DOI:
10.1039/C5RA26797H
(Paper)
RSC Adv., 2016,
6, 7139-7158
Design, synthesis and evaluation of clioquinol–ebselen hybrids as multi-target-directed ligands against Alzheimer's disease†
Received
15th December 2015
, Accepted 4th January 2016
First published on 7th January 2016
Abstract
A novel series of compounds obtained by fusing the metal-chelating agent clioquinol and the antioxidant ebselen were designed, synthesized and evaluated as multi-target-directed ligands against Alzheimer's disease (AD). Specifically, compared with their parent compounds clioquinol and ebselen, these hybrids demonstrated significant potency in inhibiting self- and Cu(II)-induced amyloid-β (Aβ) aggregation and acted as remarkable antioxidants and biometal chelators. In addition, the hybrids showed considerable improvements in ebselen-related pharmacological properties, including the ability to mimic glutathione peroxidase and scavenge H2O2. Of these molecules, compound 10h was identified as a potential lead compound for AD therapy. Importantly, this compound was found to possess rapid H2O2 scavenging activity and glutathione peroxidase-like (GPx-like) activity. Moreover, compound 10h was able to efficiently disassemble preformed self- and Cu(II)-induced Aβ aggregates. Furthermore, 10h was able to penetrate the central nervous system (CNS) and did not exhibit any acute toxicity in mice at doses up to 2000 mg kg−1.
Introduction
Alzheimer's disease (AD), a multifaceted and devastating neuro-degenerative disorder, is one of the public health challenges facing our generation. Currently, more than 44 million people suffer from AD worldwide, and the global cost is estimated at US $604 billion.1 The etiology of AD has not been fully detailed at present, but multiple factors are known to play important roles in its pathogenesis, including low levels of acetylcholine, β-amyloid (Aβ) deposits, τ-protein aggregation, oxidative stress, inflammation and dyshomeostasis of biometals.2 However, the only therapeutic options approved by the US Food and Drug Administration (FDA) are cholinesterase inhibitors (donepezil, rivastigmine, and galantamine) and an N-methyl-D-aspartate (NMDA) receptor antagonist (memantine), which result in modest improvement in AD symptoms but have little efficacy in preventing progression of the neurodegenerative disorder.3
The production of oligomeric aggregates of Aβ in the brain and the accumulation of intracellular neurofibrillary tangles are among the clearest indicators of the pathogenesis of AD, and these two events are thought to initiate the disease, ultimately leading to neuronal loss and dementia.3,4 Aβ can also efficiently generate reactive oxygen species (ROS) in the presence of some transition metals and form stable dityrosine cross-linked dimers, which are generated by free radical attack under oxidative conditions. The interaction of Aβ peptides with dysregulated redox-active metal ions, such as remarkably high levels of iron (1 mM), zinc (1 mM), and copper (0.4 mM), promote Aβ aggregation and overproduction of ROS.5 Additionally, AD patients exhibit a significant disease-dependent increase in oxidative markers due to decreased protection of neurons from mitochondrial oxidative stress, resulting in higher levels of oxidative stress.6–8 Therefore, successful inhibition of Aβ aggregation, modulation of the biometals in the brain and protection of neuronal cells from oxidative damage could potentially prevent AD.
Because of the multifactorial pathogenesis of AD, in recent years, many multitarget-directed ligands (MTDLs) acting on diverse targets have been developed for the treatment of AD.9–15 Recent studies have indicated that the strategies using MTDLs have provided a great deal of potential advantages such as improving safety (reduced risk of drug–drug interactions) and therapeutic effects (simultaneously inhibiting multiple targets) and facilitating clinical studies (reduced uncertainty in pharmacokinetics).12 The successful multitarget-directed lead compound ladostigil, which combines the carbamate moiety of rivastigmine with rasagiline, has undergone phase II clinical studies as an anti-AD agent (Fig. 1).16
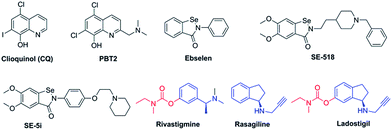 |
| Fig. 1 Structures of clioquinol, PBT2, ebselen, SE-518, SE-5i, rivastigmine, rasagiline and ladostigil. | |
Clioquinol (CQ, Fig. 1) and its second-generation analogue PBT2 (Fig. 1), 8-hydroxyquinoline derivatives with moderate affinity for Cu and Zn that inhibit metal-induced Aβ aggregation and ROS generation, have been used for the treatment of AD and have shown evidence of slowing cognitive deterioration and significantly decreasing plasma Aβ1–42 levels.17–19
The fundamental small molecule utilized in cellular antioxidant defence mechanisms is glutathione (GSH), which protects cells by reducing harmful peroxides under the catalysis of glutathione peroxidase (GPx). The small, organoselenium compound ebselen (Fig. 1), which possesses the catalytic ability to detoxify ROS, has been demonstrated to be an outstanding GPx mimic and shown significant beneficial effects in a primate model of neuro-degenerative diseases.20,21
Previously, we designed and synthesized a series of “selenpezil” compounds (SE-518 and SE-5i, Fig. 1) by fusing the pharmacophores of donepezil and ebselen, which inhibit acetylcholinesterase (AChE) and mimic GPx function.22,23 In addition, a novel series of selenium-containing clioquinol compounds, which exhibit significant antioxidation and inhibition of metal-induced Aβ aggregation effects, were also investigated in our previous study.24 Considering that both redistributing biometal and enhancing antioxidation in the brain are significant approaches for the treatment of AD, in this work (Fig. 2), we fused the pharmacophores of clioquinol and ebselen to one molecule, which does not require the major structural modifications necessary for other MTDLs but results in strong Aβ aggregation inhibitory activity and a remarkable ability to mimic the enzymatic properties of GPx.
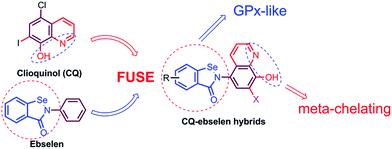 |
| Fig. 2 Drug design strategy for multi-target-directed ligands (CQ–ebselen hybrids). | |
Results and discussion
Chemistry
The synthetic route of the CQ–ebselen hybrids is described in Schemes 1–3. Commercially available quinolin-8-ol (1) first reacted with NaNO2/H2SO4 and was subsequently oxidized by HNO3 to generate 2a. Compounds 2b (X = Cl) and 2c (X = I) were prepared from 2a using previously published methods,25–27 and the phenolic hydroxy group of 2b and 2c were protected by the reacting them with di-tert-butyl dicarbonate ester to give compounds 3b (X = Cl) and 3c (X = I). Reduction of 3b and 3c with Na2S·9H2O in EtOH provided 4b (X = Cl) and 4c (X = I), respectively (Scheme 1).
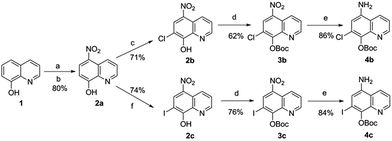 |
| Scheme 1 Synthesis of 2a–2c, 3b,3c, 4b,4c. Reagents and conditions: (a) H2O, NaNO2, H2SO4, 15–18 °C; (b) H2O, HNO3; (c) H2O, KOH, NaClO, rt; (d) CH2Cl2 : hexane = 1 : 1, (Boc)2O, DIPEA, DMAP, 50 °C, 72 h; (e) EtOH, Na2S·9H2O; (f) H2O, KOH, KI, I2, rt. | |
The target compounds were obtained through the route described in Schemes 2 and 3. First, Na2Se2 was reacted with the corresponding benzenediazonium salts, which were prepared from commercially available substituted 2-amino benzoic acids (5a–5q) and HNO2 to give 2,2-diselenobisbenzoic acids 6a–6q. Subsequent reaction with SOCl2 under reflux provided 2-chloroselenobenzoyl chlorides 7a–7q, which were reacted with the corresponding amine in anhydrous CH2Cl2 to provide key intermediates 8a–8q (X = Cl) and 9a–9q (X = I). The hydrochloride compounds 10a–10q (X = Cl) and 11a–11q (X = I) were obtained by removal of the Boc group under HCl gas.
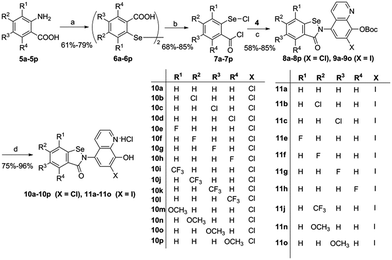 |
| Scheme 2 Synthesis of 6a–6p, 7a–7p, 8a–8p (X = Cl), 9a–9o (X = I), 10a–10p (X = Cl) and 11a–11o (X = I). Reagents and conditions: (a) H2O, NaNO2, HCl, 0–5 °C, 30 min, Na2Se2, 50 °C; (b) SOCl2, reflux; (c) anhydrous CH2Cl2, triethylamine, rt; (d) CH2Cl2, HCl (g), 0–5 °C. | |
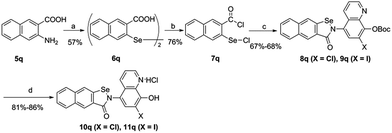 |
| Scheme 3 Synthesis of 6q, 7q, 8q (X = Cl), 9q (X = I), 10q (X = Cl) and 11q (X = I). Reagents and conditions: (a) H2O, NaNO2, HCl, 0–5 °C, 30 min, Na2Se2, 50 °C; (b) SOCl2, reflux; (c) anhydrous CH2Cl2, triethylamine, 16 h; (d) CH2Cl2, HCl (g), 0–5 °C. | |
Glutathione peroxidase-like activity and computational studies
GPx, a mammalian antioxidant enzyme, is able to decompose both metabolic and exogenous sources of ROS to H2O in the cell by using GSH or other related thiols as cofactors. Small molecular mimics of GPx have been identified as potential therapeutics in studies of anti-inflammation and neurodegenerative disease.20,21
The GPx-like activity of the clioquinol–ebselen hybrids was evaluated by the classical method of GSH–GSSG coupled reductase (see ESI†).28,29 The initial reduction rates (ν0) for hydrogen peroxide (H2O2), tert-butyl hydroperoxide (t-BuOOH) and cumene hydroperoxide (Cum-OOH) by reduced glutathione (GSH) in the presence and absence of the test compounds were calculated from a linear fit spanning the first 5–10% of the reaction by following the oxidation of reduced nicotinamide adenine dinucleotide (NADPH) in phosphate buffer at 340 nm. Most of the compounds provided much better or comparable catalytic activity compared with ebselen (Table 1, entry 29. H2O2: 124.3, t BuOOH: 27.4 and Cum-OOH: 61.3 μM min−1), SE-518 (entry 32. H2O2: 124.1, t BuOOH: 16.3 and Cum-OOH: 74.4 μM min−1) and SE-5i (entry 33. H2O2: 122.7, t BuOOH: 23.1 and Cum-OOH: 56.6 μM min−1). Specifically, the results in Table 1 show that the substituent groups, such as Cl, F and CF3, in the R4 position of the benzoselenazol-3(2H)-one moiety were indispensable for high GPx activity. For example, compounds 10d (entry 7. R4 = Cl, H2O2: 219.7, t BuOOH: 31.0 and Cum-OOH: 181.6 μM min−1), 10h (entry 14. R4 = F, H2O2: 228.0, t BuOOH: 55.0 and Cum-OOH: 172.1 μM min−1) and 10l (entry 20. R4 = CF3, H2O2: 212.8, t BuOOH: 39.5 and Cum-OOH: 163.0 μM min−1), with Cl, F and CF3 in the R4 position of the benzoselenazol-3(2H)-one moiety, exhibited much better results than other analogs with substituent groups removed or shifted to the R1, R2 or R3 position (such as 10a, entry 1; 10b,10c, entry 3, 5; 10e–10g, entry 8, 10 and 12; 10i–10k, 16, 17 and 19). However, replacement of the substituent group in the R4 position with methoxy, i.e., 10p (entry 26, R4 = OMe, H2O2: 128.4, t-BuOOH: 20.2 and Cum-OOH: 73.6 μM min−1), resulted in a substantial decrease in GPx-like activity. In addition, compounds with substituent groups in the R1 position, such as 10e (F, entry 8), 10i (CF3, entry 16), and 10m (OMe, entry 21), exhibited better catalytic activity than compounds 10f,10g (R2 or R3 = F), 10j,10k (R2 or R3 = CF3) and 10n,10o (R2 or R3 = OMe), respectively. The presence of a chlorine group on the oxine moiety was shown to be favorable for GPx-like activity compared with the presence of an iodine group. For instance, 10a (entry 1, X = Cl and R = H, H2O2: 97.8, t-BuOOH: 16.1, Cum-OOH: 47.5 μM min−1), with a chlorine group in the oxine moiety, exhibited much better catalytic activity compared with its analogue 11a (entry 2, X = I and R = H, H2O2: 59.8, t-BuOOH: 6.8, Cum-OOH: 28.1 μM min−1), which bears an iodine on the oxine moiety. The same tendency was also observed between 10e (entry 8, X = Cl and R1 = F, H2O2: 151.8, t-BuOOH: 24.3, Cum-OOH: 113.3 μM min−1) and 11e (entry 9, X = I and R1 = F, H2O2: 141.7, t BuOOH: 22.8 and Cum-OOH: 107.3 μM min−1), as well as between 10f vs. 11f, 10g vs. 11g, and 10h vs. 11h (Table 1).
Table 1 Initial rates, ν0 (μM min−1) for the reduction of peroxide by GSH in the presence of clioquinol and ebselen hybrid derivatives
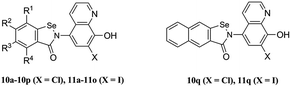
|
Entry |
Compound |
R1 |
R2 |
R3 |
R4 |
X |
GPx-like v0 (μM min−1) ± SDa |
H2O2 |
t-BuOOH |
Cum-OOH |
Assay conditions: reactions were carried out in phosphate buffer (100 mM, pH 7.5) at 25 °C, with 1 mM ethylene diamine tetraacetate (EDTA), 2 mM GSH, 0.4 mM NADPH, 1.3 unit per mL glutathione reductase (GR), 80 μM selenium compounds, and 1.6 mM peroxide. All values were determined in triplicate for the initial 10 s, and the average values with standard deviation were obtained. Control values were obtained from the reduction of peroxide by GSH in the absence of compound. |
1 |
10a·HCl |
H |
H |
H |
H |
Cl |
97.8 ± 2.1 |
16.1 ± 2.5 |
47.5 ± 2.7 |
2 |
11a·HCl |
H |
H |
H |
H |
I |
59.8 ± 3.7 |
6.8 ± 1.2 |
28.1 ± 1.8 |
3 |
10b·HCl |
H |
Cl |
H |
H |
Cl |
78.4 ± 7.4 |
20.2 ± 2.9 |
73.2 ± 3.3 |
4 |
11b·HCl |
H |
Cl |
H |
H |
I |
63.3 ± 17 |
4.3 ± 1.1 |
28.3 ± 1.6 |
5 |
10c·HCl |
H |
H |
Cl |
H |
Cl |
90.9 ± 10.6 |
9.7 ± 2.1 |
45.9 ± 2.9 |
6 |
11c·HCl |
H |
H |
Cl |
H |
I |
40.3 ± 5.8 |
5.6 ± 1.7 |
22.3 ± 3.4 |
7 |
10d·HCl |
H |
H |
H |
Cl |
Cl |
219.7 ± 3.9 |
31.0 ± 1.5 |
181.6 ± 11.6 |
8 |
10e·HCl |
F |
H |
H |
H |
Cl |
151.8 ± 10.1 |
24.3 ± 3.2 |
113.3 ± 5.6 |
9 |
11e·HCl |
F |
H |
H |
H |
I |
141.7 ± 6.7 |
22.8 ± 5.2 |
107.3 ± 5.9 |
10 |
10f·HCl |
H |
F |
H |
H |
Cl |
102.7 ± 3.1 |
14.0 ± 1.9 |
79.1 ± 2.0 |
11 |
11f·HCl |
H |
F |
H |
H |
I |
86.5 ± 1.1 |
2.0 ± 0.7 |
48.0 ± 0.5 |
12 |
10g·HCl |
H |
H |
F |
H |
Cl |
147.0 ± 0.88 |
31.6 ± 1.7 |
123.3 ± 0.9 |
13 |
11g·HCl |
H |
H |
F |
H |
I |
59.9 ± 7.5 |
12.7 ± 1.4 |
32.2 ± 3.3 |
14 |
10h·HCl |
H |
H |
H |
F |
Cl |
228.0 ± 9.8 |
55.0 ± 4.5 |
172.1 ± 3.4 |
15 |
11h·HCl |
H |
H |
H |
F |
I |
193.6 ± 4.2 |
24.7 ± 0.9 |
98.1 ± 5.1 |
16 |
10i·HCl |
CF3 |
H |
H |
H |
Cl |
103.5 ± 10.8 |
15.3 ± 1.5 |
113.2 ± 7.8 |
17 |
10j·HCl |
H |
CF3 |
H |
H |
Cl |
83.6 ± 7.4 |
3.6 ± 1.0 |
46.0 ± 4.7 |
18 |
11j·HCl |
H |
CF3 |
H |
H |
I |
65.4 ± 4.2 |
17.0 ± 6.9 |
23.4 ± 1.8 |
19 |
10k·HCl |
H |
H |
CF3 |
H |
Cl |
108.5 ± 4.1 |
13.7 ± 2.4 |
86.0 ± 7.2 |
20 |
10l·HCl |
H |
H |
H |
CF3 |
Cl |
212.8 ± 5.3 |
39.5 ± 2.4 |
163.0 ± 3.3 |
21 |
10m·HCl |
OMe |
H |
H |
H |
Cl |
155.4 ± 5.4 |
38.3 ± 0.3 |
135.5 ± 0.3 |
22 |
10n·HCl |
H |
OMe |
H |
H |
Cl |
75.5 ± 5.3 |
10.2 ± 1.2 |
40.3 ± 3.0 |
23 |
11n·HCl |
H |
OMe |
H |
H |
I |
56.2 ± 1.8 |
13.9 ± 2.0 |
18.2 ± 1.0 |
24 |
10o·HCl |
H |
H |
OMe |
H |
Cl |
76.6 ± 10.6 |
10.7 ± 1.1 |
42.9 ± 4.4 |
25 |
11o·HCl |
H |
H |
OMe |
H |
I |
30.0 ± 2.5 |
2.9 ± 1.4 |
25.4 ± 6.3 |
26 |
10p·HCl |
H |
H |
H |
OMe |
Cl |
128.4 ± 4.3 |
20.2 ± 2.9 |
73.6 ± 4.3 |
27 |
10q·HCl |
— |
— |
— |
— |
Cl |
43.1 ± 5.1 |
12.2 ± 3.3 |
11.6 ± 0.7 |
28 |
11q·HCl |
— |
— |
— |
— |
I |
44.1 ± 2.8 |
5.4 ± 0.4 |
15.7 ± 2.6 |
29 |
Ebselen |
— |
— |
— |
— |
— |
124.3 ± 3.4 |
27.4 ± 0.1 |
61.3 ± 0.1 |
30 |
Clioquinol |
— |
— |
— |
— |
— |
37.6 ± 0.3 |
4.7 ± 0.8 |
5.9 ± 1.5 |
31 |
Ebselen + clioquinol |
|
|
— |
— |
— |
126.1 ± 2.9 |
28.1 ± 0.5 |
59.9 ± 0.6 |
32 |
SE-518 |
— |
— |
— |
— |
— |
124.1 ± 6.8 |
16.3 ± 1.2 |
74.4 ± 5.2 |
33 |
SE-5i |
— |
— |
— |
— |
— |
122.7 ± 3.6 |
23.1 ± 2.8 |
56.6 ± 4.6 |
34 |
Controlb |
— |
— |
— |
— |
— |
37.2 ± 0.7 |
5.0 ± 0.8 |
5.3 ± 1.1 |
The investigations of classical GPx-like mechanism and its rate-determining step (Scheme 4) demonstrated that the weak intramolecular nonbonding Se⋯O interaction in the selenenyl sulfide was favorable for the thiol to attack the sulfur atom instead of selenium atom in the selenenyl sulfide to obtain the selenol intermediate and facilitated the GPx-like cycle.28,30–33
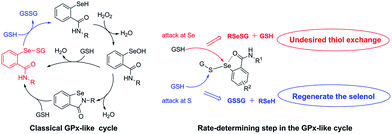 |
| Scheme 4 The classical GPx-like cycle and the rate-determining step in the classical GPx-like cycle. | |
To obtain better insight into the different GPx-like activities of the CQ–ebselen hybrids, density functional theory (DFT) calculations and natural bond orbital (NBO) analyses were carried out for the key intermediate selenenyl sulfides. The geometries of the selenenyl sulfides were fully optimized using calculations at the B3LYP/6-311+g(d) level. As indicated by the results in Table 2, weak intramolecular nonbonding Se⋯O interactions were present in the selenenyl sulfides 10d-S (R4 = Cl, 3.48 kcal mol−1), 10h-S (R4 = F, 2.27 kcal mol−1), and 10l-S (R4 = CF3, 2.79 kcal mol−1), but there was a strong Se⋯O interaction in 10p-S (R1 = OMe, 17.69 kcal mol−1). These results led to the increased GPx-like activity of 10d, 10h and 10l, but weaker activity of 10p. Weak noncovalent Se⋯O interactions were also observed in 10e-S (R1 = F, 1.15 kcal mol−1), 10i-S (R1 = CF3, 0.67 kcal mol−1) and 10m-S (R1 = OMe, 0.57 kcal mol−1). However, substituent groups in the R1 position, which were next to the selenium–sulfur bond, may have caused steric hindrance and prevented GSH from attacking the selenenyl sulfides, resulting in moderate GPx-like activity by 10e, 10i and 10m. Replacement of the chlorine group on the oxine moiety with an iodine group increased the Se⋯O interaction from 2.27 kcal mol−1 (10h-S, R4 = F, X = Cl) to 2.83 kcal mol−1 (11h-S, R4 = F, X = I), which gave rise to a decrease in GPx-like activity.
