DOI:
10.1039/C6RA02308H
(Paper)
RSC Adv., 2016,
6, 31092-31100
Investigation into the adsorption of partially hydrolyzed polyacrylamide onto in situ formed magnesium hydroxide particles†
Received
26th January 2016
, Accepted 14th March 2016
First published on 16th March 2016
Abstract
In situ formed magnesium hydroxide (Mg(OH)2) particles were investigated through the direct wet precipitation method in the presence of two partially hydrolyzed polyacrylamides (HPAM) of different molecular weights. The influence of the reaction parameters, such as MgCl2 concentration, pH, and reaction temperature, on the interactions between in situ formed Mg(OH)2 particles and HPAM molecules was investigated. The Mg(OH)2 particles obtained in the absence and presence of polymers were characterized in terms of their morphology, particle size, and crystal habit by high-resolution transmission electron microscopy, X-ray diffraction, Fourier transform infrared spectroscopy, dynamic light scattering, and zeta potential measurements. It was found that different pH values of the reaction solution led to different predominant species distribution for MgCl2. The adsorbed polymer led to the formation of four distinct adsorption regions as the concentration of polymer increased. The adsorption of partially hydrolyzed polyacrylamide on the oppositely charged Mg(OH)2 particles was explained by electrostatic attraction, hydrogen bonding, and bridging.
1. Introduction
Water-soluble polymers are a diverse group of chemicals that are often added to colloidal-based products to improve their colloidal dispersity, viscosity, tactility, and rheological properties.1 They generally consist of polar groups, which are well-solvated in water, and a non-polar repeating unit structure, which is not easily dissolved in water. Hence, water-soluble polymers combine polar and non-polar properties in one molecule.2,3 Water-soluble polymers are extensively used in industrial fields, such as mineral separation, drug formulation, food processing, agriculture, oil recovery, water treatment, and soil remediation.4–7 In petroleum industry, water-soluble polymers, for example, partially hydrolyzed polyacrylamide (HPAM), is added to displacing fluids to enhance oil recovery (EOR) in the chemical flooding, which includes polymer flooding, surfactant flooding, alkali/polymer flooding, and alkali/surfactant/polymer (ASP) flooding.8–10 Polymer flooding and ASP flooding are considered to be the most attractive chemical flooding processes. The role of HPAM in most EOR applications is to increase the viscosity and control the mobility of the aqueous phase. Because of the negatively charged carboxyl groups along the HPAM chains, HPAM chains are strongly repelled by electrostatic repulsion, but the charge also gives a more expanded chain conformation, which results in higher aqueous phase viscosity.11,12 The higher aqueous phase viscosity can enhance sweep efficiency during EOR processes.12 After the EOR process, residual HPAM and its degradation products are dispersed in produced water. It could associate with suspended solid and oil, which are steadily dispersed in produced water and difficult to remove.9,10 Residual HPAM which is present in the produced water that leads to the formation of stable and persistent oil-in-water (O/W) emulsions is a challenge for petroleum industry.9,13,14 Because HPAM has a certain surface activity, it can rapidly adsorb at the surface of oil droplets and form highly viscoelastic film which protects the oil droplets against coalescence.14,15 In this case, the produced water becomes very stable. At the same time, the viscosity of the aqueous phase may significantly increase. Furthermore, the viscosity of aqueous phase increases with an increase of the molecular weight of HPAM. With the concentration of residual HPAM increasing in the produced water, the separation of oil from produced water is more and more difficult.8,16 In the current treatment process, even though oil could be removed from produced water, most of residual HPAM still remained in the aqueous phase. The mobility and transfer efficiency of the aqueous phase might significantly decrease. Therefore, effective removal of HPAM from aqueous phase became urgent and important.
Magnesium hydroxide (Mg(OH)2) has been attracted widely interest, for its application in flame-retardant fields, smoke suppressant in various polymeric materials, and environment protection.17–19 Mg(OH)2 has a layered crystal structure with the hydroxyl groups oriented bonding to the hexagonal basal plane.17,20 Such layered metal hydroxides have several attractive features. For example, the large specific surface area is potentially applicable to promote adsorption. Another important aspect is the higher charge density of the sheets, which contributed to maintain higher reactivity.21 Several methods have been used to prepare Mg(OH)2 with controlled shape and size.17,19 Some literature have reported the synthesis of nano-sized Mg(OH)2 by sol–gel,18,22,23 hydrothermal19,24 and solvothermal techniques.25,26 However, these methods require more complicated process or longer synthesis time, which make them not practical for industrial production.27
In this study, the in situ wet precipitation method was used because it is simple and suitable for industrial processing. In this method, MgCl2 and HPAM solutions were initially present in the reactor at a controlled temperature, and Mg(OH)2 particles were obtained by the addition of alkali. In situ formed Mg(OH)2 particles were used as effective adsorbent to remove HPAM from aqueous solution. The molecular weight and charge density are two specific characteristics of water-soluble polymers. Thus, we used two different molecular weights of HPAM with different charge densities as model water-soluble polymers to investigate the interactions between HPAM molecules and oppositely charged Mg(OH)2 particles. The effects of the reaction parameters, such as MgCl2 concentration, pH, and temperature, on the adsorption efficiency were investigated. The related process mechanism was also discussed.
2. Experimental section
2.1. Materials
Analytical reagent grade magnesium chloride hexahydrate (MgCl2·6H2O) and NaOH were purchased from Sinopharm Chemical Reagent Co., Ltd. (Shanghai, China). The solvent used in the experiment was distilled water. One type of partially hydrolyzed polyacrylamide with weight-average molecular weight (Mw) of 5.0 × 106 and hydrolysis degree of 25–28% was supplied by Sumitomo Chemical Company, and designated as HPAM-1. Another type of partially hydrolyzed polyacrylamide with Mw = 1.2 × 107 and hydrolysis degree of 28% was provided by Shengli Oilfield in China, and designated as HPAM-2. All of the other reagents were of analytical grade and used without further purification.
