated, making it an effective technique for qualitative screening of aqueous lignin samples. The assay was also used to monitor the consumption of syringic acid by Sphingobium sp. SYK6. The study provided evidence for the sequestration of soluble lignin moieties within insoluble lignin aggregates. The sequestered lignin moieties were readily solubilized by mild alkaline treatment via a combination of ferricyanide assay and aqueous SEC analyses. The simplicity and versatility of the assay makes it an excellent tool for comparative qualitative and semi-quantitative analysis of aqueous lignin prior to analysis with more sophisticated techniques such as NMR. However, quantitative application of the assay is limited by complexity and heterogeneity of lignin. For effective application of this ferricyanide assay as a tool for rapid comparative analysis of lignin, the mode of preparation, level of aggregation of the moieties, molecular weight distribution soluble moieties and H/G/S content of the lignin extract must all be taken into consideration. Overall, this study advocated for further development of simple and reproducible analytical tools to improve our understanding of lignin.
Experimental
Source of materials
The Klason lignin extract12 from switchgrass that was used in this study was prepared following the protocol described in the (ESI†). The ionic liquid (1-ethyl-3-methylimidazolium acetate)-derived lignin extract53 from Eucalyptus was provided by the Advanced Biofuels Process Demonstration Unit (ABPDU) at Lawrence Berkeley National Laboratory, Emeryville, CA. The two alkali lignin extracts and benzoic acid derived aromatic monomers were obtained from Sigma-Aldrich, (St. Louis, MO). The Sphingobium sp. SYK-6 (NBRC 103272) strain was obtained from National Institute of Technology and Evaluation, Chiba, Japan.
Ferricyanide assay
The ferricyanide assays described in the study were carried out by adding 10 μL of freshly prepared 1% ferricyanide reagent to 200 μL of aqueous solution/suspension of lignin or lignin-derived aromatic molecules. The ferricyanide reagent was prepared by combining equal volumes of aqueous solutions of 2% ferric chloride hexahydrate and 2% potassium ferricyanide. A reagent control reactions prepared by adding 10 μL of the ferricyanide reagent to 200 μL of DI-water was included in every experiment. The reactions were mixed vigorously and incubated at room temperature for 30 min with occasional mixing. After incubation, the absorbance of 10-fold dilution of each reaction was measured at 750 nm wavelength (Abs750) using a Beckman-Coulter DU-800 spectrophotometer (Brea, CA). The relative Prussian blue production or ferricyanide activity of each substrate was determined by subtracting the mean Abs750 value of the reagent control reaction from each substrate. The assay was also adapted to 96-well micro-plate format to enhance the throughput of the assay. The microplate assay was initiated by adding 10 μL of freshly prepared 1% ferricyanide reagent to 200 μL of appropriate dilutions the substrates in dark-wall 96-well plate and mixed properly. The reaction was incubated room temperature for 30 min with intermittent gently mixing. The absorbance of each sample was measured directly using Spectramax M2 microplate reader (Molecular Devices, Sunnyvale, CA).
Analysis of aromatic monomers
The influence of phenolic hydroxyl and methoxy groups of lignin molecules on ferricyanide assay was evaluated using 0.05 mg mL−1 aqueous solutions of syringic acid (SA), vanillic acid (VA), p-toluic acid (TA), 4-hydroxy-benzoic acid (HBA) and 4-methyl salicylic acid (MSA). Each reaction was initiated by adding 10 μL of freshly prepared 1% ferricyanide reagent to 200 μL solution of each aromatic molecule and incubated at room temperature for 30 min. The amount of Prussian blue produced (ferricyanide activity) by each aromatic molecule was determined from the difference between the mean A750 value of each substrate and the reagent control reaction. Ferricyanide activity per unit molecule was determined by dividing the mean A750 value of each substrate by the amount of molecules (moles) in the reaction. The linear range of the assay was determined using different starting concentrations syringic acid (0.012, 0.024, 0.060, 0.120, 0.180 and 0.240 mM). The effect of potential reductants such as extrinsic protons (H+) in the reaction matrix on ferricyanide reaction was evaluated by conducting the assay on 0.05 mg mL−1 of syringic acid in 1, 10 and 25 mM of phosphate buffer (pH = 6.5) and acetate buffer (pH = 5.5). Each assay described above and the reagent control reactions were carried out in triplicates.