Table 2 Summary of density functional theory (DFT) calculations and natural bond orbital (NBO) analysis on the selenenyl sulfides at B3LYP/6-311+G(d,p) level on the B3LYP/6-311+G(d) level-optimized geometries
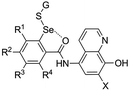
|
Selenenyl sulfides |
R |
X |
rSe⋯O [Å] |
qO⋯Se–S [°] |
qSe |
qS(−) |
ESe⋯O [kcal mol−1] |
Using Lanl2dz as the basis set for the I atom and 6-311+G(d,p) for C, H, O, N, F, S, Se atoms. |
10a-S |
R = H |
Cl |
2.609 |
175.8 |
0.366 |
0.030 |
10.38 |
10b-S |
R2 = Cl |
Cl |
2.611 |
175.8 |
0.374 |
0.026 |
13.51 |
10c-S |
R3 = Cl |
Cl |
2.618 |
175.4 |
0.371 |
0.025 |
14.74 |
10d-S |
R4 = Cl |
Cl |
2.677 |
175.4 |
0.367 |
0.027 |
3.48 |
10e-S |
R1 = F |
Cl |
2.973 |
152.9 |
0.315 |
0.010 |
1.15 |
10f-S |
R2 = F |
Cl |
2.601 |
175.8 |
0.377 |
0.028 |
14.21 |
10g-S |
R3 = F |
Cl |
2.620 |
175.5 |
0.365 |
0.025 |
15.81 |
10h-S |
R4 = F |
Cl |
2.681 |
175.8 |
0.388 |
0.035 |
2.27 |
11h-S |
R4 = F |
Ia |
2.679 |
176.0 |
0.389 |
0.038 |
2.83 |
10i-S |
R1 = CF3 |
Cl |
3.036 |
152.0 |
0.309 |
0.029 |
0.67 |
10j-S |
R2 = CF3 |
Cl |
2.625 |
175.4 |
0.375 |
0.020 |
13.56 |
10k-S |
R3 = CF3 |
Cl |
2.608 |
175.8 |
0.383 |
0.024 |
11.21 |
10l-S |
R4 = CF3 |
Cl |
2.795 |
174.2 |
0.335 |
0.005 |
2.79 |
10m-S |
R1 = OMe |
Cl |
3.124 |
144.0 |
0.299 |
0.013 |
0.57 |
10n-S |
R2 = OMe |
Cl |
2.616 |
175.9 |
0.364 |
0.028 |
13.97 |
10o-S |
R3 = OMe |
Cl |
2.628 |
175.2 |
0.355 |
0.030 |
10.41 |
10p-S |
R4 = OMe |
Cl |
2.479 |
176.4 |
0.390 |
0.047 |
17.69 |
Determination of the catalytic parameters
Kinetic experiments were conducted to further understand the catalytic behavior of the target compound, with ebselen as the control compound. Lineweaver–Burk (double reciprocal) plots for 10h were obtained by plotting the reciprocal of the initial rate (1/ν0) against the reciprocal of the substrate concentration (1/[substrate]) and were used to determine the catalytic parameters.28,29 These catalytic parameters, including maximum velocity (Vmax), Michaelis constant (KM), catalytic constant (kcat) and catalytic efficiency (η), were obtained for the reduction of H2O2, t-BuOOH and Cum-OOH in the presence of the hybrid 10h and ebselen. It is evident from Table 3 that the KM value of 10h (0.64, 1.16 and 0.75 mM at various peroxide concentrations; 0.47, 0.45 and 0.40 mM at various GSH concentrations) was much lower compared with the KM value obtained for ebselen (1.32, 3.33 and 1.11 mM at various peroxide concentrations; 1.05, 0.83 and 1.18 mM at various GSH concentrations). Furthermore, the η values that were determined for 10h at various thiol concentrations for each peroxide concentration were found to be approximately three to five times higher than those of ebselen. Overall, these observations revealed that the hybrid 10h results in significant GPx-like activity compared with ebselen.
Table 3 Effect of peroxide and GSH concentrations on Vmax (maximum velocity), KM (Michaelis constant), kcat (catalytic constant), and η (catalytic efficiency) of ebselen and 11h
Compound |
Peroxide |
Vmax (μM min−1) |
KM (mM) |
kcat (min−1) |
η (mM−1 min−1) |
Assay conditions: reactions were carried out at 25 °C in phosphate buffer (100 mM, pH 7.5) containing 2 mM GSH, 0.4 mM NADPH, 1 mM EDTA, 1.3 U mL−1 GR, variable peroxide, and 80 μM test compound. Assay conditions: reactions were carried out at 25 °C in phosphate buffer (100 mM, pH 7.5) containing variable GSH, 0.4 mM NADPH, 1 mM EDTA, 1.3 U mL−1 GR, 1.6 mM peroxide, and 80 μM test compound. |
Effect of peroxide concentrationa |
Ebselen |
H2O2a |
222.71 ± 3.46 |
1.32 ± 0.04 |
2.78 ± 0.20 |
2.10 ± 0.1 |
t-BuOOHa |
58.55 ± 3.00 |
3.33 ± 0.17 |
0.73 ± 0.03 |
0.22 ± 0.01 |
Cum-OOHa |
141.44 ± 8.10 |
1.11 ± 0.06 |
1.77 ± 0.10 |
1.59 ± 0.09 |
10h·HCl |
H2O2a |
351.52 ± 5.42 |
0.64 ± 0.11 |
4.39 ± 0.18 |
6.86 ± 0.41 |
t-BuOOHa |
102.21 ± 6.24 |
1.16 ± 0.04 |
1.28 ± 0.06 |
1.10 ± 0.08 |
Cum-OOHa |
246.54 ± 6.17 |
0.75 ± 0.03 |
3.08 ± 0.07 |
4.10 ± 0.09 |
![[thin space (1/6-em)]](https://www.rsc.org/images/entities/char_2009.gif) |
Effect of thiol concentrationb |
Ebselen |
H2O2b |
187.96 ± 2.77 |
1.05 ± 0.01 |
2.35 ± 0.03 |
2.23 ± 0.02 |
t-BuOOHb |
43.01 ± 0.60 |
0.83 ± 0.01 |
0.54 ± 0.07 |
0.65 ± 0.08 |
Cum-OOHb |
75.64 ± 4.21 |
1.18 ± 0.06 |
0.95 ± 0.05 |
0.80 ± 0.04 |
10h·HCl |
H2O2b |
290.09 ± 9.21 |
0.47 ± 0.08 |
3.62 ± 0.11 |
7.70 ± 0.23 |
t-BuOOHb |
68.83 ± 3.43 |
0.45 ± 0.03 |
0.86 ± 0.08 |
1.91 ± 0.03 |
Cum-OOHb |
201.21 ± 4.87 |
0.40 ± 0.05 |
2.51 ± 0.06 |
6.27 ± 0.15 |
Hydrogen peroxide scavenging activity
Cellular damage in the nervous system by free radical species has been implicated in AD pathology.20,34 Exogenous free radicals, generated by H2O2, are highly reactive species capable of causing damage to lipids, protein and DNA.35 Experimental evidence has indicated that oxidative stress is a key event in AD onset and progression, and ROS- and reactive nitrogen species (RNS)-mediated injury have been observed in AD brain.36 Therefore, the antioxidant effects of H2O2 detoxification by antioxidants are significant for AD patients. To further evaluate the antioxidant activity of the hybrids, compounds 10d, 10f, 10g, 10h, 10l and 11h were selected for a horseradish peroxidase assay (HRPA); clioquinol and ebselen were used as reference compounds.24 As the results in Fig. 3 indicate, all compounds exhibited significantly better scavenging activity for hydrogen peroxide than CQ, ebselen and ebselen + CQ. Compounds 10d, 10f, 10g, 10h, 10l and 11h demonstrated complete scavenging activity for hydrogen peroxide at concentrations of 80–200 μM. These results were significantly better than our previous compounds SE-518 and SE-5i, which were unable to completely scavenge H2O2 at concentrations lower than 200 μM.22,23 Furthermore, 10h and 11h maintained the effects at concentrations as low as 50 μM. In contrast, CQ, ebselen and ebselen + CQ showed low activity even at high concentrations.
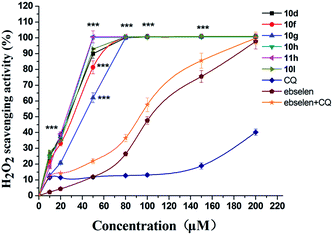 |
| Fig. 3 Scavenging effects on hydrogen peroxide in the HRPA assay (H2O2 = 100 μM). Values reported are means ± SD of three independent experiments. ***p < 0.001 in comparison to ebselen. | |
Inhibition of self-mediated Aβ1–42 aggregation
The inhibition activity of the target compounds against Aβ1–42 aggregation was determined via thioflavin T (ThT) fluorescence assay,37–41 using curcumin and resveratrol as reference compounds (Table 4). All the hybrid compounds exhibited more significant inhibition of Aβ aggregation than ebselen, CQ and ebselen + CQ. Specifically, the results demonstrated that the hybrids 10h and 11h, containing a fluorine group in the R4 position of the benzoselenazol-3(2H)-one moiety, showed better inhibition than the other hybrids (10h, IC50 = 9.6 μM; 11h, 8.1 μM). Shifting the fluorine group of the benzoselenazol-3(2H)-one moiety to R1, R2 and R3 to generate 10e–10g and 11e–11g resulted in less potent activity than the parent compounds (10e–10g gave 9.9–15.6 of the IC50 values, and 11e–11g showed 9.5–10.5 μM of the IC50 values), showing that the fluorine group in the R4 position of the benzoselenazol-3(2H)-one moiety was important. Replacement of the F in 10h and 11h with an H, Cl, CF3 or OCH3 group resulted in compounds 10a–10d, 10i–10p, 11a–11c and 11j–11o (exhibited IC50 values of 10.1–12.7, 11.1–16.6, 9.9–10.6 and 8.6–9.3 μM), which provided less potent activity than 10h and 11h. Compounds 10q and 11q, which have a naphthaselenazol-3(2H)-one structure, also exhibited weaker inhibitory activity than 10h and 11h, respectively (10q, IC50 = 13.8 μM; 11q, 8.5 μM). The presence of a halogen on the oxine moiety, especially iodine, was also shown to be important for inhibitory activity. Compounds 10h and 11h, with a chlorine and iodine group on the oxine moiety, exhibited IC50 values of 9.6 μM and 8.1 μM, respectively.
Table 4 Inhibition of Aβ1–42 aggregation, Oxygen Radical Absorbance Capacity (ORAC, Trolox equivalents)
Compound |
Inhibition of Aβ1–42 aggregation IC50a (μM) |
ORACb |
Compound |
Inhibition of Aβ1–42 aggregation IC50 (μM) SDa |
ORACb |
The thioflavin-T fluorescence method was used for the inhibition of Aβ1–42 (concentration 25 μM) aggregation. The concentration (μM) required for 50% inhibition was determined from dose–response curves. Experiments were performed in triplicate. The means ± SD of three independent experiments. Data are expressed as μmol of Trolox equivalent/μmol of tested compound. n.t. means ‘not tested’. |
10a·HCl |
11.7 ± 0.3 |
2.6 ± 0.1 |
11a·HCl |
9.9 ± 0.2 |
1.0 ± 0.1 |
10b·HCl |
11.2 ± 0.1 |
2.6 ± 0.3 |
11b·HCl |
10.6 ± 0.4 |
1.0 ± 0.1 |
10c·HCl |
10.2 ± 1.0 |
2.3 ± 0.2 |
11c·HCl |
10.5 ± 0.3 |
0.8 ± 0.1 |
10d·HCl |
10.1 ± 0.9 |
2.6 ± 0.1 |
11e·HCl |
10.3 ± 0.7 |
0.9 ± 0.1 |
10e·HCl |
12.3 ± 0.5 |
2.5 ± 0.1 |
11f·HCl |
10.5 ± 0.2 |
1.5 ± 0.3 |
10f·HCl |
9.9 ± 1.1 |
2.4 ± 0.3 |
11g·HCl |
9.5 ± 0.2 |
0.9 ± 0.1 |
10g·HCl |
15.6 ± 0.1 |
2.7 ± 0.3 |
11h·HCl |
8.1 ± 0.3 |
0.6 ± 0.2 |
10h·HCl |
9.6 ± 0.4 |
2.6 ± 0.1 |
11j·HCl |
9.3 ± 0.2 |
0.9 ± 0.2 |
10i·HCl |
15.3 ± 1.6 |
2.5 ± 0.2 |
11n·HCl |
8.9 ± 0.5 |
1.3 ± 0.2 |
10j·HCl |
14.2 ± 0.3 |
2.3 ± 0.4 |
11o·HCl |
8.6 ± 0.4 |
0.9 ± 0.1 |
10k·HCl |
16.6 ± 1.3 |
2.4 ± 0.1 |
11q·HCl |
8.5 ± 0.5 |
1.3 ± 0.1 |
10l·HCl |
12.1 ± 0.7 |
2.6 ± 0.3 |
CQ |
>50 |
0.6 ± 0.1 |
10m·HCl |
14.7 ± 0.8 |
2.5 ± 0.1 |
Ebselen |
>100 |
0.2 ± 0.1 |
10n·HCl |
13.8 ± 1.6 |
2.5 ± 0.3 |
Ebselen + CQ |
>50 |
0.7 ± 0.1 |
10o·HCl |
11.1 ± 0.1 |
2.9 ± 0.1 |
Curcumin |
18.3 ± 1.3 |
n.t.c |
10p·HCl |
11.3 ± 1.7 |
2.6 ± 0.2 |
Resveratrol |
15.1 ± 1.6 |
n.t.c |
10q·HCl |
13.8 ± 0.5 |
3.5 ± 0.1 |
|
|
|
Oxygen radical absorbance capacity
The antioxidant activity of the target compounds was also determined using an oxygen radical absorbance capacity assay with fluorescein (ORAC-FL).42,43 Trolox, a vitamin E analogue responsible for capturing radicals, was used as a standard. As shown in Table 4, most of the target compounds demonstrated higher antioxidant activity (ORAC-FL values of 0.6–3.5 Trolox equivalents) than ebselen, CQ and ebselen + CQ. Notably, compound 10q with the naphthaselenazol-3(2H)-one moiety exhibited the best antioxidant activity (10q, ORAC-FL value = 3.5). The structure–activity relationship of the halogen on the oxine moiety observed with the GPx-like activity was also observed with the antioxidant activity. The presence of a halogen on the oxine moiety, especially iodine, was unfavorable for antioxidant activity. Compounds 10h and 11h, with a chlorine or iodine group on the oxine moiety, showed ORAC-FL values of 2.6 and 0.6, respectively.
Metal-chelating properties
The biometal-chelating (such as Cu2+, Zn2+, Fe2+ and Fe3+) ability of 10h was studied by UV-visible spectrometry.24 New absorption features were detected in the UV-vis spectra for compound 10h upon the addition of CuSO4 (426 nm) or ZnCl2 (263 nm) FeSO4 (260 nm) and FeCl3 (264 nm), indicating that 10h was able to interact with Cu2+/Zn2+/Fe2+/Fe3+ to form complexes (Fig. 4). The binding stoichiometry of the Cu2+–10h complex was determined by following the absorption changes at 426 nm. The presence of an isosbestic point indicated the formation of a unique Cu2+–10h complex (Fig. 4B), and titration analysis was consistent with a 1
:
2 Cu2+/ligand molar ratio (Fig. 4B inset).
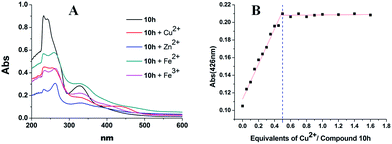 |
| Fig. 4 (A) UV absorbance spectrum of compound 10h (50 μM) alone or in the presence of CuSO4, ZnCl2, FeSO4 or FeCl3 (50 μM) in buffer (20 mM HEPES, 150 mM NaCl, pH 7.4). (B) UV-vis titration of compound 10h (80 μM) with Cu2+ in buffer (20 mM HEPES, 150 mM NaCl, pH 7.4) at room temperature. | |
Modulation of self- and metal-induced Aβ aggregation and disaggregation of Aβ aggregates by 10h
To evaluate the capacity of compound 10h to inhibit Aβ1–42 aggregation and disassemble preformed Aβ1–42 aggregates, ThT fluorescence (to measure the formation of Aβ aggregates) and transmission electron microscopy (TEM) assays (to determine the morphological changes in the Aβ species) were performed. The results in Fig. 5A show that compound 10h inhibited 65% (Fig. 5Aa) of Aβ1–42 aggregation and disaggregated 67% (Fig. 5Ab) of preformed Aβ1–42 aggregates. In contrast, CQ (30%; 19%), ebselen (less than 10%) and ebselen + CQ (32%; 20%) exhibited relatively weak effects compared with 10h. These observations were further demonstrated by TEM, and smaller and shorter Aβ fibrils were observed in the presence of 10h (Fig. 5Ac and d, sequence 5) compared with the controls, ebselen, CQ and ebselen + CQ (Fig. 5Ad and e, sequence 2, 3, 4 and 6, respectively).