2.2. Preparation of Mg(OH)2 particles and Mg(OH)2–HPAM complexes
Mg(OH)2 particles were prepared with the following procedure: 20 mL of 0.6 mol L−1 NaOH solution was dropped into 20 mL of 0.3 mol L−1 MgCl2 solution at room temperature to obtain Mg(OH)2 particles.
Different amounts of HPAM were pre-dissolved in 1000 mL deionized water to obtain a series of solutions. After the HPAM powders were completely dissolved, 20 mL of HPAM solution was added to 20 mL of MgCl2 solution with mechanical agitation for 1 h, and then 20 mL of NaOH solution was added to the above mixtures. The mixtures were continuously agitated on a shaker for 4 h at a controlled temperature to obtain Mg(OH)2–HPAM complexes.
2.3. Characterization
The morphology and size of the synthesized Mg(OH)2 particles with and without HPAM were characterized using a JEM-2100 high-resolution transmission electron microscope (HRTEM, JEOL Ltd., Japan). The crystal structure of samples was identified by X-ray diffraction (XRD) diffractometer (X'Pert PRO, PANalytical Ltd., Netherlands) with CuKα radiation (40 kV, 40 mA) in continuous scanning mode. The 2θ scanning ranged from 5° to 80° with a scanning rate of 2° min−1. The chemical bonds and surface functional groups of the samples were recorded by a Fourier transform infrared spectrometer (Tensor 27, Bruker, USA) by the KBr pellet technique, and scanned from 500 to 4000 cm−1 with a 4 cm−1 spectral resolution.
The zeta potential of Mg(OH)2 particles, HPAM-1, and HPAM-2 in the NaCl solution (10−3 M) as a function of pH was determined by a ZetaPALS analyzer (Brookhaven, New York, USA). For each sample, an appropriate amount of undiluted solution was placed in a cuvette, and the average zeta potential value was determined from three separate measurements.
Size analyses experiments were carried out using a dynamic laser light scattering system (DLS, Brookhaven, New York, USA) consisting of a BI-9000AT digital correlator and a BI-200SM goniometer with a 35 mW HeNe laser source. The temperature of all of the experiments was set to 25 ± 0.1 °C using a Polyscience temperature controller. All data were collected at a scattering angle of 90°.
2.4. Adsorption experiments
Adsorption experiments were performed using a batch method. For the adsorption experiments, 20 mL of HPAM solution was added to 150 mL glass Erlenmeyer flasks containing 20 mL of different concentrations of magnesium chloride solution. The concentration of the magnesium chloride solutions were between 0.1 and 1.5 mol L−1, and the concentration of the alkaline solutions (NaOH) were between 0.2 and 3.0 mol L−1. The alkaline solution was added to the Erlenmeyer flasks containing mixtures of magnesium chloride and HPAM. The mixtures were vigorously stirred during the addition of alkali, and then continuously shaken at 150 rpm for 4 h using a thermostatic shaker. To determine the concentration of HPAM adsorbed on in situ formed Mg(OH)2, at given time intervals, the solid phase was separated from the aqueous phase by centrifuging at 10
000 g for 15 min. The HPAM concentration in the supernatant was analyzed for total organic carbon (TOC) with a TOC analyzer (TOC-VCPN FA, CN200, Shimadzu Co., Kyoto, Japan), and the adsorbed HPAM concentration (mg-HPAM/g-Mg(OH)2) was calculated by mass balance.
The effect of pH on adsorption was investigated at 30 °C with an initial HPAM concentration of 100 mg L−1, MgCl2 dosage of 0.3 mol L−1, and an adsorption time of 4 h. The effect of temperature on adsorption was assessed by varying the temperature from 20 to 40 °C. Adsorption isotherm studies were then conducted using a fixed amount (0.3 mol L−1) of MgCl2 with HPAM solutions at different concentrations (20–500 mg L−1) for 4 h at 40 °C.
3. Results and discussion
3.1. Characterization
High-resolution transmission electron microscopy. The morphology and nanostructure of Mg(OH)2 with and without HPAM were examined by HRTEM. Typical HRTEM images of the samples are shown in Fig. 1(a)–(c). The Mg(OH)2 crystals were composed of small, laminated thin platelets with a average size in the range of 50–100 nm. As shown in Fig. 1(b) and (c), small Mg(OH)2 particles with HPAM can easily form aggregates. The aggregates were composed of numerous closely packed nanosheets with high density. The aggregates became larger with an increase of the molecular weight of HPAM, with diameters ranging from several to tens of microns. This means that HPAM may interact with Mg(OH)2 particles in aqueous solution. The classical DLVO theory readily explains the aggregation processes of colloidal particles resulting from van der Waals forces and electrostatic interactions.28,29 This is important because the adsorbed HPAM lead to charge neutralization or charge reversal. These interactions are likely to drive the aggregation of charged particles in the presence of an oppositely charged polymer. As the chain length of the polymer increases and the surfaces of the particles are fully covered with polymer, polymer can interact with two or more particles and cause aggregation by bridging.30
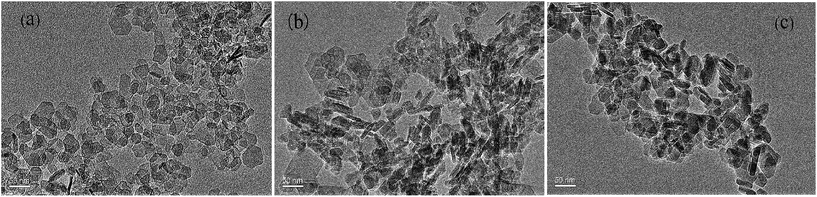 |
| Fig. 1 HRTEM images of (a) Mg(OH)2, (b) in situ formed Mg(OH)2 with HPAM-1, and (c) in situ formed Mg(OH)2 with HPAM-2 (Mw of HPAM-1 = 5.0 × 106 and Mw of HPAM-2 = 1.2 × 107). | |
X-ray diffraction. The in situ formed Mg(OH)2 with and without HPAM were rapidly dried to form powders, and then the powders were analyzed by XRD. In Fig. 2(a), all of the diffraction peaks can be indexed as these of hexagonal Mg(OH)2 crystal diffraction (JCPDS card no. 82-2453). Mg(OH)2 had layered structure with a basal spacing of 4.78 Å. Upon adsorption of HPAM on the Mg(OH)2 particles, the basal spacing of in situ formed Mg(OH)2 with HPAM-1 and HPAM-2 slightly increased from 4.78 to 4.79 and 4.81 Å, respectively, and the intensity of the diffraction peaks slightly decreased. Charge neutralization is an important aspect. Negatively charged of the HPAM segments adsorb on oppositely charged particle surfaces, forming a adsorbed layer, and the thickness of the adsorbed layer may increase with an increase of the molecular weight of HPAM. The addition of negatively charged polymer to a oppositely charged dispersion causes strong electrostatic attraction between the particles and the adsorbed polymer segments31 in order to form a close-packed Mg(OH)2–HPAM complexes. Hence, the basal spacing of the Mg(OH)2–HPAM complexes only slightly increased compared with that of Mg(OH)2.