Analysis of lignin suspensions
Ferricyanide reactivity of lignin was evaluated using aqueous suspensions of Klason lignin from switchgrass, ionic-liquid derived lignin from Eucalyptus, standard alkali lignin (AL) and low sulfonate alkali lignin (ALS) from Sigma-Aldrich (St Louis, MO). The Klason and ionic-liquid derived lignin suspensions were prepared using protocol described in the ESI† and consist of particles ≤20 μm. The alkali lignin suspension (1 mg mL−1) were prepared by adding 0.1 g of each alkali lignin extract to 100 mL of DI-water and mixing vigorously. Prior to the assay, a 1.0 mL aliquots of each total lignin suspension (TLS) were centrifuged at 20
000 × g for 30 min. The supernatant (SUP) from each sample containing soluble lignin moieties was filtered through 0.45 μm membrane filter (VWR Scientific, Radnor, PA) to remove insoluble lignin aggregates. The pellet (PEL) from each sample was re-suspending in 1.0 mL of DI-water and centrifuged at 20
000 × g for 15–30 min washing. The supernatant was discard to remove any residual soluble lignin moieties. The PEL fraction form each lignin sample was three times (total) and re-suspended in 1.0 mL of DI-water. The aromatic and insoluble lignin content of the TLS, SUP and PEL fractions were estimated prior to ferricyanide assay by measuring the absorbance of each fraction at 280 and 600 nm wavelength respectively. The assay was initiated by adding 10 μL of freshly prepared ferricyanide reagent to 200 μL of TLS, SUP and PEL fractions from each lignin suspension and reagent control reaction in triplicates. Each reaction was mixed vigorously and incubated at room temperature for 30 min with occasional mixing. After incubation, the absorbance of 10-fold dilution of each reaction was measured at 750 nm wavelength. The ferricyanide activity of each fraction was determined by subtracting the mean Abs750 of the reagent control from the mean Abs750 of the substrate. The ferricyanide activity of each fraction was normalized by dividing the subtracted the mean Abs750 by the corresponding substrate absorbance at 280 nm (Abs750/280) or at 600 nm (Abs750/600). The alkali lignin suspensions were processed as described in the Results and discussion section.
Syringic acid consumption by Sphingobium sp. SYK-6
Ferricyanide assay was used to monitor syringic acid consumption by Sphingobium sp. SYK-6 in triplicates. The organism was grown at 30 °C (200 rpm) in 50 mL of minimal salt medium (MSM) that was supplemented with 0.5 g L−1 syringic acid in 250 mL screw-cap flasks. The composition of MSM broth is described in Table S1 in ESI section.† Approximately 0.6 mL aliquot of each culture was withdrawn at intervals to monitor growth and residual syringic acid. A 10-dilution of 0.1 mL of each aliquot was used to measure the growth of the organism at 600 nm (OD600). The remaining 0.5 mL aliquots were centrifuged at 10
000 × g for 10 min. Each supernatant was filtered through a 3 kDa Amicon-Ultra filter (Millipore, Billerica, MA). The 3 kDa filtrates were diluted 10-fold and subjected to ferricyanide reaction in triplicates in 96-well microplate as described earlier. The mean Abs750 of each aliquot was corrected for reagent absorbance as previously described.