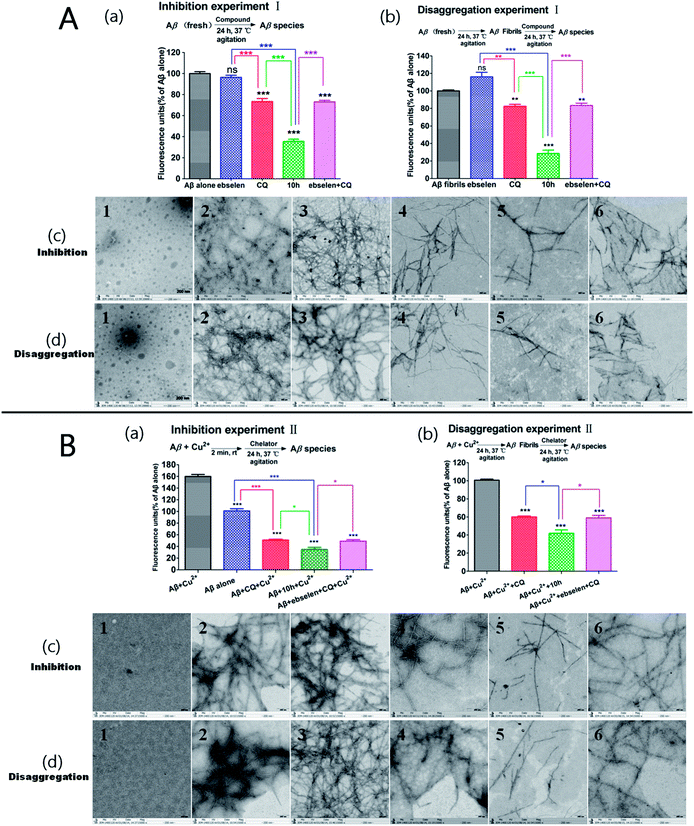 |
| Fig. 5 Inhibition and disaggregation experiments in the presence of 10h. (A) self-induced Aβ1–42 aggregation experiments including (a and b) top: scheme for the inhibition or disaggregation experiments. Bottom: The fluorescence intensity of the ThT binding assay. Statistical significance was analyzed by ANOVA: (**) p < 0.01, (***) p < 0.001. (c and d) TEM image analysis of the inhibition of Aβ1–42 aggregation and disaggregation of Aβ1–42 fibrils. Serial number: (1) fresh Aβ1–42, (2) Aβ1–42 alone, (3) Aβ1–42 + ebselen, (4) Aβ1–42 + CQ, (5) Aβ1–42 + 10h, (6) Aβ1–42 + ebselen + CQ. Experimental conditions: Aβ1–42 (25 μM); CQ, ebselen or 10h (25 μM); PBS (50 mM); pH 7.4; 37 °C. (B) Cu2+-induced Aβ1–42 aggregation experiments including (a and b) top: scheme for the inhibition or disaggregation experiments. Bottom: The fluorescence intensity of the ThT binding assay. Statistical significance was analyzed by ANOVA: (*) p < 0.05, (***) p < 0.001. (c and d) TEM image analysis of the inhibition of Cu2+-induced Aβ1–42 aggregation and disaggregation of Cu2+-induced Aβ1–42 fibrils. Serial number: (1) fresh Aβ1–42, (2) Aβ1–42 alone, (3) Aβ1–42 + Cu2+, (4) Aβ1–42 + Cu2+ + CQ, (5) Aβ1–42 + Cu2+ + 10h, (6) Aβ1–42 + ebselen + CQ. Experimental conditions: Aβ1–42 (25 μM); CuSO4 (25 μM); CQ, or 10h (50 μM); HEPES (20 μM) and NaCl (150 μM); pH 6.6; 37 °C. | |
Additionally, as indicated by the results presented in Fig. 5Ba and b, less Cu(II)-triggered Aβ aggregation was found in the presence of 10h (inhibition: 78%; disaggregation: 58%) compared with CQ (67%; 40%) and ebselen + CQ (68%; 39%). In accordance with the ThT binding assay results, TEM images indicated that more well-structured Cu(II)-induced Aβ fibrils (Fig. 5Bc and d, sequence 3) were generated compared with the samples of Aβ alone, CQ, 10h and ebselen + CQ (Fig. 5Bc and d, sequence 2, 4, 5 and 6, respectively). Overall, the results of both the ThT fluorescence and TEM assays revealed that 10h was able to control self- and Cu(II)-mediated Aβ aggregation and disassemble preformed self- and Cu(II)-induced aggregates more effectively than CQ, ebselen and ebselen + CQ.
Neuronal antioxidative effects against the production of ROS induced by t-BuOOH
To evaluate the intracellular antioxidant efficacy of the optimal compound, we carried out a cellular anti-oxidative activity assay in human SH-SY5Y cells with Trolox as the reference compound, which was able to directly determine intracellular ROS production based on the fluorescence probe of dichlorofluorescein diacetate (DCFH-DA). First, as a colorimetric MTT [3-(4,5-dimethyl-2-thiazolyl)-2,5-diphenyl-2H-tetrazolium bromide] assay showed that compound 10h (1–10 μM, 100% cell viability; 25 μM, 75%; 50 μM, 37%) did not exhibit any toxicity at 1–10 μM (Fig. 6), we used 10 μM as the maximum concentration of 10h in the intracellular antioxidant assay. As shown in Fig. 7, after the cells were treated with t-BuOOH, intracellular ROS production increased from 32% (PBS) to 100% (t-BuOOH). However, significantly less ROS production was found in the 10h-treated cells (10 μM, 61%; 5 μM, 69%; 1 μM, 82%), which also exhibited more potent intracellular antioxidant efficacy than CQ (10 μM, 90%; 5 μM, 93%; 1 μM, 97%), ebselen (10 μM, 76%; 5 μM, 82%; 1 μM, 94%) and ebselen + CQ (10 μM, 70%; 5 μM, 79%; 1 μM, 92%). These results indicate that compound 10h has the potential to be an efficient multifunctional agent, showing antioxidant activity, for the treatment of AD.
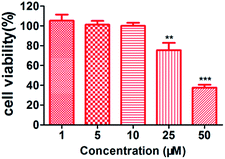 |
| Fig. 6 Effect of 10h on cell viability in SH-SY5Y cells. | |
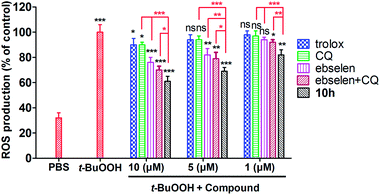 |
| Fig. 7 Inhibition of t-BuOOH-induced ROS production by Trolox, CQ, ebselen, ebselen + CQ, and 10h determined by DCFH-DA. Data are the mean ± SD of at least three independent experiments performed in sextuplicate and expressed as percent of control (cells only treated with t-BuOOH). Statistical comparisons with control (only t-BuOOH) were performed by one-way ANOVA (levels of significance *p < 0.05; **p < 0.01; ***p < 0.001, ns p > 0.05). | |
Blood–brain barrier permeation assay
To evaluate the brain penetration of the hybrids, a parallel artificial membrane permeability (PAMPA) assay, as previously described, was used for a blood–brain barrier (PAMPA-BBB) assay.24 The in vitro permeability (Pe) of target compounds and 13 commercial drugs through a porcine polar brain lipid were detected using a mixture of PBS and ethanol at a ratio of 70
:
30 (Table S13, see ESI†). A plot of experimental permeability versus reported values was determined for the assay validation, which produced a good linear correlation, Pe(exp) = 1.4574Pe(bibil) − 1.0773 (R2 = 0.9427) (see ESI, Fig. S1†). From this equation, and considering the limit established by Di et al. for blood–brain barrier permeation, we were convinced that compounds with permeabilities > 4.7 × 10−6 cm s−1 could cross the blood–brain barrier (see ESI, Table S14†). On the basis of the measured permeability, which is presented in Table 5, most of the compounds would be expected to be able to cross the blood–brain barrier. The structure–activity relationship study showed that compounds 10b–10l and 10q, which have hydrophobic groups, such as fluorine, chlorine, trifluoromethyl and naphthaline, on the benzoselenazol-3(2H)-one moiety, had much higher Pe values. However, for compounds with weak hydrophobic groups, such as 10a, and 10m–10p, the Pe values were slightly lower. The effect of halogens, especially chlorine, on the oxine moiety was favorable for brain penetration. We expected that compound 10h, which contains a chlorine group on the oxine, would be able to penetrate the BBB and reach its biological targets in the CNS, whereas the ability of 11h to penetrate the BBB was questionable. Therefore, given that brain penetration is the primary requirement for successful anti-AD drugs, 10h was chosen for further evaluation.
Table 5 Permeability (Pe × 10−6 cm s−1) determined by the PAMPA-BBB assay for target compounds and predicted penetration of the CNS
Compounda |
Peb (×10−6 cm s−1) |
Prediction |
Compounda |
Peb (×10−6 cm s−1) |
Prediction |
Compounds were dissolved in DMSO at 5 mg mL−1 and diluted with PBS/EtOH (70 : 30). Values are expressed as the means ± SD of three independent experiments, compounds with permeabilities Pe > 4.7 × 10−6 cm s−1 could cross the blood–brain barrier by passive diffusion. |
10a·HCl |
8.6 ± 0.1 |
CNS+ |
10p·HCl |
3.1 ± 0.6 |
CNS± |
10b·HCl |
14.3 ± 0.5 |
CNS+ |
10q·HCl |
20.1 ± 1.0 |
CNS+ |
10c·HCl |
14.6 ± 0.1 |
CNS+ |
11a·HCl |
8.1 ± 0.1 |
CNS+ |
10d·HCl |
7.1 ± 0.4 |
CNS+ |
11b·HCl |
10.3 ± 0.1 |
CNS+ |
10e·HCl |
12.1 ± 0.2 |
CNS+ |
11c·HCl |
10.2 ± 0.2 |
CNS+ |
10f·HCl |
12.9 ± 0.1 |
CNS+ |
11e·HCl |
11.3 ± 0.2 |
CNS+ |
10g·HCl |
13.2 ± 0.1 |
CNS+ |
11f·HCl |
7.8 ± 0.2 |
CNS+ |
10h·HCl |
6.4 ± 0.1 |
CNS+ |
11g·HCl |
10.3 ± 0.2 |
CNS+ |
10i·HCl |
12.8 ± 0.7 |
CNS+ |
11h·HCl |
4.5 ± 0.2 |
CNS± |
10j·HCl |
14.2 ± 0.1 |
CNS+ |
11j·HCl |
13.6 ± 0.2 |
CNS+ |
10k·HCl |
13.6 ± 0.2 |
CNS+ |
11n·HCl |
10.2 ± 0.6 |
CNS+ |
10l·HCl |
8.7 ± 0.6 |
CNS+ |
11o·HCl |
7.3 ± 0.1 |
CNS+ |
10m·HCl |
4.9 ± 0.3 |
CNS+ |
11q·HCl |
9.8 ± 0.2 |
CNS+ |
10n·HCl |
10.7 ± 0.4 |
CNS+ |
Hydrocortisone |
1.9 ± 0.1 |
CNS− |
10o·HCl |
10.2 ± 0.2 |
CNS+ |
Chlorpromazine |
5.8 ± 0.3 |
CNS+ |
Acute toxicity of compound 10h
The acute toxicity profile is considered a very important criterion in the development of new drugs. Compound 10h, which was perhaps the most promising multifunctional anti-AD agent, was evaluated using this assay. Twenty KM mice (common closed-colony mice that are most widely used in biomedical research in China) were randomly divided into four groups, and the test compound was given in single oral doses of 0, 677, 1333, or 2000 mg kg−1. After administration of the compound, mice were observed continuously for the first 4 h for any abnormal behavior and mortality changes, intermittently for the next 24 h, and occasionally thereafter for 14 days for the onset of any delayed effects. All mice were sacrificed on the 14th day of drug administration and examined macroscopically for possible damage to the heart, liver, and kidneys. Animals treated with compound 10h did not show any acute toxicity or mortality either immediately or during the post-treatment period. Furthermore, no significant abnormal changes were observed during the experimental period in terms of water or food consumption or body weight. Therefore, compound 10h was non-toxic and well tolerated at doses of up to 2000 mg kg−1.
Conclusions
In conclusion, our study involved the synthesis of a new series of multi-target-directed ligands for the treatment of AD that were designed as fusions of clioquinol and ebselen. These compounds were evaluated as potential inhibitors of Aβ1–42 aggregation, GPx mimics, superfast antioxidant catalysts against H2O2, and metal-chelating ligands. Of the synthesized compounds, 10h exhibited the most potent inhibition of Aβ1–42 aggregation, significant GPx-like activity, excellent antioxidation, and disaggregation of Aβ fibrils generated by self- and Cu(II)-induced Aβ aggregation. According to the results of an in vitro blood–brain barrier model assay, this compound was able to penetrate the CNS, and toxicity tests in mice showed that 10h had no acute toxicity at doses of up to 2000 mg kg−1. These properties highlight the potential of compound 10h to be a lead compound for the treatment of AD.
Experimental
The NMR spectra were recorder using TMS as the internal standard on a Bruker AvanceIII spectrometer at 400.132 (1H NMR) and 100.614 (13C NMR) MHz. MS spectra were generated on an Agilent LC-MS 6120 instrument with an ESI and APCI mass selective detector. The melting points were determined using an SRS-Opti Melt automated melting point instrument. The reactions were followed by thin-layer chromatography (TLC) on glass-packed precoated silica gel plates and visualized in an iodine chamber or with a UV lamp. Flash column chromatography was performed using silica gel (200–300 mesh) purchased from Qingdao Haiyang Chemical Co. Ltd. The high-resolution mass spectra were obtained using a Shimadzu LCMS-IT-TOF or LTQ Orbitrap XL (Thermo Scientific) mass spectrometer. The purity (≥95%) of the samples was determined by HPLC, conducted on a Shimadzu LC-20AT series system, TC-C18 column (4.6 × 250 mm, 5 μm), eluted with CH3OH/PBS (25 mM NaH2PO4 pH 3.0), 30/70, at a flow rate of 0.5 mL min−1.
5-Nitroquinolin-8-ol (2a)
Compound 2a was synthesised according to the literature.25,27 Yellow solid, 80% yield. Rf = 0.30 (CH2Cl2/CH3OH = 20/1). 1H NMR (400 MHz, DMSO-d6) δ 9.14 (d, J = 8.8 Hz, 1H), 9.02 (d, J = 3.5 Hz, 1H), 8.54 (d, J = 8.7 Hz, 1H), 7.88 (dd, J = 8.7, 3.9 Hz, 1H), 7.21 (d, J = 8.8 Hz, 1H). 13C NMR (101 MHz, DMSO-d6) δ 160.91, 149.08, 137.35, 134.80, 132.35, 129.06, 125.17, 122.57, 110.02. LC/MS (ESI): 189.0 [M − H]−.
7-Chloro-5-nitroquinolin-8-ol (2b)
Compound 2b was synthesised according to the literature.26 2a (12.0 g, 63.1 mmol) and KOH (3.5 g, 63.1 mmol) was dissolved in 1000 mL distilled water, 100 mL of NaClO (7.5%) solution was added dropwise at room temperature. The reaction was monitored by TLC, after the completion of the reaction, the solution was adjusted to pH = 6.0 with acetic acid, filtrated, the precipitation was washed with 400 mL distilled water, dried in the air to get the product as a yellow solid. 71% yield. Rf = 0.32 (CH2Cl2/CH3OH = 20/1). 1H NMR (400 MHz, DMSO-d6) δ 9.34 (d, J = 8.5 Hz, 1H), 8.99 (d, J = 3.7 Hz, 1H), 8.64 (s, 1H), 7.97 (dd, J = 8.8, 4.4 Hz, 1H). 13C NMR (101 MHz, DMSO-d6) δ 159.14, 147.69, 136.05, 135.77, 131.41, 130.06, 125.79, 122.71, 115.71. LC/MS (ESI): 223.0 [M − H]−.
7-Iodo-5-nitroquinolin-8-ol (2c)
Compound 2c was synthesised according to the literature.25 2a (12.0 g, 63.1 mmol) and KOH (10.6 g, 189.5 mmol) was dissolved in 1000 mL distilled water. A mixture of I2 (24.0 g, 94.6 mmol) in KI (15.7 g, 94.6 mmol) was added in portion at room temperature. After the completion of the reaction, the product was precipitated out by adjusted pH = 6.0 with acetic acid, filtrated, washed with 500 mL distilled water, dried in the air. Yellow solid, 74% yield. Rf = 0.34 (CH2Cl2/CH3OH = 20/1). 1H NMR (400 MHz, DMSO-d6) δ 9.28 (d, J = 8.6 Hz, 1H), 8.92 (d, J = 3.6 Hz, 1H), 8.83 (s, 1H), 7.92 (dd, J = 8.8, 4.3 Hz, 1H). 13C NMR (101 MHz, DMSO-d6) δ 162.58, 147.88, 137.91, 135.70, 134.30, 133.17, 125.95, 123.42, 79.40. LC/MS (ESI): 314.9 [M − H]−.
General procedures for the synthesis of 3b,3c
DMAP (2.10 g, 17.20 mmol) and DIPEA (12 mL, 68.90 mmol) were added at room temperature to a stirred suspension of nitroxoline (10.0 g, 34.45 mmol) and di-tert-butyl dicarbonate (15.03 g, 68.90 mmol) in a 1
:
2 mixture of hexanes–DCM (500 mL). The mixture was stirred for 72 h at 50 °C, filtered, and concentrated under reduced pressure to provide a crude product which was purified by flash column chromatography on silica gel (eluent
:
petroleum ether/DCM = 8
:
2).
tert-Butyl(7-chloro-5-nitroquinolin-8-yl)carbonate (3b). Yellow solid, 62% yield. Rf = 0.58 (petroleum/EtOAc = 10/1). 1H NMR (400 MHz, CDCl3) δ 9.05 (d, J = 8.8 Hz, 2H), 8.50 (s, 1H), 7.62 (dd, J = 8.7, 4.1 Hz, 1H), 1.59 (s, 9H). 13C NMR (101 MHz, CDCl3) δ 156.60, 150.93, 145.35, 139.91, 132.26, 128.07, 127.26, 123.75, 121.40, 88.22, 29.86.
tert-Butyl(7-iodo-5-nitroquinolin-8-yl)carbonate (3c). Yellow solid, 76% yield. Rf = 0.67 (petroleum/EtOAc = 10/1). 1H NMR (400 MHz, CDCl3) δ 9.04 (dd, J = 8.7, 1.3 Hz, 1H), 8.98 (d, J = 2.5 Hz, 1H), 8.82 (s, 1H), 7.64 (dd, J = 8.8, 4.1 Hz, 1H), 1.66 (s, 9H). 13C NMR (101 MHz, CDCl3) δ 161.82, 150.24, 143.59, 140.13, 135.31, 132.45, 123.98, 122.51, 92.06, 89.15, 30.69.
General procedures for the synthesis of 4b,4c
To a solution of tert-butyl(7-chloro-5-nitroquinolin-8-yl)carbonate (3b) or tert-butyl(7-iodo-5-nitroquinolin-8-yl)carbonate (3c) (1.0 equiv.) in 20 mL of ethanol was added 5.0 equiv. of Na2S·9H2O in one portion. The mixture was stirred at room temperature for 3 h.