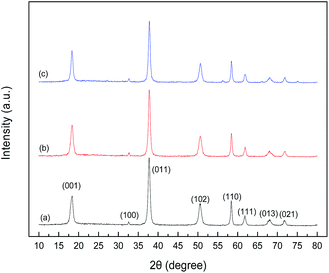 |
| Fig. 2 XRD patterns of Mg(OH)2 with and without HPAM. (a) Mg(OH)2, (b) Mg(OH)2 with HPAM-1, and (c) Mg(OH)2 with HPAM-2 (Mw of HPAM-1 = 5.0 × 106 and Mw of HPAM-2 = 1.2 × 107). | |
Fourier transform infrared spectroscopy. Mg(OH)2, HPAM, and the Mg(OH)2–HPAM complexes were characterized by FT-IR spectroscopy. In the spectra of the Mg(OH)2 particles (Fig. 3), a strong adsorption band at 3699 cm−1 is assigned to free OH stretching vibration in Mg(OH)2.32 The adsorption band appeared at 1642 cm−1 can be attributed to the bending vibration of H–O–H.32,33 The broad adsorption band between 3300 and 3500 cm−1 is related to O–H stretching vibration. The band in the low wavenumber region (<500 cm−1) of the spectrum can be as attributed to lattice vibrations of Mg(OH)2, such as the O–Mg–O vibration.33 The absorption band positions of HPAM-1 and HPAM-2 are shown in Fig. 3. We focused our discussion on the functional groups (e.g., carboxylic, amide group, amino group, and methylene group) as well as their bonds, because these functional groups provide insight into the mechanisms of bonding between the polymer and Mg(OH)2.17,34 For HPAM-1 and HPAM-2, the FT-IR bands at 3449 and 3440 cm−1 can be assigned to asymmetric stretching vibration of the amino group of the primary amide, and the bands at 1637 and 1669 cm−1 can be attributed to stretching vibration of the C
O bond in carboxylic group.34 The symmetric stretching vibration of the C–O bond is split (1456 and 1405 cm−1 for HPAM-1, and 1461 and 1401 cm−1 for HPAM-2).35,36 The split bands at 1135 and 1113 cm−1 for HPAM-1, and 1178 and 1118 cm−1 for HPAM-2 can be attributed to stretching vibration of the C–N bond of the secondary amide.37 The bands at 412 and 590 cm−1 for HPAM-1 and HPAM-2 are attributed to bending vibration of the C–C
O bond of the amide group.34 Moreover, the bands at 2851–2921 cm−1 and 2847–2925 cm−1 for HPAM-1 and HPAM-2 can be assigned to stretching vibration of the C–H bond of the methylene group.
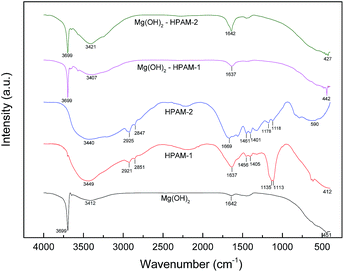 |
| Fig. 3 FTIR spectra of Mg(OH)2, HPAM, and Mg(OH)2–HPAM complexes. | |
In the spectra of the Mg(OH)2–HPAM complexes, the bands of HPAM-1 and HPAM-2 have distinct shifts: a red-shift in the asymmetric stretching band of the amino group from 3449 and 3440 cm−1 to 3407 and 3421 cm−1 for HPAM-1 and HPAM-2, respectively, and a 27 cm−1 red-shift in the stretching vibration of the C
O bond from 1669 to 1642 cm−1 for HPAM-2. There is a 30 cm−1 blue-shift in the bending vibration of the C–C
O bond for HPAM-1, and a 163 cm−1 red-shift in the bending vibration of the C–C
O bond for HPAM-2. The stretching vibrations of C–H bond of the methylene group and C–N bond are not visible in the spectra as Mg(OH)2 is miscible with HPAM. This is because MgCl2 is dissociated into Mg2+ and Cl− ions while Mg(OH)2 particles were in situ formed with the addition of alkaline solution, and hydrogen bonds formed between the nitrogen atom of the amino group and the hydroxyl group of the Mg(OH)2 particles. At the same time, when water-soluble HPAM remains in aqueous solution, the carboxylic group of the HPAM can ionize to give negatively charged carboxylate anion. There is strong association between polymer segments and oppositely charged Mg(OH)2 particles. In this case, the HPAM segments might directly pack into host Mg(OH)2 crystals. This may lead to the shift and disappearance of some bands.
Zeta potential measurements. Zeta potential is widely used for the characterization of charged particles. It is well known that the zeta potential of Mg(OH)2 is pH dependent.38 The zeta potentials of the Mg(OH)2 suspensions and HPAM solutions as a function of pH are shown in Fig. 4. It showed that Mg(OH)2 particles had a positive zeta potential in the 10−3 M NaCl solution owing to the high value of the point of zero charge (PZC). With increasing solution pH, the hydroxyl ions were adsorbed on the Mg(OH)2 particle surfaces, the zeta potential decreased and was reduced or reversed as the pH of solution further increased. The surface charge of the Mg(OH)2 particles depends on the composition of their surface layer: [Mz+(OH)n]z−n, where z represents the valence of the cation and n represents the number of hydroxide ions.38,39 At relatively low pH values, two surface species, Mg2+ and MgOH+, are responsible for the high surface charge density of particles. Thus the surface is positively charged. At high pH values, [Mg2+(OH)n]2−n (n > 2) responsible for the surface charge density of particles. Therefore, the surface is negatively charged.