Size exclusion chromatography analysis
Aqueous size exclusion chromatography analysis of soluble lignin moieties was carried out using Agilent 1200 series binary liquid chromatography (LC) system. HPLC grade DI-water (Honeywell, Morris Plains, NJ) DI-water as mobile phase at a flowrate of 1.0 mL min−1 at 25 °C. A 50 μL injection of each sample was resolved with Agilent PL-Aquagel-OH mixed-M (8 μm, 300 × 7.5 mm) column that was fitted with PL-Aquagel-OH guard column (8 μm, 50 × 7.5 mm). The eluates were detected with Agilent 1200 series diode array detector (DAD).
Acknowledgements
This work was part of the DOE Joint BioEnergy Institute (http://www.jbei.org) supported by the U. S. Department of Energy, Office of Science, Office of Biological and Environmental Research, through contract DE-AC02-05CH11231 between Lawrence Berkeley National Laboratory and the U. S. Department of Energy. The United States Government retains and the publisher, by accepting the article for publication, acknowledges that the United States Government retains a non-exclusive, paid-up, irrevocable, world-wide license to publish or reproduce the published form of this manuscript, or allow others to do so, for United States Government purposes. The authors extend their gratitude to Chen Lin and Deepti Tanjore at the Advanced Biofuels Process Demonstration Unit (ABPDU) at Lawrence Berkeley National Laboratory, Emeryville, CA for providing lignin extract from Eucalyptus.
Notes and references
- S. Sarkanen, D. C. Teller, E. Abramowski and J. L. McCarthy, Macromolecules, 1982, 15, 1098–1104 CrossRef CAS.
- A. M. González, J. L. Salager, M. R. Brunetto, M. Gallignani, J. L. Burguera, M. Burguera and Y. Petit de Peña, J. High Resolut. Chromatogr., 2000, 23, 693–696 CrossRef.
- D. L. Crawford, A. L. Pometto and R. L. Crawford, Appl. Environ. Microbiol., 1983, 45, 898–904 CAS.
-
J. Connors William, S. Sarkanen and L. McCarthy Joseph, in Holzforschung-International Journal of the Biology, Chemistry, Physics and Technology of Wood, 1980, vol. 34, p. 80 Search PubMed.
-
D. Cullen and P. J. Kersten, in Biochemistry and Molecular Biology, ed. R. Brambl and G. Marzluf, Springer, Berlin Heidelberg, 2004, vol. 3, ch. 13, pp. 249–273 Search PubMed.
- D. S. Wong, Appl. Biochem. Biotechnol., 2009, 157, 174–209 CrossRef CAS PubMed.
- D. J. Yelle, J. Ralph, F. Lu and K. E. Hammel, Environ. Microbiol., 2008, 10, 1844–1849 CrossRef CAS PubMed.
- W. Boerjan, J. Ralph and M. Baucher, Annu. Rev. Plant Biol., 2003, 54, 519–546 CrossRef CAS PubMed.
- T. D. H. Bugg, M. Ahmad, E. M. Hardiman and R. Rahmanpour, Nat. Prod. Rep., 2011, 28, 1883–1896 RSC.
- R. D. Hatfield, H.-J. G. Jung, J. Ralph, D. R. Buxton and P. J. Weimer, J. Sci. Food Agric., 1994, 65, 51–58 CrossRef CAS.
- H.-J. G. Jung, V. H. Varel, P. J. Weimer and J. Ralph, J. Agric. Food Chem., 1999, 47, 2005–2008 CrossRef CAS PubMed.
- O. Theander and E. A. Westerlund, J. Agric. Food Chem., 1986, 34, 330–336 CrossRef CAS.
- V. Hervé, X. Le Roux, S. Uroz, E. Gelhaye and P. Frey-Klett, Environ. Microbiol., 2014, 16, 2238–2252 CrossRef PubMed.
- D. J. Yelle, D. Wei, J. Ralph and K. E. Hammel, Environ. Microbiol., 2011, 13, 1091–1100 CrossRef CAS PubMed.
- R. S. Fukushima and M. S. Kerley, J. Agric. Food Chem., 2011, 59, 3505–3509 CrossRef CAS PubMed.