5-Amino-7-chloroquinolin-8-yl tert-butyl carbonate (4b). Yellow solid, 86%Yield. Rf = 0.54 (petroleum/EtOAc = 5/1). 1H NMR (400 MHz, CDCl3) δ 8.89 (dd, J = 4.1, 1.4 Hz, 1H), 8.08 (dd, J = 8.5, 1.5 Hz, 1H), 7.30 (dd, J = 8.5, 4.1 Hz, 2H), 6.81 (s, 1H), 1.50 (s, 9H). 13C NMR (101 MHz, CDCl3) δ 149.89, 146.16, 142.36, 137.93, 129.77, 129.62, 119.60, 118.72, 111.46, 83.60, 29.65. LC/MS (ESI): 251.1 [M − CO2 + H]+.
5-Amino-7-iodoquinolin-8-yl tert-butyl carbonate (4c). Yellow solid, 84% yield. Rf = 0.67 (petroleum/EtOAc = 5/1). 1H NMR (400 MHz, CDCl3) δ 8.86 (dd, J = 4.0, 1.5 Hz, 1H), 8.08 (dd, J = 8.5, 1.5 Hz, 1H), 7.34 (dd, J = 8.5, 4.1 Hz, 1H), 7.23 (s, 1H), 3.96 (s, 2H), 1.57 (s, 9H). 13C NMR (101 MHz, CDCl3) δ 149.25, 147.65, 144.54, 138.43, 130.78, 129.91, 119.92, 119.51, 96.58, 84.30, 30.45. LC/MS (ESI): 343.0 [M − CO2 + H]+.
General procedures for the synthesis of 6a–6q and 7a–7q
Compound 6a–6q and 7a–7q were synthesized according to literature procedures.44
General procedures for the synthesis of 8a–8q (X = Cl) and 9a–9q (X = I)
To a solution of 20 mL of anhydrous DCM, 10.0 equiv. of anhydrous triethylamine and 1.0 equiv. of 4b or 4c, a solution of 1.5 equiv. of the corresponding chloride in 2 mL of anhydrous DCM was added dropwise. The reaction was stirred at room temperature for 16 h. Saturated NaHCO3 was added to the mixture, which was then extracted with DCM, washed with brine, dried over anhydrous Na2SO4, and concentrated under reduced pressure. The residue was purified by silica-column chromatography.
tert-Butyl(7-chloro-5-(3-oxobenzo[d][1,2]selenazol-2(3H)-yl)quinolin-8-yl)carbonate (8a). Pale yellow solid, 69% yield. Rf = 0.53 (petroleum/EtOAc = 3/1). 1H NMR (400 MHz, CDCl3) δ 8.92 (d, J = 2.6 Hz, 1H), 8.13 (d, J = 7.7 Hz, 1H), 7.99 (d, J = 8.5 Hz, 1H), 7.72 (d, J = 7.9 Hz, 1H), 7.69–7.63 (m, 2H), 7.50 (t, J = 7.4 Hz, 1H), 7.35 (dd, J = 8.5, 4.0 Hz, 1H), 1.57 (s, 9H). 13C NMR (101 MHz, CDCl3) δ 167.16, 151.30, 150.50, 146.05, 139.24, 132.89, 132.05, 129.69, 129.61, 129.51, 128.86, 126.77, 126.06, 125.68, 124.25, 121.64, 85.96, 29.85. LC/MS (ESI): 433.0 [M − CO2 + H]+.
tert-Butyl(7-chloro-5-(6-chloro-3-oxobenzo[d][1,2]selenazol-2(3H)-yl)quinolin-8-yl)carbonate (8b). Pale yellow solid, 63% yield. Rf = 0.34 (petroleum/EtOAc = 5/1). 1H NMR (400 MHz, CDCl3) δ 8.95 (dd, J = 4.1, 1.6 Hz, 1H), 8.06 (d, J = 8.4 Hz, 1H), 7.98 (dd, J = 8.5, 1.7 Hz, 1H), 7.74 (d, J = 1.4 Hz, 1H), 7.67 (s, 1H), 7.50 (dd, J = 8.4, 1.8 Hz, 1H), 7.39 (dd, J = 8.5, 4.1 Hz, 1H), 1.59 (s, 9H). 13C NMR (101 MHz, CDCl3) δ 166.25, 151.54, 150.61, 146.06, 140.35, 139.70, 131.86, 130.41, 129.67, 129.22, 128.91, 127.66, 125.97, 124.21, 124.11, 121.76, 86.14, 29.85. LC/MS (ESI): 467.0 [M − CO2 + H]+.
tert-Butyl(7-chloro-5-(5-chloro-3-oxobenzo[d][1,2]selenazol-2(3H)-yl)quinolin-8-yl)carbonate (8c). Pale yellow solid, 74% yield. Rf = 0.33 (petroleum/EtOAc = 5/1). 1H NMR (400 MHz, CDCl3) δ 8.94 (d, J = 2.9 Hz, 1H), 8.12 (s, 1H), 7.97 (d, J = 8.0 Hz, 1H), 7.66 (d, J = 4.7 Hz, 3H), 7.38 (dd, J = 8.5, 4.1 Hz, 1H), 1.58 (s, 9H). 13C NMR (101 MHz, CDCl3) δ 165.83, 151.95, 150.53, 144.68, 142.46, 136.79, 133.68, 133.41, 132.33, 132.10, 130.93, 129.46, 129.21, 126.96, 126.90, 125.91, 125.25, 122.48, 84.79, 27.64. LC/MS (ESI): 467.0 [M − CO2 + H]+.
tert-Butyl(7-chloro-5-(4-chloro-3-oxobenzo[d][1,2]selenazol-2(3H)-yl)quinolin-8-yl)carbonate (8d). Pale yellow solid, 58% yield. Rf = 0.37 (petroleum/EtOAc = 5/1). 1H NMR (400 MHz, CDCl3) δ 8.96 (d, J = 3.9 Hz, 1H), 8.03 (dd, J = 8.5, 2.5 Hz, 1H), 7.66 (t, J = 4.1 Hz, 1H), 7.63 (dd, J = 10.6, 3.7 Hz, 1H), 7.58 (dd, J = 7.8, 4.2 Hz, 1H), 7.52 (dd, J = 7.7, 3.1 Hz, 1H), 7.41 (dd, J = 8.5, 4.2 Hz, 1H), 1.58 (s, 9H). 13C NMR (101 MHz, CDCl3) δ 164.99, 151.46, 150.54, 146.04, 141.87, 137.48, 132.57, 131.95, 129.84, 129.32, 129.09, 128.86, 126.16, 122.91, 121.78, 120.77, 86.07, 29.83. LC/MS (ESI): 467.0 [M − CO2 + H]+.
tert-Butyl(7-chloro-5-(7-fluoro-3-oxobenzo[d][1,2]selenazol-2(3H)-yl)quinolin-8-yl)carbonate (8e). Pale yellow solid, 66% yield. Rf = 0.27 (petroleum/EtOAc = 5/1). 1H NMR (400 MHz, CDCl3) δ 8.97 (dd, J = 4.1, 1.5 Hz, 1H), 8.06–7.94 (m, 2H), 7.70 (s, 1H), 7.55 (tt, J = 10.0, 5.0 Hz, 1H), 7.46 (t, J = 8.3 Hz, 1H), 7.41 (dd, J = 8.5, 4.1 Hz, 1H), 1.60 (s, 9H). 13C NMR (101 MHz, CDCl3) δ 166.17 (d, 1JC–F = 2.4 Hz), 158.76 (s), 156.31 (s), 151.63 (s), 150.65 (s), 146.06 (s), 131.85 (s), 129.74 (s), 129.20 (s), 128.99 (s), 128.91 (d, 2JC–F = 3.1 Hz), 128.18 (d, 3JC–F = 4.4 Hz), 125.97 (s), 125.21 (d, 4JC–F = 3.1 Hz), 121.82 (s), 118.58 (d, 5JC–F = 19.0 Hz), 86.12 (s), 29.89 (s). LC/MS (ESI): 451.0 [M − CO2 + H]+.
tert-Butyl(7-chloro-5-(6-fluoro-3-oxobenzo[d][1,2]selenazol-2(3H)-yl)quinolin-8-yl)carbonate (8f). Pale yellow solid, 82% yield. Rf = 0.32 (petroleum/EtOAc = 5/1). 1H NMR (400 MHz, CDCl3) δ 8.95 (dd, J = 4.0, 1.5 Hz, 1H), 8.13 (dd, J = 8.6, 5.2 Hz, 1H), 8.00 (dd, J = 8.5, 1.5 Hz, 1H), 7.67 (s, 1H), 7.44 (dd, J = 7.7, 2.0 Hz, 1H), 7.39 (dd, J = 8.5, 4.1 Hz, 1H), 7.23 (dd, J = 8.6, 2.1 Hz, 1H), 1.59 (s, 9H). 13C NMR (101 MHz, CDCl3) δ 166.57 (d, 1JC–F = 80.9 Hz), 164.42 (s), 151.51 (s), 150.60 (s), 146.07 (s), 140.81 (d, 2JC–F = 10.2 Hz), 131.91 (s), 131.49 (d, 3JC–F = 9.8 Hz), 129.71 (s), 129.13 (d, 4JC–F = 44.2 Hz), 126.04 (s), 122.03 (s), 121.74 (s), 115.42 (d, 5JC–F = 23.5 Hz), 111.22 (d, 6JC–F = 26.2 Hz), 86.12 (s), 29.86 (s). LC/MS (ESI): 451.0 [M − CO2 + H]+.
tert-Butyl(7-chloro-5-(5-fluoro-3-oxobenzo[d][1,2]selenazol-2(3H)-yl)quinolin-8-yl)carbonate (8g). Pale yellow solid, 81% yield. Rf = 0.31 (petroleum/EtOAc = 5/1). 1H NMR (400 MHz, CDCl3) δ 8.97 (dd, J = 4.1, 1.6 Hz, 1H), 8.00 (dd, J = 8.5, 1.7 Hz, 1H), 7.84 (dd, J = 8.1, 2.6 Hz, 1H), 7.70 (dd, J = 8.8, 4.4 Hz, 1H), 7.67 (s, 1H), 7.45 (td, J = 8.6, 2.7 Hz, 1H), 7.40 (dd, J = 8.5, 4.2 Hz, 1H), 1.57 (s, 9H). 13C NMR (101 MHz, CDCl3) δ 166.29 (d, 1JC–F = 3.3 Hz), 163.35 (s), 160.89 (s), 151.48 (s), 150.59 (s), 146.02 (s), 133.87 (d, 2JC–F = 2.0 Hz), 131.90 (s), 129.57 (s), 129.39 (s), 128.86 (s), 127.40 (d, 3JC–F = 8.0 Hz), 125.93 (d, 4JC–F = 2.0 Hz), 125.86 (s), 121.74 (s), 121.26 (d, 5JC–F = 24.1 Hz), 115.52 (d, 6JC–F = 23.4 Hz), 86.11 (s), 29.84 (s). LC/MS (ESI): 451.0 [M − CO2 + H]+.
tert-Butyl(7-chloro-5-(4-fluoro-3-oxobenzo[d][1,2]selenazol-2(3H)-yl)quinolin-8-yl)carbonate (8h). Pale yellow solid, 78% yield. Rf = 0.30 (petroleum/EtOAc = 5/1). 1H NMR (400 MHz, CDCl3) δ 8.95 (dd, J = 4.1, 1.6 Hz, 1H), 8.04 (dd, J = 8.5, 1.6 Hz, 1H), 7.66 (s, 1H), 7.64 (dd, J = 8.1, 4.7 Hz, 1H), 7.49 (d, J = 7.6 Hz, 1H), 7.40 (dd, J = 8.5, 4.1 Hz, 1H), 7.16 (dd, J = 9.5, 8.4 Hz, 1H), 1.59 (s, 9H). 13C NMR (101 MHz, DMSO-d6) δ 164.25 (d, 1JC–F = 3.4 Hz), 163.78 (s), 161.18 (s), 151.30 (s), 150.28 (s), 145.71 (s), 143.43 (s), 133.74 (d, 2JC–F = 8.3 Hz), 132.96 (s), 131.12 (s), 129.30 (s), 128.27 (s), 126.42 (s), 122.67 (d, 3JC–F = 3.8 Hz), 122.59 (s), 115.18 (d, 5JC–F = 11.5 Hz), 113.41 (d, 6JC–F = 19.5 Hz), 85.28 (s), 30.10 (s). LC/MS (ESI): 451.0 [M − CO2 + H]+.
tert-Butyl(7-chloro-5-(3-oxo-7-(trifluoromethyl)benzo[d][1,2]selenazol-2(3H)-yl)quinolin-8-yl)carbonate (8i). White solid, 75% yield. Rf = 0.40 (petroleum/EtOAc = 5/1). 1H NMR (400 MHz, CDCl3) δ 8.98 (dd, J = 4.1, 1.6 Hz, 1H), 8.35 (d, J = 7.6 Hz, 1H), 8.06–7.96 (m, 2H), 7.74–7.64 (m, 2H), 7.42 (dd, J = 8.5, 4.1 Hz, 1H), 1.60 (s, 9H). 13C NMR (101 MHz, CDCl3) δ 165.78 (s), 151.68 (s), 150.68 (s), 146.09 (s), 135.96 (d, 1JC–F = 2.0 Hz), 133.04 (s), 131.81 (s), 130.63 (dd, 2JC–F = 6.9, 3.4 Hz), 129.68 (s), 128.93 (s), 128.67 (s), 127.53 (s), 127.30 (s), 125.90 (s), 125.89 (dd, 3JC–F = 68.7, 34.9 Hz), 121.84 (s), 86.17 (s), 29.87 (s). LC/MS (ESI): 501.0 [M − CO2 + H]+.
tert-Butyl(7-chloro-5-(3-oxo-6-(trifluoromethyl)benzo[d][1,2]selenazol-2(3H)-yl)quinolin-8-yl)carbonate (8j). White solid, 85% yield. Rf = 0.43 (petroleum/EtOAc = 5/1). 1H NMR (400 MHz, CDCl3) δ 8.96 (dd, J = 4.1, 1.6 Hz, 1H), 8.28 (d, J = 8.1 Hz, 1H), 8.05 (s, 1H), 7.98 (dd, J = 8.5, 1.6 Hz, 1H), 7.78 (d, J = 8.1 Hz, 1H), 7.69 (s, 1H), 7.40 (dd, J = 8.5, 4.1 Hz, 1H), 1.60 (s, 9H). 13C NMR (101 MHz, CDCl3) δ 166.21 (s), 151.62 (s), 150.63 (s), 146.00 (s), 140.13 (s), 134.56 (q, 1JC–F = 32.7 Hz), 131.69 (s), 129.84 (s), 129.57 (s), 128.93 (d, 2JC–F = 9.5 Hz), 128.61 (s), 125.84 (s), 123.61 (d, 3JC–F = 3.1 Hz), 123.46 (q, 4JC–F = 273.6 Hz), 122.16 (d, 5JC–F J = 3.4 Hz), 121.82 (s), 86.19 (s), 29.78 (s). LC/MS (ESI): 501.0 [M − CO2 + H]+.
tert-Butyl(7-chloro-5-(3-oxo-5-(trifluoromethyl)benzo[d][1,2]selenazol-2(3H)-yl)quinolin-8-yl)carbonate (8k). White solid, 81% yield. Rf = 0.41 (petroleum/EtOAc = 5/1). 1H NMR (400 MHz, CDCl3) δ 8.98 (d, J = 4.0 Hz, 1H), 8.44 (s, 1H), 8.06–7.92 (m, 2H), 7.89 (d, J = 8.4 Hz, 1H), 7.70 (s, 1H), 7.42 (ddd, J = 8.5, 4.1, 0.5 Hz, 1H), 1.60 (s, 9H). 13C NMR (101 MHz, CDCl3) δ 166.14 (s), 151.68 (s), 150.64 (s), 146.05 (s), 143.39 (s), 131.74 (s), 129.67 (q, 1JC–F = 33.4 Hz), 129.64 (s), 129.03 (dd, 2JC–F = 6.5, 3.0 Hz), 128.93 (d, 3JC–F = 6.3 Hz), 126.57 (q, 4JC–F = 4.0 Hz), 126.19 (s), 125.87 (s), 125.19 (s), 123.61 (q, 5JC–F = 272.4 Hz), 121.80 (s), 86.23 (s), 29.83 (s). LC/MS (ESI): 501.0 [M − CO2 + H]+.
tert-Butyl(7-chloro-5-(3-oxo-4-(trifluoromethyl)benzo[d][1,2]selenazol-2(3H)-yl)quinolin-8-yl)carbonate (8l). White solid, 73% yield. Rf = 0.37 (petroleum/EtOAc = 5/1). 1H NMR (400 MHz, CDCl3) δ 8.96 (dd, J = 4.1, 1.6 Hz, 1H), 8.01 (dd, J = 8.5, 1.6 Hz, 1H), 7.95 (d, J = 8.1 Hz, 1H), 7.91 (d, J = 7.6 Hz, 1H), 7.80 (t, J = 7.8 Hz, 1H), 7.69 (s, 1H), 7.42 (dd, J = 8.5, 4.1 Hz, 1H), 1.58 (s, 9H). 13C NMR (101 MHz, CDCl3) δ 164.42 (s), 151.42 (s), 150.57 (s), 145.95 (s), 142.28 (s), 131.84 (s), 131.65 (s), 131.40 (dd, 1JC–F = 67.3, 33.5 Hz), 129.80 (s), 129.31 (s), 128.83 (s), 128.62 (s), 126.07 (s), 125.45 (q, 2JC–F = 6.5 Hz), 122.72 (q, 3JC–F = 274.1 Hz), 121.96 (s), 121.89 (s), 86.16 (s), 29.79 (s). LC/MS (ESI): 501.0 [M − CO2 + H]+.
tert-Butyl(7-chloro-5-(7-methoxy-3-oxobenzo[d][1,2]selenazol-2(3H)-yl)quinolin-8-yl)carbonate (8m). Pale yellow solid, 71% yield. Rf = 0.38 (petroleum/EtOAc = 5/1). 1H NMR (400 MHz, CDCl3) δ 8.95 (dd, J = 4.0, 1.6 Hz, 1H), 8.01 (dd, J = 8.5, 1.6 Hz, 1H), 7.77 (d, J = 7.7 Hz, 1H), 7.68 (s, 1H), 7.52 (t, J = 7.9 Hz, 1H), 7.39 (dd, J = 8.5, 4.1 Hz, 1H), 7.16 (d, J = 8.0 Hz, 1H), 4.02 (s, 3H), 1.60 (s, 9H). 13C NMR (101 MHz, CDCl3) δ 167.36, 154.21, 151.24, 150.54, 146.04, 132.09, 130.00, 129.56, 128.84, 128.69, 126.84, 126.04, 121.68, 121.23, 114.96, 112.71, 111.68, 85.94, 56.11, 29.88. LC/MS (ESI): 463.0 [M − CO2 + H]+.
tert-Butyl(7-chloro-5-(6-methoxy-3-oxobenzo[d][1,2]selenazol-2(3H)-yl)quinolin-8-yl)carbonate (8n). White solid, 74% yield. Rf = 0.37 (petroleum/EtOAc = 5/1). 1H NMR (400 MHz, CDCl3) δ 8.95 (d, J = 3.9 Hz, 1H), 8.11–7.98 (m, 2H), 7.67 (s, 1H), 7.39 (dd, J = 8.4, 4.2 Hz, 1H), 7.17 (d, J = 2.1 Hz, 1H), 7.07 (dd, J = 8.6, 2.1 Hz, 1H), 3.95 (s, 3H), 1.59 (s, 9H). 13C NMR (101 MHz, CDCl3) δ 166.85, 163.79, 151.26, 150.46, 146.10, 140.95, 132.13, 130.70, 129.88, 129.71, 128.87, 126.22, 121.59, 118.61, 114.96, 107.64, 85.93, 55.88, 29.86. LC/MS (ESI): 463.0 [M − CO2 + H]+.