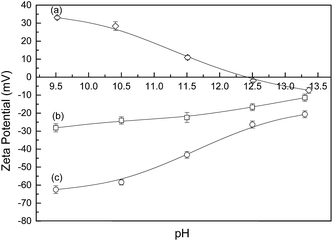 |
| Fig. 4 Zeta potential of (a) Mg(OH)2, (b) HPAM-1, and (c) HPAM-2 as a function of pH at 25 °C in 10−3 M NaCl (Mw of HPAM-1 = 5.0 × 106 and Mw of HPAM-2 = 1.2 × 107). | |
At pHpzc, Mg(OH)2 is responsible for the surface charge density of particles.38–41 In this work, the PZC of the Mg(OH)2 particles was about pH 12.3 (Fig. 4).
In the zeta potential measurements, no potential reversal was observed for HPAM-1 and HPAM-2, that is, the HPAM surface was negatively charged within the pH range of this study. The number of OH− ions combining with amide groups of HPAM increases with increasing solution pH, and thus negative charge density of the polymer increases, and the present counterion concentration is not enough to effectively screen charges on the polymer chains. Moreover, the zeta potential of HPAM-1 and HPAM-2 increased with an increase in solution pH, meaning that the absolute value of the zeta potential decreased. In general, increasing solution pH increases ionic strength of the solution. As a result, the electrical double layers on the polymer chains are compressed, and the negative charges on the carboxylic groups are shielded. Therefore, the absolute value of the zeta potential will decrease. Furthermore, the hydrolysis degree of HPAM increases with increasing solution pH. Because HPAM-2 molecules have more amide groups on the polymer chains than that of HPAM-1, leading to higher hydrolysis degree and negative charge density. At high pH value, the charges on the polymer chains of HPAM-2 might be shielded faster than that of HPAM-1. Hence, the variation in zeta potential was significantly larger than that of HPAM-1.
Size distribution analysis. As shown in Fig. S1 (ESI†), the particle size is larger than the results of the HRTEM analysis because of adhesion of particles and hydration. There is only one peak for all of the samples, and the distribution of the hydrodynamic diameter is narrow. The average hydrodynamic diameters of Mg(OH)2, HPAM-1, and HPAM-2 were 116, 146, and 180 nm, respectively. In the peak with small diameter (<1 μm), Mg(OH)2 and HPAM exhibited a good polydispersity index.After the HPAM adsorbed on the surface of oppositely charged Mg(OH)2 particles by electrostatic attraction, Mg(OH)2 and HPAM tended to form Mg(OH)2–HPAM complexes or aggregates, as shown in Fig. S2 (ESI†). As time passes, large aggregates appear and grow by the accretion of other primary aggregates until a network composed of partially aggregated particles forms, leading to flocculation. In this case, the aggregates separate from the aqueous phase.
3.2. Polymer adsorption studies
Effect of MgCl2 concentration. The adsorption of HPAM on the in situ formed Mg(OH)2 particles was strongly affected by the initial MgCl2 concentration. Adsorption experiments were conducted to further investigate the relationship between the adsorption behavior of HPAM and the formation process of Mg(OH)2. The initial HPAM concentration was 100 mg L−1 and the MgCl2 concentration ranged from 0.1 to 1.5 mol L−1 at pH 9.5. The adsorption efficiency of HPAM rapidly increased with an increase of MgCl2 concentration, and then gradually reached a plateau (Fig. 5).
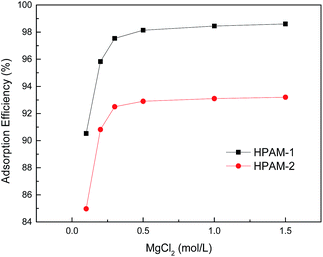 |
| Fig. 5 Effect of MgCl2 concentration on adsorption efficiency. Experimental conditions: initial HPAM concentration = 100 mg L−1, pH = 9.5, contact time = 4 h at 30 °C. | |
At alkaline conditions, magnesium ions precipitate as Mg(OH)2. Mg(OH)2 belongs to the family of divalent metal hydroxides, which are isostructural with a layered compound.42 In the process of crystal growth, OH− ions and water molecules coexist in the system, and the water molecules compete with OH− ions to form Mg–O–H bonds.43 The association of magnesium and hydroxide ions form MgOH+ species.44 When the concentration of MgOH+ species becomes sufficiently high, they aggregate into small nuclei through homogeneous nucleation. After the extremely fast nucleation, with a continuous supply of the building nuclei, these nuclei act as seeds for further growth to form the layered structure. With an increase in MgCl2 concentration, more Mg2+ is involved in the nucleation process. These factors led to the growth of nano-sized Mg(OH)2 crystals via self-assembly of lamellar nanocrystals. HPAM-1 and HPAM-2 were adsorbed on the surfaces of Mg(OH)2 through electrostatic attraction and hydrogen bonding. As other particles were close to the polymer molecules, they also interacted with the polymer molecules. Because the long polymer chains may be extended from the particle surfaces, it is possible for charged parts of the polymer to become attracted to other particles, bridging or linking particles together.31 In this case, HPAM molecules acted as “bridges” to join neighboring Mg(OH)2 crystals. It is possible that HPAM molecules and Mg(OH)2 particles can form three-dimensional network structure by oriented cohesion and adhesion as well as intermolecular hydrogen bonds.45 Therefore, the existence of HPAM in the solution may enhance the nucleation and growth of Mg(OH)2, and the adsorbed amount of HPAM rapidly increased during these processes. Moreover, the HPAM molecules adsorbed on the MgOH+ species may decrease the surface energy for forming Mg(OH)2 lamellae. As a result, the nano-sized Mg(OH)2 lamellae with HPAM molecules self-assembled into a larger microstructure in the form of flocculent aggregates. Co-precipitation/adsorption processes occur during the growth of complex Mg(OH)2 crystals. The MgCl2 concentration plays an important role in the growth rate of nuclei and the amount of Mg(OH)2 formed.