- R. D. Hatfield, J. Grabber, J. Ralph and K. Brei, J. Agric. Food Chem., 1999, 47, 628–632 CrossRef CAS PubMed.
- R. S. Fukushima and R. D. Hatfield, J. Agric. Food Chem., 2001, 49, 3133–3139 CrossRef CAS PubMed.
- R. Hatfield and R. S. Fukushima, Crop Sci., 2005, 45, 832–839 CrossRef CAS.
- R. S. Fukushima and R. D. Hatfield, J. Agric. Food Chem., 2004, 52, 3713–3720 CrossRef CAS PubMed.
- J. G. Buta, F. Zadrazil and G. C. Galletti, J. Agric. Food Chem., 1989, 37, 1382–1384 CrossRef CAS.
- J. R. Gasson, D. Forchheim, T. Sutter, U. Hornung, A. Kruse and T. Barth, Ind. Eng. Chem. Res., 2012, 51, 10595–10606 CrossRef CAS.
- L. Sun, P. Varanasi, F. Yang, D. Loqué, B. A. Simmons and S. Singh, Biotechnol. Bioeng., 2012, 109, 647–656 CrossRef CAS PubMed.
- T.-Q. Yuan, S.-N. Sun, F. Xu and R.-C. Sun, J. Agric. Food Chem., 2011, 59, 10604–10614 CrossRef CAS PubMed.
- M. Sette, R. Wechselberger and C. Crestini, Chem.–Eur. J., 2011, 17, 9529–9535 CrossRef CAS PubMed.
- J. Ralph, C. Lapierre, J. M. Marita, H. Kim, F. Lu, R. D. Hatfield, S. Ralph, C. Chapple, R. Franke, M. R. Hemm, J. Van Doorsselaere, R. R. Sederoff, D. M. O'Malley, J. T. Scott, J. J. MacKay, N. Yahiaoui, A.-M. Boudet, M. Pean, G. Pilate, L. Jouanin and W. Boerjan, Phytochemistry, 2001, 57, 993–1003 CrossRef CAS PubMed.
- J. Ralph, R. D. Hatfield, S. Quideau, R. F. Helm, J. H. Grabber and H.-J. G. Jung, J. Am. Chem. Soc., 1994, 116, 9448–9456 CrossRef CAS.
- S. Reale, A. Di Tullio, N. Spreti and F. De Angelis, Mass Spectrom. Rev., 2004, 23, 87–126 CrossRef CAS PubMed.
- R. Bayerbach, V. D. Nguyen, U. Schurr and D. Meier, J. Anal. Appl. Pyrolysis, 2006, 77, 95–101 CrossRef CAS.
- T. K. Kirk and R. L. Farrell, Annu. Rev. Microbiol., 1987, 41, 465–501 CrossRef CAS PubMed.
- R. Bourbonnais, M. G. Paice, I. D. Reid, P. Lanthier and M. Yaguchi, Appl. Environ. Microbiol., 1995, 61, 1876–1880 CAS.
- A. Hatakka, FEMS Microbiol. Rev., 1994, 13, 125–135 CrossRef CAS.
- T. K. Kirk, W. J. Connors and J. G. Zeikus, Appl. Environ. Microbiol., 1976, 32, 192–194 CAS.
- T. D. H. Bugg, M. Ahmad, E. M. Hardiman and R. Singh, Curr. Opin. Biotechnol., 2011, 22, 394–400 CrossRef CAS PubMed.
- M. Ahmad, C. R. Taylor, D. Pink, K. Burton, D. Eastwood, G. D. Bending and T. D. H. Bugg, Mol. BioSyst., 2010, 6, 815–821 RSC.
-
S. Baumberger, A. Abaecherli, M. Fasching, G. Gellerstedt, R. Gosselink, B. Hortling, J. Li, B. Saake and E. de Jong, in Holzforschung, 2007, vol. 61, p. 459 Search PubMed.