tert-Butyl(7-chloro-5-(5-methoxy-3-oxobenzo[d][1,2]selenazol-2(3H)-yl)quinolin-8-yl)carbonate (8o). White solid, 71% yield. Rf = 0.35 (petroleum/EtOAc = 5/1). 1H NMR (400 MHz, CDCl3) δ 8.95 (dd, J = 4.0, 1.6 Hz, 1H), 8.01 (dd, J = 8.5, 1.6 Hz, 1H), 7.68 (s, 1H), 7.63 (d, J = 2.6 Hz, 1H), 7.59 (d, J = 8.7 Hz, 1H), 7.39 (dd, J = 8.6, 4.2 Hz, 1H), 7.35 (dd, J = 8.8, 2.7 Hz, 1H), 3.93 (s, 3H), 1.60 (s, 9H). 13C NMR (101 MHz, CDCl3) δ 167.14, 159.27, 151.24, 150.51, 146.02, 132.09, 130.32, 129.88, 129.50, 128.83, 126.68, 126.01, 125.13, 122.67, 121.63, 111.15, 85.95, 55.78, 29.85. LC/MS (ESI): 463.0 [M − CO2 + H]+.
tert-Butyl(7-chloro-5-(4-methoxy-3-oxobenzo[d][1,2]selenazol-2(3H)-yl)quinolin-8-yl)carbonate (8p). White solid, 82% yield. Rf = 0.24 (petroleum/EtOAc = 3/1). 1H NMR (400 MHz, CDCl3) δ 8.93 (dd, J = 4.1, 1.6 Hz, 1H), 8.06 (dd, J = 8.5, 1.6 Hz, 1H), 7.64 (s, 1H), 7.60 (t, J = 8.1 Hz, 1H), 7.37 (dd, J = 8.5, 4.1 Hz, 1H), 7.25 (d, J = 7.9 Hz, 1H), 6.93 (d, J = 8.3 Hz, 1H), 4.00 (s, 3H), 1.59 (s, 9H). 13C NMR (101 MHz, CDCl3) δ 166.00, 161.80, 151.09, 150.41, 146.03, 141.61, 133.71, 132.32, 130.03, 129.78, 128.82, 126.34, 121.60, 116.12, 113.35, 108.49, 85.84, 56.01, 29.85. LC/MS (ESI): 463.0 [M − CO2 + H]+.
tert-Butyl(7-chloro-5-(3-oxonaphtho[2,3-d][1,2]selenazol-2(3H)-yl)quinolin-8-yl)carbonate (8q). Pale yellow solid, 67% yield. Rf = 0.41 (petroleum/EtOAc = 5/1). 1H NMR (400 MHz, CDCl3) δ 8.96 (d, J = 3.9 Hz, 1H), 8.76 (s, 1H), 8.13 (s, 1H), 8.12–8.06 (m, 2H), 7.92 (d, J = 8.2 Hz, 1H), 7.74 (s, 1H), 7.72–7.65 (m, 1H), 7.64–7.56 (m, 1H), 7.40 (dd, J = 8.5, 4.1 Hz, 1H), 1.60 (s, 9H). 13C NMR (101 MHz, CDCl3) δ 167.15, 151.85, 150.57, 144.40, 142.46, 135.95, 133.02, 132.35, 131.62, 130.95, 130.44, 129.71, 129.12, 128.97, 127.21, 126.98, 126.63, 126.06, 124.08, 122.87, 122.34, 84.69, 27.65. LC/MS (ESI): 483.0 [M − CO2 + H]+.
tert-Butyl(7-iodo-5-(3-oxobenzo[d][1,2]selenazol-2(3H)-yl)quinolin-8-yl)carbonate (9a). Pale yellow solid, 73% yield. Rf = 0.37 (petroleum/EtOAc = 5/1). 1H NMR (400 MHz, CDCl3) δ 8.92 (dd, J = 4.1, 1.7 Hz, 1H), 8.22–8.13 (m, 1H), 8.04 (s, 1H), 8.02 (dd, J = 8.5, 1.7 Hz, 1H), 7.74 (dd, J = 1.5, 1.0 Hz, 1H), 7.73 (d, J = 1.0 Hz, 1H), 7.59–7.51 (m, 1H), 7.42 (dd, J = 8.5, 4.1 Hz, 1H), 1.67 (s, 9H). 13C NMR (101 MHz, CDCl3) δ 164.70, 149.88, 137.50, 135.05, 132.95, 132.45, 132.20, 131.15, 129.63, 129.12, 126.83, 125.77, 124.74, 124.13, 121.97, 94.23, 86.81, 30.67. LC/MS (ESI): 524.9 [M − CO2 + H]+.
tert-Butyl(5-(6-chloro-3-oxobenzo[d][1,2]selenazol-2(3H)-yl)-7-iodoquinolin-8-yl)carbonate (9b). Pale yellow solid, 65% yield. Rf = 0.41 (petroleum/EtOAc = 5/1). 1H NMR (400 MHz, CDCl3) δ 8.89 (d, J = 2.4 Hz, 1H), 8.03 (d, J = 8.3 Hz, 1H), 7.99 (s, 1H), 7.95 (d, J = 7.3 Hz, 1H), 7.73 (s, 1H), 7.46 (d, J = 8.3 Hz, 1H), 7.39 (dd, J = 8.4, 4.0 Hz, 1H), 1.62 (s, 9H). 13C NMR (101 MHz, CDCl3) δ 165.90, 151.71, 150.97, 148.13, 141.66, 137.07, 133.41, 133.08, 131.94, 129.24, 127.64, 127.29, 127.14, 125.37, 122.49, 120.59, 95.59, 84.15, 30.82. LC/MS (ESI): 558.9 [M − CO2 + H]+.
tert-Butyl(5-(5-chloro-3-oxobenzo[d][1,2]selenazol-2(3H)-yl)-7-iodoquinolin-8-yl)carbonate (9c). Pale yellow solid, 67% yield. Rf = 0.42 (petroleum/EtOAc = 5/1). 1H NMR (400 MHz, CDCl3) δ 8.91 (d, J = 4.0 Hz, 1H), 8.14 (s, 1H), 8.04–7.93 (m, 2H), 7.67 (dd, J = 15.6, 8.5 Hz, 2H), 7.41 (dd, J = 8.5, 4.0 Hz, 1H), 1.66 (s, 9H). 13C NMR (101 MHz, CDCl3) δ 165.94, 151.61, 150.51, 149.71, 141.49, 137.01, 136.54, 133.57, 133.30, 132.62, 132.17, 129.30, 127.00, 126.95, 125.39, 122.75, 89.98, 84.70, 27.73. LC/MS (ESI): 558.9 [M − CO2 + H]+.
tert-Butyl(5-(7-fluoro-3-oxobenzo[d][1,2]selenazol-2(3H)-yl)-7-iodoquinolin-8-yl)carbonate (9e). Pale yellow solid, 68% yield. Rf = 0.31 (petroleum/EtOAc = 5/1). 1H NMR (400 MHz, CDCl3) δ 8.95–8.89 (m, 1H), 8.04 (d, J = 1.4 Hz, 1H), 7.98 (t, J = 7.9 Hz, 2H), 7.54 (ddd, J = 12.9, 7.8, 1.5 Hz, 1H), 7.45 (d, J = 8.2 Hz, 1H), 7.43–7.39 (m, 1H), 1.66 (s, 9H). 13C NMR (101 MHz, CDCl3) δ 166.16 (d, 1JC–F = 2.4 Hz), 158.77 (s), 156.92 (s), 156.32 (s), 149.98 (s), 144.47 (s), 137.58 (s), 131.98 (s), 129.50 (s), 128.93 (d, 2JC–F = 6.1 Hz), 128.22 (d, 3JC–F = 4.5 Hz), 127.07 (s), 125.22 (d, 4JC–F = 3.0 Hz), 122.09 (s), 118.56 (d, 5JC–F = 19.0 Hz), 94.19 (s), 86.92 (s), 30.69 (s). LC/MS (ESI): 543.0 [M − CO2 + H]+.
tert-Butyl(5-(6-fluoro-3-oxobenzo[d][1,2]selenazol-2(3H)-yl)-7-iodoquinolin-8-yl)carbonate (9f). Pale yellow solid, 69%yield. Rf = 0.37 (petroleum/EtOAc = 5/1). 1H NMR (400 MHz, CDCl3) δ 8.91 (dd, J = 4.0, 1.6 Hz, 1H), 8.12 (dd, J = 8.6, 5.2 Hz, 1H), 8.01 (s, 1H), 7.98 (dd, J = 8.5, 1.6 Hz, 1H), 7.47–7.36 (m, 2H), 7.23 (dd, J = 8.6, 2.2 Hz, 1H), 1.65 (s, 9H). 13C NMR (101 MHz, CDCl3) δ 166.53 (d, 1JC–F = 87.4 Hz), 164.42 (s), 156.80 (s), 149.88 (s), 144.47 (s), 140.76 (d, 2JC–F = 10.2 Hz), 137.55 (s), 132.02 (s), 131.49 (d, 3JC–F = 9.8 Hz), 129.66 (s), 127.15 (s), 121.98 (s), 115.38 (d, 4JC–F = 23.6 Hz), 111.16 (d, 5JC–F = 26.2 Hz), 94.13 (s), 86.87 (s), 30.64 (s). LC/MS (ESI): 542.9 [M − CO2 + H]+.
tert-Butyl(5-(5-fluoro-3-oxobenzo[d][1,2]selenazol-2(3H)-yl)-7-iodoquinolin-8-yl)carbonate (9g). Pale yellow solid, 75% yield. Rf = 0.38 (petroleum/EtOAc = 5/1). 1H NMR (400 MHz, CDCl3) δ 8.92 (dd, J = 4.1, 1.6 Hz, 1H), 8.02 (s, 1H), 7.99 (dd, J = 8.5, 1.6 Hz, 1H), 7.86 (dd, J = 8.1, 2.6 Hz, 1H), 7.69 (dd, J = 8.7, 4.4 Hz, 1H), 7.49 (td, J = 8.5, 2.7 Hz, 1H), 7.42 (dd, J = 8.5, 4.1 Hz, 1H), 1.66 (s, 9H). 13C NMR (101 MHz, CDCl3) δ 166.10 (d, 1JC–F = 2.8 Hz), 163.39 (s), 160.93 (s), 156.82 (s), 149.94 (s), 144.45 (s), 137.45 (s), 133.58 (s), 132.03 (s), 129.67 (s), 127.40 (d, 2JC–F = 7.9 Hz), 127.01 (s), 125.64 (d, 3JC–F = 7.5 Hz), 122.03 (s), 121.35 (d, 4JC–F = 24.2 Hz), 115.73 (d, 5JC–F = 23.4 Hz), 94.17 (s), 86.92 (s), 30.66 (s). LC/MS (ESI): 542.9 [M − CO2 + H]+.
tert-Butyl(5-(4-fluoro-3-oxobenzo[d][1,2]selenazol-2(3H)-yl)-7-iodoquinolin-8-yl)carbonate (9h). Pale yellow solid, 65% yield. Rf = 0.35 (petroleum/EtOAc = 5/1). 1H NMR (400 MHz, CDCl3) δ 8.91 (d, J = 3.6 Hz, 1H), 8.09–7.96 (m, 2H), 7.65 (td, J = 7.9, 4.7 Hz, 1H), 7.48 (d, J = 7.9 Hz, 1H), 7.42 (dd, J = 8.4, 4.1 Hz, 1H), 7.21–7.12 (m, 1H), 1.66 (s, 9H). 13C NMR (101 MHz, DMSO-d6) δ 164.26 (d, 1JC–F = 3.6 Hz), 163.77 (s), 161.18 (s), 155.60 (s), 150.53 (s), 144.27 (s), 143.34 (s), 137.12 (s), 133.62 (d, 2JC–F = 8.3 Hz), 132.98 (s), 131.19 (s), 127.32 (s), 122.63 (s), 115.17 (d, 3JC–F = 11.4 Hz), 113.36 (d, 4JC–F = 19.4 Hz), 95.41 (s), 85.75 (s), 30.86 (s). LC/MS (ESI): 543.0 [M − CO2 + H]+.
tert-Butyl(7-iodo-5-(3-oxo-6-(trifluoromethyl)benzo[d][1,2]selenazol-2(3H)-yl)quinolin-8-yl)carbonate (9j). Pale yellow solid, 78% yield. Rf = 0.48 (petroleum/EtOAc = 5/1). 1H NMR (400 MHz, CDCl3) δ 8.92 (dd, J = 4.1, 1.6 Hz, 1H), 8.26 (d, J = 8.2 Hz, 1H), 8.05 (s, 1H), 8.02 (s, 1H), 7.96 (dd, J = 8.5, 1.7 Hz, 1H), 7.77 (d, J = 8.1 Hz, 1H), 7.42 (dd, J = 8.5, 4.1 Hz, 1H), 1.65 (s, 9H). 13C NMR (101 MHz, CDCl3) δ 165.94 (s), 157.01 (s), 149.97 (s), 144.46 (s), 139.71 (s), 137.50 (s), 134.73 (q, 1JC–F = 32.9 Hz), 131.86 (s), 130.06 (s), 129.30 (s), 128.55 (s), 126.97 (s), 126.14 (dd, 2JC–F = 192.1, 81.2 Hz), 123.72 (d, 3JC–F = 3.3 Hz), 122.09 (s), 121.81 (dd, 4JC–F = 7.4, 3.5 Hz), 94.12 (s), 87.00 (s), 30.64 (s). LC/MS (ESI): 592.9 [M − CO2 + H]+.
tert-Butyl(7-iodo-5-(6-methoxy-3-oxobenzo[d][1,2]selenazol-2(3H)-yl)quinolin-8-yl)carbonate (9n). White solid, 81% yield. Rf = 0.37 (petroleum/EtOAc = 5/1). 1H NMR (400 MHz, CDCl3) δ 8.90 (dd, J = 4.0, 1.5 Hz, 1H), 8.10–7.98 (m, 3H), 7.41 (dd, J = 8.5, 4.1 Hz, 1H), 7.16 (d, J = 1.7 Hz, 1H), 7.07 (dd, J = 8.3, 1.7 Hz, 1H), 3.95 (s, 3H), 1.66 (s, 9H). 13C NMR (101 MHz, CDCl3) δ 166.93, 163.69, 156.49, 149.83, 144.47, 141.14, 137.53, 132.29, 130.60, 130.20, 127.32, 121.91, 118.62, 114.96, 107.68, 94.27, 86.73, 55.89, 30.66. LC/MS (ESI): 592.9 [M − CO2 + H]+.
tert-Butyl(7-iodo-5-(5-methoxy-3-oxobenzo[d][1,2]selenazol-2(3H)-yl)quinolin-8-yl)carbonate (9o). White solid, 72% yield. Rf = 0.38 (petroleum/EtOAc = 5/1). 1H NMR (400 MHz, CDCl3) δ 8.91 (dd, J = 4.0, 1.5 Hz, 1H), 8.02 (s, 1H), 8.00 (dd, J = 8.5, 1.6 Hz, 1H), 7.63 (d, J = 2.5 Hz, 1H), 7.58 (d, J = 8.7 Hz, 1H), 7.41 (dd, J = 8.5, 4.1 Hz, 1H), 7.36 (dd, J = 8.7, 2.6 Hz, 1H), 3.93 (s, 3H), 1.66 (s, 9H). 13C NMR (101 MHz, CDCl3) δ 167.10, 159.26, 156.53, 149.85, 144.43, 137.34, 132.23, 130.29, 130.18, 127.12, 126.69, 125.10, 122.70, 121.92, 111.16, 94.27, 86.72, 55.82, 30.66. LC/MS (ESI): 592.9 [M − CO2 + H]+.
tert-Butyl(7-iodo-5-(3-oxonaphtho[2,3-d][1,2]selenazol-2(3H)-yl)quinolin-8-yl)carbonate (9q). Pale yellow solid, 68% yield. Rf = 0.40 (petroleum/EtOAc = 5/1). 1H NMR (400 MHz, CDCl3) δ 8.93 (dd, J = 4.1, 1.7 Hz, 1H), 8.76 (s, 1H), 8.15–8.06 (m, 4H), 7.94 (d, J = 8.3 Hz, 1H), 7.73–7.68 (m, 1H), 7.64–7.59 (m, 1H), 7.43 (dd, J = 8.5, 4.1 Hz, 1H), 1.69 (s, 9H). 13C NMR (101 MHz, CDCl3) δ 167.03, 156.60, 149.90, 144.52, 137.47, 135.93, 132.43, 132.23, 131.64, 130.39, 130.16, 129.75, 128.90, 127.18, 127.15, 126.57, 124.34, 122.74, 121.96, 94.34, 86.81, 30.66. LC/MS (ESI): 575.0 [M − CO2 + H]+.
General procedure for the synthesis of 10a–10q and 11a–11q
To a solution of 300 mg of 8 (X = Cl), or 9 (X = I) in 12 mL CH2Cl2, HCl gas was bubbled at room temperature for 4 h. The reaction was stirred under an HCl atmosphere overnight at 0 °C. The mixture was filtered and dried under reduced pressure to yield the product.
2-(7-Chloro-8-hydroxyquinolin-5-yl)benzo[d][1,2]selenazol-3(2H)-one hydrochloride (10a). Yellow solid, 89% yield. Mp 246.7–247.6 °C. Rf = 0.25 (CH2Cl2/CH3OH = 10/1). 1H NMR (400 MHz, DMSO-d6) δ 9.03 (d, J = 3.3 Hz, 1H), 8.50 (d, J = 8.0 Hz, 1H), 8.31 (d, J = 8.0 Hz, 1H), 7.89 (d, J = 7.2 Hz, 1H), 7.80 (dd, J = 8.5, 4.6 Hz, 1H), 7.72 (s, 1H), 7.65 (t, J = 7.1 Hz, 1H), 7.47 (t, J = 7.2 Hz, 1H). 13C NMR (101 MHz, DMSO-d6) δ 166.52, 147.79, 146.76, 141.60, 137.36, 135.13, 131.54, 129.27, 128.01, 127.84, 127.59, 127.07, 125.84, 125.58, 122.47, 117.96. [M + H]+ for C16H9ClN2O2Se pred. 376.9591, meas. 376.9591; HPLC purity: 98.31%.
6-Chloro-2-(7-chloro-8-hydroxyquinolin-5-yl)benzo[d][1,2]selenazol-3(2H)-one hydrochloride (10b). Yellow solid, 91% yield. Mp 258.2–259.1 °C. Rf = 0.35 (CH2Cl2/CH3OH = 10/1). 1H NMR (400 MHz, DMSO-d6) δ 9.02 (d, J = 3.4 Hz, 1H), 8.64 (d, J = 1.4 Hz, 1H), 8.27 (d, J = 8.0 Hz, 1H), 7.86 (d, J = 8.2 Hz, 1H), 7.77 (dd, J = 8.5, 4.6 Hz, 1H), 7.69 (s, 1H), 7.50 (dd, J = 8.2, 1.5 Hz, 1H). 13C NMR (101 MHz, DMSO-d6) δ 165.57, 146.84, 145.30, 143.70, 139.87, 136.45, 133.21, 129.56, 128.91, 128.49, 127.65, 126.79, 126.02, 125.77, 122.48, 119.66. [M + H]+ for C16H8Cl2N2O2Se pred. 410.9201, meas. 410.9201; HPLC purity: 99.80%.