When polymer molecules adsorb at a solid–liquid interface, the polymer chains with weak affinity for the particle surfaces can construct flexible adsorbed polymer layers. It is likely that the adsorbed polymer layers effectively occupy the available surface area and block adsorption sites on the particle surfaces, leading to steric hindrance between the adsorbed polymer layers and particle surfaces, and hence the adsorbed amount of polymer remains almost constant with further increasing MgCl2 concentration. On the other hand, the experimental data also suggested that the adsorption efficiency of HPAM-1 was higher than that of HPAM-2. This is because HPAM-2 has more amide groups on the molecular chains than that of HPAM-1, which leads to a larger molecular size. Compared to HPAM-2, HPAM-1 has higher diffusion rate from the bulk phase to the solid–liquid interface. Through electrostatic attraction and hydrogen bonding, the adsorption of HPAM-1 molecules on particle surfaces might occur. The particle surfaces will become highly covered by forming closely-packed HPAM-1 layers. It follows that the adsorbed amount should be high, therefore, the adsorption efficiency of HPAM-1 was higher than HPAM-2.
Effect of pH. The pH value of the reaction solution is an important parameter that controls dissolution/precipitation of Mg(OH)2 and the surface charge density. In this study, the pH ranged from 8.5 to 13.5. The concentration of HPAM was 100 mg L−1. The effect of pH on the adsorption efficiency of HPAM on Mg(OH)2 is shown in Fig. 6. The adsorption efficiency of HPAM on Mg(OH)2 increased with an increase of pH until pH 9.5, and then the adsorption efficiency of HPAM rapidly decreased with further increase of pH.
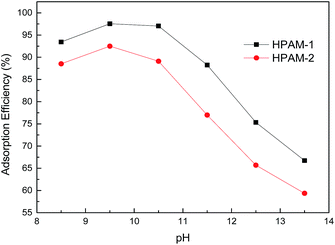 |
| Fig. 6 Effect of pH on the adsorption efficiency of HPAM on Mg(OH)2 particles. Experimental conditions: initial HPAM concentration = 100 mg L−1, Mg(OH)2 dosage = 0.3 M, contact time = 4 h at 30 °C. | |
It should be noted that different pH values of the reaction solution lead to different species distributions for MgCl2 (Visual MINTEQ ver. 3.0).46 The dominant species at the solid–liquid interface are Mg2+, MgOH+, and Mg(OH)2, as illustrated by the speciation diagram shown in Fig. 7. With an increase of pH, the concentration of Mg2+ remained almost constant for pH < 9 but rapidly decreased for pH > 9. When the pH is in the range of 9.0–11.0, Mg2+, MgOH+, and Mg(OH)2 coexist in the system. It has been suggested that this behavior is attributed to the protonation of Mg(OH)2 surface sites, leading to the formation of MgOH+ species,45,47,48 which achieve a maximum concentration at pH 9.15. This pH value may correspond to the predominance of the magnesium ion and magnesium hydroxide species: Mg2+, MgOH+, and Mg(OH)2. For pH > 11.0, the predominant species is Mg(OH)2.
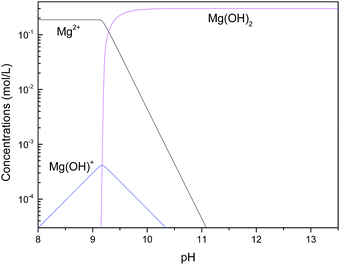 |
| Fig. 7 Species distribution diagram for 0.3 M MgCl2 in the pH range from 8 to 13.5. | |
Alkaline solution was added to the mixture of MgCl2 and HPAM, and the original pH was 12.5. Dilute HCl and NaOH solutions were used to adjust the pH from 8.5 to 13.5. At pH 9.5, the predominant species were Mg2+, MgOH+, and Mg(OH)2. A considerable increase in positive charge takes place for Mg2+ and MgOH+ species. The zeta potential of the MgOH+ species is more positive than that of Mg(OH)2. Therefore, electrostatic attraction increased between MgOH+/Mg(OH)2 and the polymer. At pH values below the PZC, the adsorption mechanism should be primarily electrostatic attraction. In contrast, at pH values above the PZC, electrostatic repulsion between Mg(OH)2 and HPAM decreased the adsorption efficiency. Moreover, the number of OH− ions combining with amide groups of HPAM increases with increasing aqueous phase pH, which leads to higher hydrolysis degree of HPAM. In this case, the solubility and negative charge density of the HPAM increases. HPAM tends to remain in aqueous phase, and hence the adsorption amount of HPAM at the solid–liquid interface decreases. The maximum adsorption efficiency of HPAM on Mg(OH)2 was obtained at pH 9.5, we chose pH 9.5 for subsequent adsorption experiments.
Effect of temperature. The adsorption efficiencies at three different temperatures (20, 30, and 40 °C) are shown in Fig. 8. The adsorption efficiency of HPAM increased with an increase of temperature, indicating that adsorption was endothermic. Furthermore, reaction temperature has stronger influence on the adsorption efficiency of HPAM-2 than that of HPAM-1. This can be explained by nucleation and growth of Mg(OH)2 particles as well as hydrophilicity/hydrophobicity of polymer at the solid–liquid interface. For a reaction system, it is clear that the reaction temperature significantly affects the particle size, aggregation level, and morphology of Mg(OH)2.49,50 The ionization rate of the magnesium salt and alkali increases with increasing aqueous phase reaction temperature, so the formation rate of Mg2+ and OH− ions increases.49,50 The reaction seemed to begin with the addition of NaOH to the mixture of MgCl2 and HPAM, followed by the formation of hexagonally shaped nuclei. The subsequent growth was along the edges of these nuclei. A temperature of 20 °C seems to be a critical point for forming lamellar crystals. When the reaction temperature was above this critical point (e.g., 30 °C), the Mg(OH)2 crystals were completely lamellar shaped.51,52 This means that the diameter of the particles increased with an increase of temperature. But the thickness of the lamellar crystal was not sensitive to the change of temperature and remained almost constant at 30–40 nm for 20–50 °C.52,53 During the crystallization process, MgOH+ is formed to the vicinity of the edges of each nuclei with high surface energy. Once the MgOH+ concentration reaches a critical value, the MgOH+ species will nucleate and grow into small nanoplates. It is possible that both reactants and MgOH+ species further self-assembled via adhesion and cohesion until the Mg(OH)2 crystalline structure is completely consumed.