- J. Asikkala, T. Tamminen and D. S. Argyropoulos, J. Agric. Food Chem., 2012, 60, 8968–8973 CrossRef CAS PubMed.
- R. D. Lillie and H. J. Burtner, J. Histochem. Cytochem., 1953, 1, 87–92 CrossRef CAS PubMed.
- R. D. Lillie and P. T. Donaldson, Histochem. J., 1974, 6, 679–684 CrossRef CAS PubMed.
- K. I. Berker, K. Güçlü, İ. Tor and R. Apak, Talanta, 2007, 72, 1157–1165 CrossRef CAS PubMed.
- G. K. Jayaprakasha, R. P. Singh and K. K. Sakariah, Food Chem., 2001, 73, 285–290 CrossRef CAS.
- H. Zhang, J. Li, K. Wang, X. Du and Q. Li, Anal. Biochem., 2009, 388, 40–46 CrossRef CAS PubMed.
-
E. Gail, S. Gos, R. Kulzer, J. Lorösch, A. Rubo, M. Sauer, R. Kellens, J. Reddy, N. Steier and W. Hasenpusch, in Ullmann's Encyclopedia of Industrial Chemistry, Wiley-VCH Verlag GmbH & Co. KGaA, 2000, DOI:10.1002/14356007.a08_159.pub3.
- B. Cerdá, R. Llorach, J. J. Cerón, J. C. Espín and F. A. Tomás-Barberán, Eur. J. Nutr., 2003, 42, 18–28 CrossRef PubMed.
- S. A. Heleno, L. Barros, A. Martins, M. J. R. P. Queiroz, P. Morales, V. Fernández-Ruiz and I. C. F. R. Ferreira, LWT--Food Sci. Technol., 2015, 63, 475–481 CrossRef CAS.
- S. B. Pointing, Fungal Divers., 1999, 2, 17–33 Search PubMed.
- R. W. Whetten, J. J. MacKay and R. R. Sederoff, Annu. Rev. Plant Physiol. Plant Mol. Biol., 1998, 49, 585–609 CrossRef CAS PubMed.
- A. Simić, D. Manojlović, D. Šegan and M. Todorović, Molecules, 2007, 12, 2327–2340 CrossRef.
- V. L. Pardini, S. K. Sakata, R. R. Vargas and H. Viertler, J. Braz. Chem. Soc., 2001, 12, 223–229 CrossRef CAS.
- T. Sawa, M. Nakao, T. Akaike, K. Ono and H. Maeda, J. Agric. Food Chem., 1998, 47, 397–402 CrossRef.
- X. Li, E. Ximenes, Y. Kim, M. Slininger, R. Meilan, M. Ladisch and C. Chapple, Biotechnol. Biofuels, 2010, 3, 27 CrossRef CAS PubMed.
-
G. Brunow, in Biopolymers Online, Wiley-VCH Verlag GmbH & Co. KGaA, 2005, DOI:10.1002/3527600035.bpol1003.
- J. Rencoret, A. Gutiérrez, L. Nieto, J. Jiménez-Barbero, C. B. Faulds, H. Kim, J. Ralph, A. T. Martínez and J. C. del Río, Plant Physiol., 2010, 155, 667–682 CrossRef PubMed.
- C. Li, D. Tanjore, W. He, J. Wong, J. L. Gardner, K. L. Sale, B. A. Simmons and S. Singh, Biotechnol. Biofuels, 2013, 6, 1–14 CrossRef.
- Z. Hu, R. Sykes, M. F. Davis, E. Charles Brummer and A. J. Ragauskas, Bioresour. Technol., 2010, 101, 3253–3257 CrossRef CAS PubMed.
- R. Vanholme, B. Demedts, K. Morreel, J. Ralph and W. Boerjan, Plant Physiol., 2010, 153, 895–905 CrossRef CAS PubMed.