5-Chloro-2-(7-chloro-8-hydroxyquinolin-5-yl)benzo[d][1,2]selenazol-3(2H)-one hydrochloride (10c). Yellow solid, 96% yield. Mp 219.7–221.6 °C. Rf = 0.36 (CH2Cl2/CH3OH = 10/1). 1H NMR (400 MHz, DMSO-d6) δ 8.99 (dd, J = 4.4, 1.3 Hz, 1H), 8.51 (d, J = 8.7 Hz, 1H), 8.16 (dd, J = 8.5, 1.1 Hz, 1H), 7.83 (d, J = 2.2 Hz, 1H), 7.72–7.71 (m, 1H), 7.70–7.68 (m, 1H), 7.66 (s, 1H). 13C NMR (101 MHz, DMSO-d6) δ 165.14, 148.54, 147.88, 140.21, 136.67, 135.34, 131.26, 130.96, 129.89, 128.98, 128.77, 127.11, 126.66, 125.22, 122.43, 116.57. [M + H]+ for C16H8Cl2N2O2Se pred. 410.9201, meas. 410.9204; HPLC purity: 96.60%.
4-Chloro-2-(7-chloro-8-hydroxyquinolin-5-yl)benzo[d][1,2]selenazol-3(2H)-one hydrochloride (10d). Yellow solid, 92% yield. Mp 244.5–245.7 °C. Rf = 0.35 (CH2Cl2/CH3OH = 10/1). 1H NMR (400 MHz, DMSO-d6) δ 9.05 (dd, J = 4.5, 1.5 Hz, 1H), 8.56 (dd, J = 8.1, 0.7 Hz, 1H), 8.28 (dd, J = 8.6, 1.4 Hz, 1H), 7.80 (dd, J = 8.6, 4.6 Hz, 1H), 7.75 (s, 1H), 7.60 (t, J = 7.9 Hz, 1H), 7.51 (dd, J = 7.8, 1.0 Hz, 1H). 13C NMR (101 MHz, DMSO-d6) δ 164.92, 148.67, 147.96, 145.27, 136.90, 136.33, 134.82, 131.85, 130.06, 128.57, 127.71, 126.55, 126.15, 123.08, 117.82. [M + H]+ for C16H8Cl2N2O2Se pred. 410.9201, meas. 410.9204; HPLC purity: 98.34%.
2-(7-Chloro-8-hydroxyquinolin-5-yl)-7-fluorobenzo[d][1,2]selenazol-3(2H)-one hydrochloride (10e). Yellow solid, 91% yield. Mp 281.3–281.9 °C. Rf = 0.31 (CH2Cl2/CH3OH = 10/1). 1H NMR (400 MHz, DMSO-d6) δ 9.06 (d, J = 4.3 Hz, 1H), 8.27 (dd, J = 8.5, 1.1 Hz, 1H), 7.91 (s, 1H), 7.86 (d, J = 7.4 Hz, 1H), 7.78 (dd, J = 8.5, 4.4 Hz, 1H), 7.72 (t, J = 8.5 Hz, 1H), 7.65 (td, J = 7.8, 5.1 Hz, 1H). 13C NMR (101 MHz, DMSO-d6) δ 166.36 (d, 1JC–F = 1.9 Hz), 157.94 (d, 2JC–F = 246.3 Hz), 149.55 (s), 149.21 (s), 136.90 (s), 135.50 (s), 131.00 (s), 129.48 (d, 3JC–F = 6.5 Hz), 129.07 (d, 4JC–F = 4.6 Hz), 126.16 (s), 126.12 (d, 5JC–F = 24.6 Hz), 125.72 (s), 125.13 (d, 6JC–F = 2.6 Hz), 123.47 (s), 119.35 (d, 7JC–F = 19.7 Hz), 117.14 (s). [M + H]+ for C16H8ClFN2O2Se pred. 394.9496, meas. 394.9504; HPLC purity: 98.81%.
2-(7-Chloro-8-hydroxyquinolin-5-yl)-6-fluorobenzo[d][1,2]selenazol-3(2H)-one hydrochloride (10f). Yellow solid, 92% yield. Mp 217.6–218.6 °C. Rf = 0.32 (CH2Cl2/CH3OH = 10/1). 1H NMR (400 MHz, DMSO-d6) δ 8.98 (d, J = 3.1 Hz, 1H), 8.26 (dd, J = 9.6, 2.2 Hz, 1H), 8.13 (d, J = 7.8 Hz, 1H), 7.91 (dd, J = 8.4, 5.6 Hz, 1H), 7.69 (dd, J = 8.6, 4.4 Hz, 1H), 7.65 (s, 1H), 7.32 (td, J = 8.6, 2.4 Hz, 1H). 13C NMR (101 MHz, DMSO-d6) δ 165.56 (s), 164.02 (d, 1JC–F = 248.8 Hz), 147.10 (s), 145.67 (s), 144.30 (d, 2JC–F = 10.7 Hz), 139.16 (s), 133.77 (s), 129.64 (s), 129.51 (d, 3JC–F = 6.9 Hz), 128.40 (s), 125.75 (s), 125.27 (s), 122.47 (s), 119.16 (s), 113.92 (d, 4JC–F = 7.2 Hz), 113.67 (d, 5JC–F = 3.1 Hz). [M + H]+ for C16H8ClFN2O2Se pred. 394.9496, meas. 394.9498; HPLC purity: 98.43%.
2-(7-Chloro-8-hydroxyquinolin-5-yl)-5-fluorobenzo[d][1,2]selenazol-3(2H)-one hydrochloride (10g). Yellow solid, 90% yield. Mp 250.9–252.0 °C. Rf = 0.31 (CH2Cl2/CH3OH = 10/1). 1H NMR (400 MHz, DMSO-d6) δ 9.01 (d, J = 3.5 Hz, 1H), 8.52 (dd, J = 8.7, 5.0 Hz, 1H), 8.21 (d, J = 8.4 Hz, 1H), 7.74 (dd, J = 8.4, 4.4 Hz, 1H), 7.68 (s, 1H), 7.62 (dd, J = 8.4, 2.4 Hz, 1H), 7.56 (td, J = 8.8, 2.5 Hz, 1H). 13C NMR (101 MHz, DMSO-d6) δ 165.44 (d, 1JC–F = 2.6 Hz), 162.27 (d, 2JC–F = 36.3 Hz), 159.86 (d, 3JC–F = 36.9 Hz), 147.26 (s), 145.99 (s), 138.75 (s), 136.74 (s), 134.05 (s), 129.94 (d, 4JC–F = 7.4 Hz), 129.33 (s), 128.21 (s), 125.58 (s), 122.47 (s), 119.21 (d, 5JC–F = 23.0 Hz), 118.88 (s), 113.05 (d, 6JC–F = 22.7 Hz). [M + H]+ for C16H8ClFN2O2Se pred. 394.9496, meas. 394.9495; HPLC purity: 98.21%.
2-(7-Chloro-8-hydroxyquinolin-5-yl)-4-fluorobenzo[d][1,2]selenazol-3(2H)-one hydrochloride (10h). Yellow solid, 87% yield. Mp 256.9–257.4 °C. Rf = 0.34 (CH2Cl2/CH3OH = 10/1). 1H NMR (400 MHz, DMSO-d6) δ 9.13 (dd, J = 5.2, 1.2 Hz, 1H), 8.75 (dd, J = 8.6, 1.0 Hz, 1H), 8.41 (d, J = 8.1 Hz, 1H), 8.04 (dd, J = 8.6, 5.2 Hz, 1H), 7.76 (d, J = 8.3 Hz, 1H), 7.63 (td, J = 8.1, 5.0 Hz, 1H), 7.56 (d, J = 8.3 Hz, 1H), 7.24 (dd, J = 10.6, 8.2 Hz, 1H). 13C NMR (101 MHz, DMSO-d6) δ 163.72 (d, 1JC–F = 3.1 Hz), 161.84 (d, 2JC–F = 259.8 Hz), 147.58 (s), 144.53 (s), 144.21 (s), 143.13 (s), 131.88 (d, 3JC–F = 8.3 Hz), 130.58 (s), 128.94 (s), 127.21 (d, 4JC–F = 8.7 Hz), 123.41 (d, 5JC–F = 3.3 Hz), 122.58 (s), 116.13 (d, 6JC–F = 10.8 Hz), 115.59 (s), 112.47 (d, 7JC–F = 19.8 Hz). [M + H]+ for C16H8ClFN2O2Se pred. 394.9496, meas. 394.9498; HPLC purity: 97.39%.
2-(7-Chloro-8-hydroxyquinolin-5-yl)-7-(trifluoromethyl)benzo[d][1,2]selenazol-3(2H)-one hydrochloride (10i). Yellow solid, 76% yield. Mp 298.5–299.0 °C. Rf = 0.38 (CH2Cl2/CH3OH = 10/1). 1H NMR (400 MHz, DMSO-d6) δ 9.00 (d, J = 3.8 Hz, 1H), 8.29 (d, J = 7.6 Hz, 2H), 8.16 (d, J = 8.4 Hz, 1H), 7.90 (s, 1H), 7.85 (t, J = 7.7 Hz, 1H), 7.67 (dd, J = 8.4, 4.2 Hz, 1H). 13C NMR (101 MHz, DMSO-d6) δ 165.81 (s), 164.87 (s), 146.55 (s), 144.15 (s), 136.02 (s), 133.44 (d, 1JC–F = 32.8 Hz), 131.69 (s), 131.19 (s), 130.84 (s), 128.35 (s), 128.18 (s), 127.15 (s), 124.81 (dd, 2JC–F = 41.1, 22.7 Hz), 124.50 (dd, 3JC–F = 271.8, 36.9 Hz), 121.77 (s), 115.09 (s), 110.59 (s). [M + H]+ for C17H8ClF3N2O2Se pred. 444.9464, meas. 444.9475; HPLC purity: 98.02%.
2-(7-Chloro-8-hydroxyquinolin-5-yl)-6-(trifluoromethyl)benzo[d][1,2]selenazol-3(2H)-one hydrochloride (10j). Yellow solid, 88% yield. Mp 211.4–212.3 °C. Rf = 0.40 (CH2Cl2/CH3OH = 10/1). 1H NMR (400 MHz, DMSO-d6) δ 9.02 (s, 2H), 8.28 (d, J = 7.8 Hz, 1H), 8.06 (d, J = 8.0 Hz, 1H), 7.82–7.73 (m, 2H), 7.71 (s, 1H). 13C NMR (101 MHz, DMSO-d6) δ 165.36 (s), 146.70 (s), 145.20 (s), 142.93 (s), 140.26 (s), 132.90 (s), 132.16 (s), 131.13 (q, 1JC–F = 31.5 Hz), 129.64 (s), 128.42 (s), 128.36 (s), 125.75 (s), 124.68 (d, 2JC–F = 4.0 Hz), 124.08 (q, 3JC–F = 273.0 Hz), 122.52 (s), 122.23 (d, 4JC–F = 3.0 Hz), 119.91 (s). [M + H]+ for C17H8ClF3N2O2Se pred. 444.9464, meas. 444.9463; HPLC purity: 99.12%.
2-(7-Chloro-8-hydroxyquinolin-5-yl)-5-(trifluoromethyl)benzo[d][1,2]selenazol-3(2H)-one hydrochloride (10k). Yellow solid, 90% yield. Mp 228.1–228.9 °C. Rf = 0.36 (CH2Cl2/CH3OH = 10/1). 1H NMR (400 MHz, DMSO-d6) δ 9.07 (dd, J = 4.5, 1.1 Hz, 1H), 8.86 (d, J = 8.5 Hz, 1H), 8.38 (d, J = 8.5 Hz, 1H), 8.12 (s, 1H), 8.01 (dd, J = 8.6, 1.7 Hz, 1H), 7.82 (dd, J = 8.6, 4.6 Hz, 1H), 7.77 (s, 1H). 13C NMR (101 MHz, DMSO-d6) δ 165.89 (s), 147.73 (s), 147.12 (s), 145.57 (s), 141.13 (s), 133.16 (s), 130.18 (s), 129.89 (s), 129.31 (s), 128.94 (s), 127.63 (d, 1JC–F = 2.8 Hz), 127.11 (d, 2JC–F = 32.3 Hz), 126.28 (s), 124.76 (dd, 3JC–F = 272.6, 27.8 Hz), 124.36 (d, 4JC–F = 3.6 Hz), 123.07 (s), 120.62 (s). [M + H]+ for C17H8ClF3N2O2Se pred. 444.9464, meas. 444.9471; HPLC purity: 97.98%.
2-(7-Chloro-8-hydroxyquinolin-5-yl)-4-(trifluoromethyl)benzo[d][1,2]selenazol-3(2H)-one hydrochloride (10l). Yellow solid, 75% yield. Mp 229.1–230.5 °C. Rf = 0.50 (CH2Cl2/CH3OH = 10/1). 1H NMR (400 MHz, DMSO-d6) δ 8.97 (dd, J = 4.2, 1.3 Hz, 1H), 8.50 (d, J = 8.6 Hz, 1H), 8.27 (d, J = 7.9 Hz, 1H), 7.82 (d, J = 8.2 Hz, 1H), 7.70–7.65 (m, 3H). 13C NMR (101 MHz, DMSO-d6) δ 164.38 (s), 149.75 (d, 1JC–F = 2.4 Hz), 144.31 (s), 132.89 (s), 131.70 (s), 131.10 (s), 129.96 (s), 129.50 (dd, 2JC–F = 72.1, 39.4 Hz), 125.93 (s), 125.37 (d, 3JC–F = 6.1 Hz), 123.65 (q, 4JC–F = 273.7 Hz), 123.59 (s), 122.93 (s), 115.17 (s). [M + H]+ for C17H8ClF3N2O2Se pred. 444.9464, meas. 444.9480; HPLC purity: 98.01%.
2-(7-Chloro-8-hydroxyquinolin-5-yl)-7-methoxybenzo[d][1,2]selenazol-3(2H)-one hydrochloride (10m). Yellow solid, 77% yield. Mp 253.7–254.9 °C. Rf = 0.36 (CH2Cl2/CH3OH = 10/1). 1H NMR (400 MHz, DMSO-d6) δ 8.86 (d, J = 2.6 Hz, 1H), 7.88 (d, J = 8.4 Hz, 1H), 7.70 (s, 1H), 7.59 (d, J = 4.3 Hz, 2H), 7.55 (dd, J = 8.6, 4.1 Hz, 1H), 7.45–7.39 (m, 1H), 4.00 (s, 3H). 13C NMR (101 MHz, DMSO-d6) δ 167.26, 154.58, 149.10, 140.37, 132.39, 130.48, 129.30, 128.33, 127.07, 126.19, 122.88, 120.89, 115.20, 113.95, 56.80. [M + H]+ for C17H11ClN2O3Se pred. 406.9696, meas. 406.9699; HPLC purity: 98.32%.
2-(7-Chloro-8-hydroxyquinolin-5-yl)-6-methoxybenzo[d][1,2]selenazol-3(2H)-one hydrochloride (10n). Yellow solid, 91% yield. Mp 254.2–245.5 °C. Rf = 0.34 (CH2Cl2/CH3OH = 10/1). 1H NMR (400 MHz, DMSO-d6) δ 9.01 (dd, J = 4.4, 1.5 Hz, 1H), 8.15 (dd, J = 8.5, 1.3 Hz, 1H), 7.96 (d, J = 2.3 Hz, 1H), 7.81 (d, J = 8.6 Hz, 1H), 7.73 (dd, J = 8.6, 4.4 Hz, 1H), 7.70 (s, 1H), 7.08 (dd, J = 8.6, 2.4 Hz, 1H), 3.88 (s, 3H). 13C NMR (101 MHz, DMSO-d6) δ 166.24, 162.08, 147.49, 146.18, 143.73, 138.12, 134.56, 129.38, 128.71, 128.29, 125.74, 122.42, 121.10, 118.44, 113.72, 110.82, 55.55. [M + H]+ for C17H11ClN2O3Se pred. 406.9696, meas. 406.9696; HPLC purity: 96.52%.
2-(7-Chloro-8-hydroxyquinolin-5-yl)-5-methoxybenzo[d][1,2]selenazol-3(2H)-one hydrochloride (10o). Yellow solid, 87% yield. Mp 221.3–222.5 °C. Rf = 0.36 (CH2Cl2/CH3OH = 10/1). 1H NMR (400 MHz, DMSO-d6) δ 9.00 (d, J = 3.2 Hz, 1H), 8.30 (d, J = 8.8 Hz, 1H), 8.16 (d, J = 7.8 Hz, 1H), 7.73 (dd, J = 8.5, 4.4 Hz, 1H), 7.67 (s, 1H), 7.39 (d, J = 2.5 Hz, 1H), 7.30 (dd, J = 8.8, 2.7 Hz, 1H), 3.84 (s, 3H). 13C NMR (101 MHz, DMSO-d6) δ 166.21, 158.26, 146.91, 145.34, 139.70, 133.31, 132.71, 129.46, 129.07, 128.80, 128.31, 125.71, 122.45, 120.19, 119.55, 109.96, 55.45. [M + H]+ for C17H11ClN2O3Se pred. 406.9696, meas. 406.9694; HPLC purity: 98.45%.
2-(7-Chloro-8-hydroxyquinolin-5-yl)-4-methoxybenzo[d][1,2]selenazol-3(2H)-one hydrochloride (10p). Yellow solid, 76% yield. Mp 263.4–264.2 °C. Rf = 0.31 (CH2Cl2/CH3OH = 5/1). 1H NMR (400 MHz, DMSO-d6) δ 8.95 (s, 1H), 7.94 (d, J = 8.2 Hz, 1H), 7.71 (d, J = 7.7 Hz, 1H), 7.61–7.57 (m, 3H), 7.04 (d, J = 7.8 Hz, 1H), 3.85 (s, 3H). 13C NMR (101 MHz, DMSO-d6) δ 165.76, 161.26, 150.25, 149.98, 143.34, 139.20, 133.28, 133.15, 129.69, 126.48, 125.88, 122.97, 118.16, 115.36, 114.52, 108.99, 56.06. [M + H]+ for C17H11ClN2O3Se pred. 406.9696, meas. 406.9706; HPLC purity: 99.01%.
2-(7-Chloro-8-hydroxyquinolin-5-yl)naphtho[2,3-d][1,2]selenazol-3(2H)-one hydrochloride (10q). Yellow solid, 81% yield. Mp 229.9–231.1 °C. Rf = 0.42 (CH2Cl2/CH3OH = 10/1). 1H NMR (400 MHz, DMSO-d6) δ 9.04 (d, J = 3.3 Hz, 1H), 8.93 (s, 1H), 8.56 (s, 1H), 8.35 (d, J = 8.2 Hz, 1H), 8.16 (d, J = 8.1 Hz, 1H), 7.98 (d, J = 8.2 Hz, 1H), 7.79 (dd, J = 8.6, 4.6 Hz, 1H), 7.76 (s, 1H), 7.67–7.62 (m, 1H), 7.59–7.53 (m, 1H). 13C NMR (101 MHz, DMSO-d6) δ 166.25, 147.35, 145.92, 138.61, 135.92, 134.85, 134.27, 130.94, 129.28, 129.05, 128.62, 127.98, 127.82, 127.19, 125.70, 125.62, 125.45, 122.41, 118.84. [M + H]+ for C20H11ClN2O2Se pred. 426.9747, meas. 426.9753; HPLC purity: 99.81%.