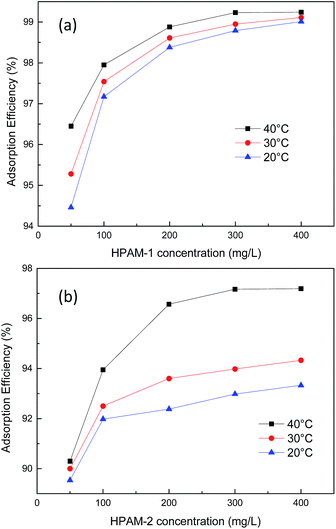 |
| Fig. 8 Effect of temperature on the adsorption efficiency of (a) HPAM-1 and (b) HPAM-2 on Mg(OH)2 particles. Experimental conditions: initial HPAM concentration = 20–500 mg L−1, Mg(OH)2 dosage = 0.3 M, contact time = 4 h at pH 9.5. | |
When particle surfaces and polymer segments are oppositely charged, and electrostatic attraction gives strong adsorption in solution. Therefore, it is possible that adsorption may occur as a result of the charge neutralization of the particle surfaces and polymer segments. However, attachment of polymer segments on particle surfaces occurs at a temperature that depends on polymer concentrations and hydrophilicity/hydrophobicity of polymer. On one hand, the hydrophobicity of polymer increases with an increase in molecular weight of polymer. On the other hand, the reaction temperature of system will significantly affect the dehydration of polymer. At higher reaction temperature, the polymer with a higher molecular weight has higher degree of dehydration than that of lower molecular weight of polymer. For these reasons, the hydrophobicity of HPAM-2 molecules was higher than that of HPAM-1 molecules. HPAM-1 molecules tend to remain in water, while HPAM-2 molecules could be precipitated from water and easily adsorbed at the solid–liquid interface. In this case, co-precipitation/adsorption process occurs, and polymer molecules directly pack into host Mg(OH)2 crystals, through electrostatic attraction, intermolecular hydrogen bonds as well as hydrophobic interactions. Therefore, the reaction temperature of system has stronger influence on the adsorption efficiency of HPAM-2 than the HPAM-1.
Adsorption isotherm. The adsorbed amount of HPAM molecules on the in situ formed Mg(OH)2 particles at pH 9.5 was determined as a function of the concentration of HPAM in solution, and the results are logarithmically plotted in Fig. 9. The adsorption isotherms could be roughly divided into four regions.52–54 For adsorption data obtained at constant ionic strength and the results are plotted on a logarithmic basis, Somasundaran and Fuerstenau55 found that hydrocarbon chain–chain interactions result in several distinct regions in adsorption isotherms. The advantage of the logarithmic curve is that it “magnifies” the initial part of the adsorption isotherms.53,54 The variations in the slope of the different regions of the adsorption isotherms indicate different adsorbed layer formation (Fig. 10).53,54 In region I, for both HPAM-1 and HPAM-2, the adsorbed amount only slowly increased with an increase of polymer concentration. The small variation in the slope for region I suggests that there might be some specific interactions between polymer segments and particle surfaces due to the nature of the polar groups on polymer chains. HPAM-1 and HPAM-2 have some functional groups (e.g., carboxylic, amide and amino groups) that can easily interact with hydroxyl groups on the particle surfaces to form hydrogen bonds. In region II, a sharp increase in the slope showed a high level surface coverage of HPAM molecules on Mg(OH)2 particles. This high level of coverage is usually attributed to charge neutralization of the particle surfaces. When negatively charged polymer molecules adsorb on the oppositely charged particle surfaces, polymer chains may continuously change configuration so that most charged sites on the polymer chains are bonded to the particle surfaces. An adsorbed polymer monolayer is formed on Mg(OH)2 by electrostatic attraction. In region III, a second layer begins to form on the previously adsorbed monolayer via chain–chain interactions, van der Waals forces and intermolecular hydrogen bonds. Chain association of adsorbed polymer segments may effectively increase surface interaction sites and adsorption affinity. Therefore, there is a strong association between adsorbed polymer monolayers. In addition, with an increase in the amount of in situ formed Mg(OH)2 particles, these particles can easily form aggregates. During this process, adsorbed polymer molecules may directly pack into Mg(OH)2 aggregates. In this case, co-precipitation/adsorption process occurs. Beyond this region, adsorption reaches almost equilibrium. In region IV, when the amount of adsorbed polymer reached a critical concentration, the adsorbed polymer layers may be sufficient to cause charge reversal of the particle surfaces and lead to electrostatic repulsion between the similarly charged polymer molecules and particle surfaces. The polymer molecules must overcome an electrostatic barrier to reach the particle surfaces. Thus, it can be concluded that the equilibrium is a result of the energy barrier for the adsorption process in the form of structural and electrostatic components.52–54 Adsorption remains almost constant in this region.