- S. A. Eichorst, C. Joshua, N. Sathitsuksanoh, S. Singh, B. A. Simmons and S. W. Singer, Appl. Environ. Microbiol., 2014, 80, 7423–7432 CrossRef CAS PubMed.
- J. B. Reeves, J. Dairy Sci., 1993, 76, 120–128 CrossRef CAS.
- K. R. Aadil, A. Barapatre, S. Sahu, H. Jha and B. N. Tiwary, Int. J. Biol. Macromol., 2014, 67, 220–227 CrossRef CAS PubMed.
- T. Kalaivani and L. Mathew, Food Chem. Toxicol., 2010, 48, 298–305 CrossRef CAS PubMed.
- R. Hatfield and R. S. Fukushima, Crop Sci., 2005, 45, 832–839 CrossRef CAS.
- J. S. Lupoi, S. Singh, R. Parthasarathi, B. A. Simmons and R. J. Henry, Renewable Sustainable Energy Rev., 2015, 49, 871–906 CrossRef CAS.
- S. Sasaki, T. Nishida, Y. Tsutsumi and R. Kondo, FEBS Lett., 2004, 562, 197–201 CrossRef CAS PubMed.
- B. Cathala and B. Monties, Int. J. Biol. Macromol., 2001, 29, 45–51 CrossRef CAS PubMed.
- M. J. Selig, S. Viamajala, S. R. Decker, M. P. Tucker, M. E. Himmel and T. B. Vinzant, Biotechnol. Prog., 2007, 23, 1333–1339 CrossRef CAS PubMed.
- S. V. Pingali, V. S. Urban, W. T. Heller, J. McGaughey, H. O'Neill, M. Foston, D. A. Myles, A. Ragauskas and B. R. Evans, Biomacromolecules, 2010, 11, 2329–2335 CrossRef CAS PubMed.
- L. Petridis, S. V. Pingali, V. Urban, W. T. Heller, H. M. O'Neill, M. Foston, A. Ragauskas and J. C. Smith, Phys. Rev. E: Stat., Nonlinear, Soft Matter Phys., 2011, 83, 061911 CrossRef PubMed.
- H. Jørgensen, J. B. Kristensen and C. Felby, Biofuels, Bioprod. Biorefin., 2007, 1, 119–134 CrossRef.
- M. E. Himmel, K. K. Oh, D. W. Sopher and H. L. Chum, J. Chromatogr. A, 1983, 267, 249–265 CrossRef CAS.
- O. Milstein, A. Hüttermann, R. Fründ and H.-D. Lüdemann, Appl. Microbiol. Biotechnol., 1994, 40, 760–767 CrossRef CAS.
-
R. Sri, L. S. Yasar and L. W. Douglas, in Lignin, American Chemical Society, 1989, vol. 397, ch. 11, pp. 144–154 Search PubMed.
- L. Bandounas, N. Wierckx, J. de Winde and H. Ruijssenaars, BMC Biotechnol., 2011, 11, 94 CrossRef CAS PubMed.
- J. R. Borgmeyer and D. L. Crawford, Appl. Environ. Microbiol., 1985, 49, 273–278 CAS.
- A. J. Ragauskas, G. T. Beckham, M. J. Biddy, R. Chandra, F. Chen, M. F. Davis, B. H. Davison, R. A. Dixon, P. Gilna, M. Keller, P. Langan, A. K. Naskar, J. N. Saddler, T. J. Tschaplinski, G. A. Tuskan and C. E. Wyman, Science, 2014, 344, 1246843 CrossRef PubMed.
- C. Crestini, F. Melone, M. Sette and R. Saladino, Biomacromolecules, 2011, 12, 3928–3935 CrossRef CAS PubMed.
Footnote |
† Electronic supplementary information (ESI) available. See DOI: 10.1039/c6ra04443c |
|
This journal is © The Royal Society of Chemistry 2016 |