2-(8-Hydroxy-7-iodoquinolin-5-yl)benzo[d][1,2]selenazol-3(2H)-one hydrochloride (11a). Yellow solid, 85% yield. Mp 218.5–219.9 °C. Rf = 0.33 (CH2Cl2/CH3OH = 10/1). 1H NMR (400 MHz, DMSO-d6) δ 8.94 (dd, J = 4.3, 1.4 Hz, 1H), 8.43 (d, J = 8.0 Hz, 1H), 8.09 (d, J = 8.5 Hz, 1H), 7.88 (d, J = 7.7 Hz, 1H), 7.85 (s, 1H), 7.69 (dd, J = 8.6, 4.4 Hz, 1H), 7.67–7.63 (m, 1H), 7.47 (t, J = 7.4 Hz, 1H). 13C NMR (101 MHz, DMSO-d6) δ 166.45, 152.29, 148.31, 141.39, 136.35, 135.40, 135.22, 135.20, 131.58, 127.85, 127.62, 126.90, 126.17, 125.87, 122.60, 80.92. [M + H]+ for C16H9IN2O2Se pred. 468.8947, meas. 468.8950; HPLC purity: 96.73%.
6-Chloro-2-(8-hydroxy-7-iodoquinolin-5-yl)benzo[d][1,2]selenazol-3(2H)-one hydrochloride (11b). Red solid, 92% yield. Mp 218.2–219.1 °C. Rf = 0.41 (CH2Cl2/CH3OH = 10/1). 1H NMR (400 MHz, DMSO-d6) δ 8.92 (d, J = 2.9 Hz, 1H), 8.52 (s, 1H), 8.03 (d, J = 8.4 Hz, 1H), 7.86 (d, J = 8.2 Hz, 1H), 7.82 (s, 1H), 7.66 (dd, J = 8.4, 4.3 Hz, 1H), 7.52 (dd, J = 8.2, 1.6 Hz, 1H). 13C NMR (101 MHz, DMSO-d6) δ 165.47, 151.49, 147.80, 143.45, 136.46, 134.47, 128.94, 128.43, 128.19, 128.04, 127.46, 126.62, 126.22, 126.07, 122.56, 82.41. [M + H]+ for C16H8ClIN2O2Se pred. 502.8557, meas. 502.8556; HPLC purity: 97.68%.
5-Chloro-2-(8-hydroxy-7-iodoquinolin-5-yl)benzo[d][1,2]selenazol-3(2H)-one hydrochloride (11c). Red solid, 84% yield. Mp 228.4–229.6 °C. Rf = 0.43 (CH2Cl2/CH3OH = 10/1). 1H NMR (400 MHz, DMSO-d6) δ 8.96 (s, 1H), 8.58 (d, J = 7.3 Hz, 1H), 8.20 (d, J = 7.9 Hz, 1H), 7.87 (s, 1H), 7.81 (s, 1H), 7.76–7.71 (m, 1H), 7.68 (d, J = 8.5 Hz, 1H). 13C NMR (101 MHz, DMSO-d6) δ 165.06, 151.66, 147.92, 140.39, 136.30, 134.67, 131.09, 130.87, 130.13, 129.19, 128.16, 126.56, 126.11, 122.56, 82.03. [M + H]+ for C16H8ClIN2O2Se pred. 502.8557, meas. 502.8556; HPLC purity: 98.12%.
7-Fluoro-2-(8-hydroxy-7-iodoquinolin-5-yl)benzo[d][1,2]selenazol-3(2H)-one hydrochloride (11e). Yellow solid, 81% yield. Mp 205.2–206.3 °C. Rf = 0.32 (CH2Cl2/CH3OH = 10/1). 1H NMR (400 MHz, DMSO-d6) δ 8.99 (dd, J = 4.2, 1.3 Hz, 1H), 8.13 (dd, J = 8.5, 1.3 Hz, 1H), 8.05 (s, 1H), 7.86 (d, J = 7.5 Hz, 1H), 7.77–7.69 (m, 2H), 7.65 (td, J = 7.8, 5.0 Hz, 1H). 13C NMR (101 MHz, DMSO-d6) δ 166.30 (d, 1JC–F = 2.0 Hz), 157.92 (d, 2JC–F = 246.0 Hz), 154.53 (s), 149.45 (s), 138.00 (s), 136.52 (s), 134.14 (s), 129.48 (d, 3JC–F = 6.5 Hz), 129.09 (d, 4JC–F = 4.7 Hz), 126.76 (s), 126.11 (s), 126.02 (d, 5JC–F = 24.4 Hz), 125.14 (s), 123.60 (s), 119.29 (d, 6JC–F = 19.8 Hz), 80.21 (s). [M + H]+ for C16H8FIN2O2Se pred. 486.8852, meas. 486.8857; HPLC purity: 99.21%.
6-Fluoro-2-(8-hydroxy-7-iodoquinolin-5-yl)benzo[d][1,2]selenazol-3(2H)-one hydrochloride (11f). Yellow solid, 89% yield. Mp 209.5–210.3 °C. Rf = 0.32 (CH2Cl2/CH3OH = 10/1). 1H NMR (400 MHz, DMSO-d6) δ 8.93 (d, J = 3.0 Hz, 1H), 8.30 (dd, J = 9.7, 2.1 Hz, 1H), 8.08 (d, J = 7.7 Hz, 1H), 7.90 (dd, J = 8.4, 5.6 Hz, 1H), 7.83 (s, 1H), 7.68 (dd, J = 8.4, 4.3 Hz, 1H), 7.31 (td, J = 8.6, 2.3 Hz, 1H). 13C NMR (101 MHz, DMSO-d6) δ 165.47 (s), 164.06 (d, 1JC–F = 249.0 Hz), 152.08 (s), 148.20 (s), 143.90 (d, 2JC–F = 10.9 Hz), 136.32 (s), 135.35 (d, 3JC–F = 22.8 Hz), 129.65 (d, 4JC–F = 9.7 Hz), 127.95 (s), 126.18 (s), 124.98 (s), 122.57 (s), 113.99 (s), 113.72 (d, 5JC–F = 6.6 Hz), 113.41 (s), 81.15 (s). [M + H]+ for C16H8FIN2O2Se pred. 486.8852, meas. 486.8854; HPLC purity: 98.41%.
5-Fluoro-2-(8-hydroxy-7-iodoquinolin-5-yl)benzo[d][1,2]selenazol-3(2H)-one hydrochloride (11g). Yellow solid, 92% yield. Mp 236.7–237.9 °C. Rf = 0.38 (CH2Cl2/CH3OH = 10/1). 1H NMR (400 MHz, DMSO-d6) δ 8.93 (dd, J = 4.2, 1.4 Hz, 1H), 8.46 (dd, J = 8.7, 5.0 Hz, 1H), 8.05 (d, J = 8.5 Hz, 1H), 7.84 (s, 1H), 7.67 (dd, J = 8.5, 4.3 Hz, 1H), 7.62 (dd, J = 8.5, 2.5 Hz, 1H), 7.57 (td, J = 8.9, 2.6 Hz, 1H). 13C NMR (101 MHz, DMSO-d6) δ 165.33 (d, 1JC–F = 3.2 Hz), 162.44 (s), 160.03 (s), 150.83 (s), 147.35 (s), 137.73 (s), 136.68 (s), 133.56 (s), 129.96 (d, 2JC–F = 7.4 Hz), 129.34 (d, 3JC–F = 7.3 Hz), 128.65 (s), 126.27 (s), 122.56 (s), 119.17 (d, 4JC–F = 22.7 Hz), 113.02 (d, 5JC–F = 22.8 Hz), 83.82 (s). [M + H]+ for C16H8FIN2O2Se pred. 486.8852, meas. 486.8851; HPLC purity: 98.24%.
4-Fluoro-2-(8-hydroxy-7-iodoquinolin-5-yl)benzo[d][1,2]selenazol-3(2H)-one hydrochloride (11h). Yellow solid, 94% yield. Mp 218.0–219.1 °C. Rf = 0.40 (CH2Cl2/CH3OH = 10/1). 1H NMR (400 MHz, DMSO-d6) δ 8.94 (dd, J = 4.1, 1.3 Hz, 1H), 8.20 (d, J = 8.1 Hz, 1H), 8.07 (dd, J = 8.5, 1.3 Hz, 1H), 7.86 (s, 1H), 7.68 (dd, J = 8.6, 4.3 Hz, 1H), 7.66–7.61 (m, 1H), 7.24 (dd, J = 10.4, 8.1 Hz, 1H). 13C NMR (101 MHz, DMSO-d6) δ 163.70 (d, 1JC–F = 3.1 Hz), 161.83 (d, 2JC–F = 259.8 Hz), 151.27 (s), 147.60 (s), 144.06 (s), 137.07 (s), 136.97 (s), 134.06 (d, 3JC–F = 2.7 Hz), 131.96 (d, 4JC–F = 8.2 Hz), 128.08 (s), 126.44 (s), 123.27 (d, 5JC–F = 3.1 Hz), 122.62 (s), 115.97 (d, 6JC–F = 11.4 Hz), 112.47 (d, 7JC–F = 19.6 Hz), 83.02 (s). [M + H]+ for C16H8FIN2O2Se pred. 486.8852, meas. 486.8849; HPLC purity: 98.39%.
2-(8-Hydroxy-7-iodoquinolin-5-yl)-6-(trifluoromethyl)benzo[d][1,2]selenazol-3(2H)-one hydrochloride (11j). Yellow solid, 91% yield. Mp 201.2–202.3 °C. Rf = 0.44 (CH2Cl2/CH3OH = 10/1). 1H NMR (400 MHz, DMSO-d6) δ 8.94 (s, 2H), 8.08 (t, J = 8.4 Hz, 2H), 7.86 (s, 1H), 7.79 (d, J = 7.7 Hz, 1H), 7.68 (dd, J = 8.2, 4.2 Hz, 1H). 13C NMR (101 MHz, DMSO-d6) δ 165.29 (s), 151.03 (s), 147.41 (s), 142.72 (s), 137.57 (s), 136.73 (s), 133.70 (s), 131.97 (s), 131.17 (q, 1JC–F = 31.3 Hz), 128.41 (s), 128.30 (s), 126.27 (s), 124.54 (d, 2JC–F = 4.0 Hz), 124.07 (q, 3JC–F = 273.0 Hz), 122.60 (s), 122.27 (d, 4JC–F = 3.1 Hz), 83.59 (s). [M + H]+ for C17H8F3IN2O2Se pred. 536.8821, meas. 536.8819; HPLC purity: 97.49%.
2-(8-Hydroxy-7-iodoquinolin-5-yl)-6-methoxybenzo[d][1,2]selenazol-3(2H)-one hydrochloride (11n). Yellow solid, 95% yield. Mp 195.4–196.7 °C. Rf = 0.31 (CH2Cl2/CH3OH = 10/1). 1H NMR (400 MHz, DMSO-d6) δ 8.92 (d, J = 2.9 Hz, 1H), 7.98 (d, J = 8.4 Hz, 1H), 7.86 (d, J = 2.0 Hz, 1H), 7.82 (s, 1H), 7.79 (d, J = 8.5 Hz, 1H), 7.65 (dd, J = 8.4, 4.3 Hz, 1H), 7.06 (dd, J = 8.6, 2.2 Hz, 1H), 3.86 (s, 3H). 13C NMR (101 MHz, DMSO-d6) δ 166.20, 162.12, 151.63, 147.89, 143.52, 136.62, 136.20, 134.66, 128.75, 128.29, 126.36, 122.56, 120.88, 113.82, 110.59, 82.09, 55.57. [M + H]+ for C17H11IN2O3Se pred. 498.9052, meas. 498.9053; HPLC purity: 98.58%.
2-(8-Hydroxy-7-iodoquinolin-5-yl)-5-methoxybenzo[d][1,2]selenazol-3(2H)-one hydrochloride (11o). Yellow solid, 83% yield. Mp 222.9–223.8 °C. Rf = 0.36 (CH2Cl2/CH3OH = 10/1). 1H NMR (400 MHz, DMSO-d6) δ 8.92 (dd, J = 4.1, 1.3 Hz, 1H), 8.20 (d, J = 7.2 Hz, 1H), 7.99 (d, J = 7.8 Hz, 1H), 7.82 (s, 1H), 7.66 (dd, J = 8.5, 4.2 Hz, 1H), 7.39 (d, J = 2.5 Hz, 1H), 7.32 (dd, J = 8.6, 2.4 Hz, 1H), 3.84 (s, 3H). 13C NMR (101 MHz, DMSO-d6) δ 166.25, 158.36, 152.29, 152.27, 148.26, 136.37, 135.26, 132.10, 128.49, 127.91, 127.73, 126.11, 122.62, 120.59, 110.10, 81.02, 55.48. [M + H]+ for C17H11IN2O3Se pred. 498.9054, meas. 498.9053; HPLC purity: 96.38%.
2-(8-Hydroxy-7-iodoquinolin-5-yl)naphtho[2,3-d][1,2]selenazol-3(2H)-one hydrochloride (11q). Yellow solid, 86% yield. Mp 232.2–233.1 °C. Rf = 0.38 (CH2Cl2/CH3OH = 10/1). 1H NMR (400 MHz, DMSO-d6) δ 8.95 (dd, J = 4.3, 1.4 Hz, 1H), 8.84 (s, 1H), 8.58 (s, 1H), 8.18 (d, J = 8.2 Hz, 1H), 8.14 (dd, J = 8.5, 1.0 Hz, 1H), 8.00 (d, J = 8.4 Hz, 1H), 7.91 (s, 1H), 7.69 (dd, J = 8.6, 4.3 Hz, 1H), 7.66 (d, J = 7.4 Hz, 1H), 7.57 (t, J = 7.3 Hz, 1H). 13C NMR (101 MHz, DMSO-d6) δ 166.18, 151.69, 147.98, 136.39, 136.17, 135.66, 134.87, 130.95, 129.09, 128.48, 128.00, 127.86, 127.56, 127.20, 126.18, 125.74, 125.25, 122.54, 82.01. [M + H]+ for C20H11IN2O2Se pred. 518.9103, meas. 518.9100; HPLC purity: 98.76%.
Biological assays
ThT assay40. Aβ1–42 (Millipore, counter ion: NaOH) was dissolved in ammonium hydroxide (1% v/v) to give a stock solution (2000 μM), which was aliquoted into small samples and stored at −80 °C.For the inhibition of self-mediated Aβ1–42 aggregation experiment, the Aβ stock solution was diluted with 50 mM phosphate buffer (pH 7.4) to 50 μM before use. A mixture of the peptide (10 μL, 25 μM, final concentration) with or without the tested compound (10 μL) was incubated at 37 °C for 48 h. Blanks using 50 mM phosphate buffer (pH 7.4) instead of Aβ with or without inhibitors were also carried out. The sample was diluted to a final volume of 200 μL with 50 mM glycine–NaOH buffer (pH 8.0) containing thioflavin T (5 μM). Then the fluorescence intensities were recorded five minutes later (excitation, 450 nm; emission, 485 nm). None of the tested compounds displayed fluorescence signals under the experimental conditions. The percent inhibition of aggregation was calculated by the expression (1 − IFi/IFc) × 100%, in which IFi and IFc are the fluorescence intensities obtained for Aβ in the presence and absence of inhibitors after subtracting the background, respectively.
For the inhibition of copper-mediated Aβ1–42 aggregation experiment, the Aβ stock solution was diluted in 20 μM HEPES (pH 6.6) with 150 μM NaCl. The mixture of the peptide (10 μL, 25 μM, final concentration) with or without copper (10 μL, 25 μM, final concentration) and the tested compound (10 μL, 50 μM, final concentration) was incubated at 37 °C for 24 h. Then 20 μL of the sample was diluted to a final volume of 200 μL with 50 mM glycine–NaOH buffer (pH 8.0) containing thioflavin T (5 μM). The detection method was the same as that of self-mediated Aβ1–42 aggregation experiment.
For the disaggregation of self-induced Aβ fibrils experiment, the Aβ stock solution was diluted with 10 mM phosphate buffer (pH 7.4). The peptide (15 μL, 50 μM) was incubated at 37 °C for 24 h. The tested compound (15 μL, 50 μM) was then added and incubated at 37 °C for another 24 h. Then 20 μL of the sample was diluted to a final volume of 200 μL with 50 mM glycine–NaOH buffer (pH 8.0) containing thioflavin T (5 μM). The detection method was the same as above.
For the disaggregation of copper-induced Aβ fibrils experiment, the Aβ stock solution was diluted in 20 μM HEPES (pH 6.6) with 150 μM NaCl. The mixture of the peptide (10 μL, 25 μM, final concentration) with copper (10 μL, 25 μM, final concentration) was incubated at 37 °C for 24 h. The tested compound (10 μL, 50 μM, final concentration) was then added and incubated at 37 °C for another 24 h.50 Then 20 μL of the sample was diluted to a final volume of 200 μL with 50 mM glycine–NaOH buffer (pH 8.0) containing thioflavin T (5 μM). The detection method was the same as above.
TEM assay45. For the metal-free experiment, Aβ stock solution was diluted with a 10 mM phosphate buffer (pH = 7.4); for the copper-induced experiment, Aβ stock solution was diluted with 20 μM HEPES (pH = 6.6) and 150 μM NaCl. The sample preparation was same as that for the ThT assay.Aliquots (10 μL) of the samples were placed on a carbon-coated copper/rhodium grid for 2 min. Each grid was stained with uranyl acetate (1%, 5 μL) for 2 min. After draining off the excess staining solution, the specimen was transferred for imaging in a transmission electron microscope (JEOL JEM-1400). All compounds are solubilized in the buffer which was used for the experiment.
Oxygen radical absorbance capacity (ORAC-FL) assay46,47 The tested compound and fluorescein (FL) stock solution were diluted with 75 mM phosphate buffer (pH 7.4) to 10 μM(or 20 μM) and 0.117 μM, respectively. The solution of (±)-6-hydroxy-2,5,7,8-tetramethylchroman-2-carboxylic acid (Trolox) was diluted with the same buffer to 100, 80, 60, 50, 40, 20, and 10 μM. The solution of 2,2′-azobis-(amidinopropane)dihydrochloride (AAPH) was prepared before the experiment by dissolving 108.4 mg AAPH in 10 mL 75 mM phosphate buffer (pH 7.4) to a final concentration of 40 mM. The mixture of the tested compound (20 μL) and FL (120 μL; 70 nM, final concentration) was pre-incubated for 10 min at 37 °C, and then 60 μL of the AAPH solution was added. The fluorescence was recorded every minute for 120 min (excitation, 485 nm; emission, 520 nm). A blank using phosphate buffer instead of the tested compound was also carried out. All reaction mixtures were prepared triple and at least three independent runs were performed for each sample. The antioxidant curves (fluorescence versus time) were normalized to the curve of the blank. The area under the fluorescence decay curve (AUC) was calculated as following equation:
where
f0 is the initial fluorescence reading at 0 min and
fi is the fluorescence reading at time
i. The net AUC was calculated by the expression: AUC
sample − AUC
blank. Regression equations between net AUC and Trolox concentrations were calculated. ORAC-FL value for each sample were calculated by using the standard curve which means the ORAC-FL value of tested compound expressed as Trolox equivalents.
Coupled reductase assay
The GPx-like activity of the organoselenium compounds was determined using a spectrophotometric method at 340 nm as described by Wilson et al.48 The test mixture contained GSH (2 mM), EDTA (1 mM), glutathione reductase (1.3 unite per mL) and NADPH (0.4 mM) in 100 mM potassium phosphate buffer, pH 7.5. GPx samples (80 μM) were added to the test mixture at 25 °C, and the reaction was initiated by the addition of peroxide (1.6 mM). The initial reduction rates were calculated from the oxidation rate of NADPH at 340 nm. The initial reduction rate was determined at least 3 times and calculated from the first 5–10% of the reaction using 6.22 mM−1 cm−1 as the extinction coefficient for NADPH.