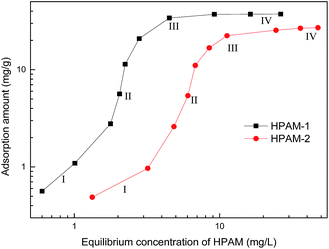 |
| Fig. 9 Adsorption isotherms of polymers on Mg(OH)2 particles at 40 °C and pH 9.5. | |
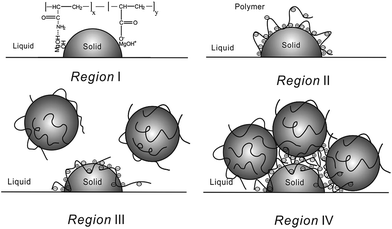 |
| Fig. 10 Schematic representation of HPAM adsorbed to Mg(OH)2 particles. | |
The maximum adsorbed amount of the HPAM varies with polymer molecular weight for a variety of reasons. (1) As HPAM molecules have some functional groups such as carboxylic, amide and amino groups on the polymer chains, the hydrolysis degree and negative charge density of the HPAM increases with increasing molecular weight of polymer. Therefore, HPAM-2 which has higher molecular weight tends to remain in bulk phase. (2) The size of HPAM-2 was larger than that of HPAM-1, leading to a decrease in the diffusion rate of the HPAM-2 molecules from the bulk phase to the solid–liquid interface. (3) Because of the differences in the polarity of the polymer segments and particles, re-configuration of adsorbed HPAM at the interface occurs until an equilibrium state reached. The HPAM-1 molecules arrive at the interface so rapidly that high surface coverage is achieved forming a denser adsorbed layer during re-configuration process, while HPAM-2 has a lower surface coverage. For these reasons, the adsorbed amount of HPAM decreased with an increase of the molecular weight.
4. Conclusions
In situ formed Mg(OH)2 particles used as an adsorbent in this study were synthesized by a simple direct wet precipitation method at controlled temperature. The present study was devoted to investigate the efficiency of in situ formed Mg(OH)2 particles for the removal of HPAM from aqueous solution. The pHpzc of the Mg(OH)2 particles was around 12.3 in 10−3 M NaCl solution. The optimum pH for HPAM adsorption was 9.5. At pH 9.5, the predominant species were Mg2+, MgOH+, and Mg(OH)2,45,47,48 and there were strong electrostatic attraction between the predominant species and polymer segments. Polymer segments can easily adsorb on oppositely charged Mg(OH)2 particle surfaces. The adsorption of HPAM on the Mg(OH)2 equilibrated within 4 h. The experimental data showed the adsorption process of HPAM on the Mg(OH)2 particles was endothermic in nature, and higher reaction temperature contributed to the adsorption process. Adsorbed polymer molecules led to the formation of four distinct adsorption regions as the concentration of polymer is increased. Moreover, changing the molecular weight of the polymer from 5.0 × 106 to 1.2 × 107 resulted in a lower adsorbed amount of HPAM on the Mg(OH)2 particles.
HPAM molecules adsorb on the Mg(OH)2 particle surfaces by complex interactions. (1) The main interaction may be electrostatic attraction between polymer molecules and oppositely charged Mg(OH)2 particles. (2) There are also some non-ionic interactions that can affect adsorption. The most important of which is hydrogen bonding. HPAM have some functional groups (e.g., carboxylic, amide and amino groups) that can easily interact with hydroxyl groups on the particle surfaces via hydrogen bonding. (3) The other important interaction is bridging. The bridging of the particles is more effective when more polymers are adsorbed.29,31 When particles with adsorbed polymer layers formed a three-dimensional network, co-precipitation/adsorption process occurred. In this case, the HPAM could be effectively removed from aqueous solution.
Acknowledgements
This work was supported by the National Natural Science Foundation of China (Grant no. 51178253 and 21333005). Thanks to Dr Edward C. Mignot, Shandong University, for linguistic advice.
Notes and references
- N. L. McFarlane, N. J. Wagner, E. W. Kaler and M. L. Lynch, Langmuir, 2010, 26, 13823–13830 CrossRef CAS PubMed.
- J. Zhang and J. Buffle, J. Colloid Interface Sci., 1995, 174, 500–509 CrossRef CAS.
- I. Ostolska and M. Wisniewska, RSC Adv., 2015, 5, 28505–28514 RSC.
- A. B. Zezin, S. V. Mikheikin, V. B. Rogacheva, M. F. Zansokhova, A. V. Sybachin and A. A. Yaroslavov, Adv. Colloid Interface Sci., 2015, 226, 17–23 CrossRef CAS PubMed.
- S. Zha, J. Yu, G. Zhang, N. Liu and R. Lee, RSC Adv., 2015, 5, 105692–105698 RSC.
- B. J. Lee, M. A. Schlautman, E. Toorman and M. Fettweis, Water Res., 2012, 46, 5696–5706 CrossRef CAS PubMed.
- A. Giwa, N. Akther, V. Dufour and S. W. Hasan, RSC Adv., 2016, 6, 8134–8163 RSC.
- H. Gong, X. Xin, G. Xu and Y. Wang, Colloids Surf., A, 2008, 317, 522–527 CrossRef CAS.
- F. Li, W. He, D. Sun, T. Wu and Y. Li, J. Cleaner Prod., 2015, 104, 468–474 CrossRef CAS.
- F. Li, L. Sun, Y. Wang, T. Wu and Y. Li, J. Pet. Sci. Eng., 2014, 124, 155–160 CrossRef CAS.
- K. C. Taylor and H. A. Nasr-El-Din, J. Pet. Sci. Eng., 1998, 19, 265–280 CrossRef CAS.
- W. Kang, B. Xu, Y. Wang, Y. Li, X. Shan, F. An and J. Liu, Colloids Surf., A, 2011, 384, 555–560 CrossRef CAS.
- B. Bolto and J. Gregory, Water Res., 2007, 41, 2301–2324 CrossRef CAS PubMed.
- L. Miao, F. Li, D. Sun, T. Wu and Y. Li, J. Pet. Sci. Eng., 2015, 133, 18–28 CrossRef CAS.
- L. Zhang, X.-C. Wang, F. Yan, L. Luo, L. Zhang, S. Zhao and J.-Y. Yu, Colloid Polym. Sci., 2008, 286, 1291–1297 CAS.
- X. Zhao, L. Liu, Y. Wang, H. Dai, D. Wang and H. Cai, Sep. Purif. Technol., 2008, 62, 199–204 CrossRef CAS.
- J. C. Yu, A. Xu, L. Zhang, R. Song and L. Wu, J. Phys. Chem. B, 2004, 108, 64–70 CrossRef CAS.
- C. Henrist, J.-P. Mathieu, C. Vogels, A. Rulmont and R. Cloots, J. Cryst. Growth, 2003, 249, 321–330 CrossRef CAS.
- K. T. Ranjit and K. J. Klabunde, Chem. Mater., 2005, 17, 65–73 CrossRef CAS.
- J. Lv, L. Qiu and B. Qu, J. Cryst. Growth, 2004, 267, 676–684 CrossRef.
- A. Takagaki, M. Sugisawa, D. Lu, J. N. Kondo, M. Hara, K. Domen and S. Hayashi, J. Am. Chem. Soc., 2003, 125, 5479–5485 CrossRef CAS PubMed.
- Y. Ding, G. Zhang, H. Wu, B. Hai, L. Wang and Y. Qian, Chem. Mater., 2001, 13, 435–440 CrossRef CAS.
- S. Utamapanya, K.
J. Klabunde and J. R. Schlup, Chem. Mater., 1991, 3, 175–181 CrossRef CAS.
- Y. Diao, W. P. Walawender, C. M. Sorensen, K. J. Klabunde and T. Ricker, Chem. Mater., 2002, 14, 362–368 CrossRef CAS.
- Y. Li, M. Sui, Y. Ding, G. Zhang, J. Zhuang and C. Wang, Adv. Mater., 2000, 12, 818–821 CrossRef CAS.
- W. Fan, S. Sun, L. You, G. Cao, X. Song, W. Zhang and H. Yu, J. Mater. Chem., 2003, 13, 3062–3065 RSC.
- X. Lv, M. Li, X. Ma, S. Ma, Y. Gao, L. Tang, J. Zhao, Y. Guo, X. Zhao and Z. Wang, Colloids Surf., A, 2007, 296, 97–103 CrossRef CAS.
- S. H. Behrens, D. I. Christl, R. Emmerzael, P. Schurtenberger and M. Borkovec, Langmuir, 2000, 16, 2566–2575 CrossRef CAS.
- W. Lin, P. Galletto and M. Borkovec, Langmuir, 2004, 20, 7465–7473 CrossRef CAS PubMed.
- Y. Otsubo, J. Colloid Interface Sci., 1999, 215, 99–105 CrossRef CAS PubMed.
- J. Gregory and S. Barany, Adv. Colloid Interface Sci., 2011, 169, 1–12 CrossRef CAS PubMed.
- L. Hao, C. Zhu, X. Mo, W. Jiang, Y. Hu, Y. Zhu and Z. Chen, Inorg. Chem. Commun., 2003, 6, 229–232 CrossRef CAS.
- D. An, X. Ding, Z. Wang and Y. Liu, Colloids Surf., A, 2010, 356, 28–31 CrossRef CAS.
- Y. Deng, J. B. Dixon, G. N. White, R. H. Loeppert and A. S. Juo, Colloids Surf., A, 2006, 281, 82–91 CrossRef CAS.
- N. Sundaraganesan, N. Puviarasan and S. Mohan, Talanta, 2001, 54, 233–241 CrossRef CAS PubMed.
- S. Yu and G. M. Chow, J. Mater. Chem., 2004, 14, 2781–2786 RSC.
- M. Li, Z. Liu, X. Cao, B. Zhang, J. Zhang and F. Sun, Acta Pet. Sin., Pet. Process. Sect., 2012, 6, 025 Search PubMed.
- H. Schott, J. Pharm. Sci., 1981, 70, 486–489 CrossRef CAS PubMed.
- R. H. Yoon, T. Salman and G. Donnay, J. Colloid Interface Sci., 1979, 70, 483–493 CrossRef CAS.
- H. Schott, J. Pharm. Sci., 1977, 66, 1548–1550 CrossRef CAS PubMed.
- O. S. Pokrovsky and J. Schott, Geochim. Cosmochim. Acta, 2004, 68, 31–45 CrossRef CAS.
- Y. Xia, P. Yang, Y. Sun, Y. Wu, B. Mayers, B. Gates, Y. Yin, F. Kim and H. Yan, Adv. Mater., 2003, 15, 353–389 CrossRef CAS.
- S. V. Krishnan and I. Iwasaki, Environ. Sci. Technol., 1986, 20, 1224–1229 CrossRef CAS.
- P. B. Hostetler, Am. J. Sci., 1963, 261, 238–258 CrossRef CAS.
- A. Oliveira and M. Torem, Colloids Surf., A, 1996, 110, 75–85 CrossRef CAS.
- J. Gustafsson, KTH Department of Land and Water Resources Engineering, Stockholm, Sweden. Based on de Allison JD, Brown DS, Novo-Gradac KJ, MINTEQA2 ver 4, 2011 Search PubMed.
- L. Cheng, L. Ye, D. Sun, T. Wu and Y. Li, Chem.–Eng. J., 2015, 264, 672–680 CrossRef CAS.
- X. Huang, T. Wu, Y. Li, D. Sun, G. Zhang, Y. Wang, G. Wang and M. Zhang, J. Hazard. Mater., 2012, 219, 82–88 CrossRef PubMed.
- L. Yan, J. Zhuang, X. Sun, Z. Deng and Y. Li, Mater. Chem. Phys., 2002, 76, 119–122 CrossRef CAS.
- D. Xue, X. Yan and L. Wang, Powder Technol., 2009, 191, 98–106 CrossRef CAS.
- M. J. McKelvy, R. Sharma, A. V. Chizmeshya, R. Carpenter and K. Streib, Chem. Mater., 2001, 13, 921–926 CrossRef CAS.
- D. W. Fuerstenau and R. Jia, Colloids Surf., A, 2004, 250, 223–231 CrossRef CAS.
- Q. Liu, S. Zhang, D. Sun and J. Xu, Colloids Surf., A, 2010, 355, 151–157 CrossRef CAS.
- T. P. Goloub and L. K. Koopal, Langmuir, 1997, 13, 673–681 CrossRef CAS.
- P. Somasundaran and D. Fuerstenau, J. Phys. Chem., 1966, 70, 90–96 CrossRef CAS.
Footnote |
† Electronic supplementary information (ESI) available. See DOI: 10.1039/c6ra02308h |
|
This journal is © The Royal Society of Chemistry 2016 |
Click here to see how this site uses Cookies. View our privacy policy here.