Computational methods
All calculations were performed using the Gaussian 09 suite of quantum chemical programs. The hybrid Becke 3-Lee–Yang–Parr (B3LYP) exchange correlation–functional was applied for DFT calculations. The computational models were fully optimized using DFT calculations at B3LYP/6-311+g(d) level of theory and characterized by frequency calculation. As glutathione was very flexible, the G group replaced with a Me group according to the reported method in literature.28,30–33 Orbital interactions were analyzed using the natural bond orbital (NBO) method at the B3LYP/6-311+g(d,p) level.
Horseradish peroxidase assay
The hydrogen peroxide scavenging property was measured using the horseradish peroxidase assay.49 Test compounds of 200 μL of different concentrations were incubated with 100 μL of 1 mM H2O2. Then, 0.1 M phosphate buffer (pH 7.4, 100 μL) and 0.1 M NaCl (50 μL) were added. The solution was incubated for 10 h at 37 °C. Then, a solution containing red phenol (0.2 mg mL−1) and horseradish peroxidase (0.5 mg mL−1) in 0.1 M phosphate buffer (500 μL) was added. After 15 min, 1 M NaOH solution (50 μL) was added to quench the reaction, and the absorbance was recorded at 610 nm. The result is expressed as the percentage of reduction of H2O2. The percentage of H2O2 scavenging activity was calculated according to the following formula: 1 − (Asample − Ablank)/(Acontrol − Ablank) × 100, where Asample is the absorbance of the samples, Ablank is the absorbance of the blank, and Acontrol is the absorbance of the control.
Neuronal antioxidative effects against the production of ROS induced by t-BuOOH
The evaluation of neuronal antioxidative effects against the production of ROS induced by t-BuOOH was carried out according to our previous reported method.24 The cytotoxicity was evaluated with the colorimetric MTT [3-(4,5-dimethyl-2-thiazolyl)-2,5-diphenyl-2H-tetrazolium bromide] assay. Human neuron-like cells, SH-SY5Y, were routinely grown at 37 °C in a humidified incubator with 5% CO2 in Dulbecco's modified Eagle's medium (DMEM, GIBCO) supplemented with 10% foetal calf serum (FCS, GIBCO), 1 mM glutamine, 50 mg μL−1 penicillin and 50 mg μL−1 streptomycin. SH-SY5Y cells were seeded at 5 × 104 cells per well in 96-well plates. After 24 h, the medium was removed and replaced with the tested compounds at different concentrations for 24 h at 37 °C. Then, the cells were incubated with MTT (2.5 mg mL−1) in PBS for 4 h. After the removal of MTT, the formazan crystals were dissolved in DMSO. The amount of formazan was measured (570 nm). Cell viability was expressed as percentage of control cells and calculated by the formula Ft/Fnt × 100, where Ft = absorbance of treated neurones and Fnt = absorbance of untreated neurones. SH-SY5Y cells were seeded at 1 × 104 cells per well in 96-well plates; after 24 h, the medium was removed and replaced with tested compounds at 37 °C and kept for another 24 h. The cells were washed with PBS and incubated with 5 μM of DCFH-DA (a fluorescent probe) in PBS at 37 °C in 5% CO2 for 30 min. After removal of DCFH-DA and further washing, the cells were exposed to 0.1 mM t-BuOOH (a compound used to induce oxidative stress) in PBS for 30 min. At the end of the incubation, the fluorescence of the cells from each well was measured (λexcitation = 485 nm, λemission = 535 nm) with a multifunctional microplate reader (Molecular Devices, Flex Station 3). The antioxidant activity was expressed as percentage of control cells and calculated by the formula (Ft − Fnt)/(F′t − Fnt) × 100, where Ft = absorbance of treated neurons with tested compound, F′t = absorbance of treated neurons without tested compound and Fnt = absorbance of neurons not treated with t-BuOOH.
In vitro blood–brain barrier permeation assay
The blood–brain barrier penetration of compounds was evaluated using the parallel artificial membrane permeation assay (PAMPA) described by Di et al.50–52 Commercial drugs were purchased from Sigma and Alfa Aesar. Porcine brain lipid (PBL) was obtained from Avanti Polar Lipids. The donor microplate (PVDF membrane, pore size 0.45 mm) and acceptor microplate were both from Millipore. The 96-well UV plate (COSTAR®) was from Corning Incorporated. The acceptor 96-well microplate was filled with 300 μL PBS/EtOH (7
:
3), and the filter membrane was impregnated with 4 μL PBL in dodecane (20 mg mL−1). Compounds were dissolved in DMSO at 5 mg mL−1 and diluted 50-fold in PBS/EtOH (7
:
3) to a final concentration of 100 μg mL−1. Then, 200 μL of the solution was added to the donor wells. The acceptor filter plate was carefully placed on the donor plate to form a sandwich, which was left undisturbed for 10 h at 25 °C. After incubation, the donor plate was carefully removed, and the concentration of compounds in the acceptor wells was determined using the UV plate reader (Flexstation® 3). Every sample was analyzed at five wavelengths in four wells and in at least three independent runs. Pe was calculated by the following expression: Pe = −Vd × Va/[(Vd + Va)A × t] × ln(1 − drugacceptor/drugequilibrium) where Vd is the volume of donor well; Va, volume in acceptor well; A, filter area; t, permeation time; drugacceptor, the absorbance obtained in the acceptor well; drugequilibrium, the theoretical equilibrium absorbance. The results are given as the mean ± standard deviation. In the experiment, 13 quality control standards (Table S13, see ESI†) of known BBB permeability were included to validate the analysis set. A plot of the experimental data versus literature values gave a strong linear correlation, Pe(exp) = 1.4574Pe(lit) − 1.0773 (R2 = 0.9427) (Fig. S1†). From this equation and the limit established by Di et al. (Pe(lit) = 4.0 × 10−6 cm s−1) for blood–brain barrier permeation, we concluded that compounds with a permeability greater than 4.7 × 10−6 cm s−1 could cross the blood–brain barrier (Table S14†).
Acute toxicity
A total of 20 KM mice (KM mice, which are common closed colony mice and most widely used in biomedical research in china) (male, 22 days, 18–20 g) purchased from the laboratory animal center of Sun Yat-sen University (Guangzhou, China) were used to evaluate the acute toxicity of compound 10h. Mice were maintained with a 12 h light/dark cycle (light from 07
:
00 to 19
:
00) at 20–22 °C with a relative humidity of 60–70%. Sterile food and water were provided according to institutional guidelines. Prior to each experiment, mice were fasted overnight and allowed free access to water. Compound 10h was suspension in 0.5% carboxymethyl cellulose sodium (CMC-Na) salt solution (677, 1333, and 2000 mg kg−1) and given via oral administration according to the divided experimental groups. After the administration of the compounds, the mice were observed continuously for the first 4 h for any abnormal behavior and mortality changes, intermittently for the next 24 h, and occasionally thereafter for 14 days for the onset of any delayed effects. All animals were sacrificed on the 14th day after drug administration and were macroscopically examined for possible damage to the heart, liver, and kidneys.
Metal-chelating study
The chelating studies were performed with a UV-Vis spectrophotometer. All compounds are solubilized in the buffer which was used for the experiment. The absorption spectra of each compound (50 μM, final concentration) alone or in the presence of CuSO4, FeSO4, or ZnCl2 (50 μM, final concentration) for 30 min in buffer (20 mM HEPES, 150 mM NaCl, pH 7.4) were recorded at room temperature.
For the stoichiometry of the compound-Cu2+ complex, a fixed amount of 10h (80 μM) was mixed with growing amounts of copper ion (0–128 μM), and the difference UV-vis spectra were examined to investigate the ratio of ligand/metal in the complex.
Statistical analysis
The results are expressed as the mean ± SD of at least three independent experiments. Data were subjected to Student's t test or one-way analysis of variance (ANOVA) followed by Dunnett's test. p values less than 0.05 were accepted to indicate the significance.
Acknowledgements
We thank the National Natural Science Foundation of China (No. 21302235, 20972198), Guangdong Natural Science Foundation (2014A030313124) and the Fundamental Research Funds for the Central Universities (15ykpy04) for financial support of this study. We also thank the National Supercomputing Center in Guangzhou for providing the computational resources. This research is also supported in part by the Guangdong Province Key Laboratory of Computational Science and the Guangdong Province Computational Science Innovative Research Team.
Notes and references
- M. Prince, E. Albanese, M. Guerchet and M. Prina, World Alzheimer Report, 2014, pp. 1–99 Search PubMed.
- E. scarpini, P. Schelterns and H. Feldman, Lancet Neurol., 2003, 2, 539–547 CrossRef CAS PubMed.
- G. G. Pepeu and M. G. Giovannini, Curr. Alzheimer Res., 2009, 6, 86–96 CrossRef CAS PubMed.
- J. Hardy, J. Neurochem., 2009, 110, 1129–1134 CrossRef CAS PubMed.
- X. Huang, C. S. Atwood, M. A. Hartshorn, G. Multhaup, L. E. Goldstein, R. C. Scarpa, M. P. Cuajungco, D. N. Gray, J. Lim, R. D. Moir, R. E. Tanzi and A. I. Bush, Biochemistry, 1999, 38, 7609–7616 CrossRef CAS PubMed.
- E. Gaggelli, H. Kozlowski, D. Valensin and G. Valensin, Chem. Rev., 2006, 106, 1995–2044 CrossRef CAS PubMed.
- P. Zambenedetti, H. P. Schmitt and P. Zatta, J. Alzheimer's Dis., 2002, 4, 459 CAS.
- P. Zatta, D. Drago, P. Zambenedetti, S. Bolognin, E. Nogara, A. Peruffo and B. Cozzi, J. Chem. Neuroanat., 2008, 36, 1–5 CrossRef CAS PubMed.
- B. Lopez-Iglesias, C. Perez, J. A. Morales-Garcia, S. Alonso-Gil, A. Perez-Castillo, A. Romero, M. G. Lopez, M. Villarroya, S. Conde and M. I. Rodriguez-Franco, J. Med. Chem., 2014, 57, 3773–3785 CrossRef CAS PubMed.
- A. Kochi, T. J. Eckroat, K. D. Green, A. S. Mayhoub, M. H. Lim and S. Garneau-Tsodikova, Chem. Sci., 2013, 4, 4137 RSC.
- A. K. Sharma, S. T. Pavlova, J. Kim, D. Finkelstein, N. J. Hawco, N. P. Rath, J. Kim and L. M. Mirica, J. Am. Chem. Soc., 2012, 134, 6625–6636 CrossRef CAS PubMed.
- E. Simoni, S. Daniele, G. Bottegoni, D. Pizzirani, M. L. Trincavelli, L. Goldoni, G. Tarozzo, A. Reggiani, C. Martini, D. Piomelli, C. Melchiorre, M. Rosini and A. Cavalli, J. Med. Chem., 2012, 55, 9708–9721 CrossRef CAS PubMed.
- M. I. Fernandez-Bachiller, C. Perez, L. Monjas, J. Rademann and M. I. Rodriguez-Franco, J. Med. Chem., 2012, 55, 1303–1317 CrossRef CAS PubMed.
- I. Bolea, J. Juarez-Jimenez, C. de Los Rios, M. Chioua, R. Pouplana, F. J. Luque, M. Unzeta, J. Marco-Contelles and A. Samadi, J. Med. Chem., 2011, 54, 8251–8270 CrossRef CAS PubMed.
- S. Lee, X. Zheng, J. Krishnamoorthy, M. G. Savelieff, H. M. Park, J. R. Brender, J. H. Kim, J. S. Derrick, A. Kochi, H. J. Lee, C. Kim, A. Ramamoorthy, M. T. Bowers and M. H. Lim, J. Am. Chem. Soc., 2014, 136, 299–310 CrossRef CAS PubMed.
- J. Sterling, Y. Herzig, T. Goren, N. Finkelstein, D. Lerner, W. Goldenberg, I. Miskolczi, S. Molnar, F. Rantal, T. Tamas, G. Toth, A. Zagyva, A. Zekany, G. Lavian, A. Gross, R. Friedman, M. Razin, W. Huang, B. Krais, M. Chorev, M. B. Youdim and M. Weinstock, J. Med. Chem., 2002, 45, 5260–5279 CrossRef CAS PubMed.
- C. W. Ritchie, A. I. Bush and A. Mackinnon, et al., Arch. Neurol., 2003, 60, 1685–1691 CrossRef PubMed.
- L. Lannfelt, K. Blennow, H. Zetterberg, S. Batsman, D. Ames, J. Harrison, C. L. Masters, S. Targum, A. I. Bush, R. Murdoch, J. Wilson and C. W. Ritchie, Lancet Neurol., 2008, 7, 779–786 CrossRef CAS PubMed.
- P. A. Adlard, R. A. Cherny, D. I. Finkelstein, E. Gautier, E. Robb, M. Cortes, I. Volitakis, X. Liu, J. P. Smith, K. Perez, K. Laughton, Q.-X. Li, S. A. Charman, J. A. Nicolazzo, S. Wilkins, K. Deleva, T. Lynch, G. Kok, C. W. Ritchie, R. E. Tanzi, R. Cappai, C. L. Masters, K. J. Barnham and A. I. Bush, Neuron, 2008, 59, 43–55 CrossRef CAS PubMed.
- P. C. Trippier, K. Jansen Labby, D. D. Hawker, J. J. Mataka and R. B. Silverman, J. Med. Chem., 2013, 56, 3121–3147 CrossRef CAS PubMed.
- S. Moussaoui, M.-C. Obinu, N. Daniel, M. Reibaud, V. Blanchard and A. Imperato, Exp. Neurol., 2000, 166, 235–245 CrossRef CAS PubMed.
- Z. Luo, J. Sheng, Y. Sun, C. Lu, J. Yan, A. Liu, H. B. Luo, L. Huang and X. Li, J. Med. Chem., 2013, 56, 9089–9099 CrossRef CAS PubMed.
- Z. Luo, L. Liang, J. Sheng, Y. Pang, J. Li, L. Huang and X. Li, Bioorg. Med. Chem., 2014, 22, 1355–1361 CrossRef CAS PubMed.
- Z. Wang, Y. Wang, W. Li, F. Mao, Y. Sun, L. Huang and X. Li, ACS Chem. Neurosci., 2014, 5, 952–962 CrossRef CAS PubMed.
- U. K. Mazumder, M. Gupta, S. Bhattacharya, S. S. Karki, S. Rathinasamy and S. Thangavel, J. Enzyme Inhib. Med. Chem., 2004, 19, 185–192 CrossRef CAS PubMed.
- Y. T. Pratt and N. L. Drake, J. Am. Chem. Soc., 1960, 82, 1155–1161 CrossRef CAS.
- N. B. Patel, J. C. Patel and S. H. Modi, J. Saudi Chem. Soc., 2011, 15, 167–176 CrossRef CAS.
- V. P. Singh, H. B. Singh and R. J. Butcher, Eur. J. Org. Chem., 2011, 2011, 5485–5497 CrossRef CAS.
- K. Selvakumar, P. Shah, H. B. Singh and R. J. Butcher, Chem.–Eur. J., 2011, 17, 12741–12755 CrossRef CAS PubMed.
- B. K. Sarma and G. Mugesh, J. Am. Chem. Soc., 2005, 127, 11477–11485 CrossRef CAS PubMed.
- B. K. Sarma and G. Mugesh, Chem.–Eur. J., 2008, 14, 10603–10614 CrossRef CAS PubMed.
- K. Satheeshkumar and G. Mugesh, Chem.–Eur. J., 2011, 17, 4849–4857 CrossRef CAS PubMed.
- A. J. Mukherjee, S. S. Zade, H. B. Singh and R. B. Sunoj, Chem. Rev., 2010, 110, 4357–4416 CrossRef CAS PubMed.
- X. Ning, Y. Guo, X. Wang, X. Ma, C. Tian, X. Shi, R. Zhu, C. Cheng, Y. Du, Z. Ma, Z. Zhang and J. Liu, J. Med. Chem., 2014, 57, 4302–4312 CrossRef CAS PubMed.
- R. Sultana, S. Newman, H. Mohmmad-Abdul, J. N. Keller and D. A. Butterfield, Free Radical Res., 2004, 38, 449–558 CrossRef CAS PubMed.
- S. Saharan and P. K. Mandal, J. Alzheimer's Dis., 2014, 40, 519–529 CAS.
- A. E. Roher, J. D. Lowenson, S. Clarke, A. S. Woods, R. J. Cotter, E. Gowing and M. J. Ball, Proc. Natl. Acad. Sci. U. S. A., 1993, 90, 10836–10840 CrossRef CAS.
- R. Mayeux, M.-X. Tang, D. M. Jacobs, J. Manly, K. Bell, C. Merchant, S. A. Small, Y. Stern, H. M. Wisniewski and P. D. Mehta, Ann. Neurol., 1999, 46, 412–416 CrossRef CAS PubMed.
- S. Varadarajan, J. Kanski, M. Aksenova, C. Lauderback and D. A. Butterfield, J. Am. Chem. Soc., 2001, 123, 5625–5631 CrossRef CAS PubMed.
- M. Rosini, E. Simoni, M. Bartolini, A. Cavalli, L. Ceccarini, N. Pascu, D. W. McClymont, A. Tarozzi, M. L. Bolognesi, A. Minarini, V. Tumiatti, V. Andrisano, I. R. Mellor and C. Melchiorre, J. Med. Chem., 2008, 51, 4381–4384 CrossRef CAS PubMed.
- R. Di Santo, R. Costi, G. Cuzzucoli Crucitti, L. Pescatori, F. Rosi, L. Scipione, D. Celona, M. Vertechy, O. Ghirardi, P. Piovesan, M. Marzi, S. Caccia, G. Guiso, F. Giorgi and P. Minetti, J. Med. Chem., 2012, 55, 8538–8548 CrossRef CAS PubMed.
- B. Ou, M. Hampsch-Woodill and R. L. Prior, J. Agric. Food Chem., 2001, 49, 4619–4626 CrossRef CAS PubMed.
- A. Dávalos, C. Gómez-Cordovés and B. Bartolomé, J. Agric. Food Chem., 2004, 52, 48–54 CrossRef PubMed.
- J. He, D. Li, K. Xiong, Y. Ge, H. Jin, G. Zhang, M. Hong, Y. Tian, J. Yin and H. Zeng, Bioorg. Med. Chem., 2012, 20, 3816–3827 CrossRef CAS PubMed.
- J.-S. Choi, J. J. Braymer, R. P. R. Nanga, A. Ramamoorthy and M. H. Lim, Proc. Natl. Acad. Sci. U. S. A., 2010, 107, 21990–21995 CrossRef CAS PubMed.
- M. I. Rodríguez-Franco, M. I. Fernández-Bachiller, C. Pérez, B. Hernández-Ledesma and B. Bartolomé, J. Med. Chem., 2006, 49, 459–462 CrossRef PubMed.
- M. Decker, B. Kraus and J. Heilmann, Bioorg. Med. Chem., 2008, 16, 4252–4261 CrossRef CAS PubMed.
- S. R. Wilson, P. A. Zucker, R. R. C. Huang and A. Spector, J. Am. Chem. Soc., 1989, 111, 5936–5939 CrossRef CAS.
- P. Merino-Montiel, Ó. López and J. G. Fernández-Bolaños, Tetrahedron, 2012, 68, 3591–3595 CrossRef CAS.
- L. Di, E. H. Kerns, K. Fan, O. J. McConnell and G. T. Carter, Eur. J. Med. Chem., 2003, 38, 223–232 CrossRef CAS PubMed.
- P. Camps, X. Formosa, C. Galdeano, D. Muñoz-Torrero, L. Ramírez, E. Gómez, N. Isambert, R. Lavilla, A. Badia, M. V. Clos, M. Bartolini, F. Mancini, V. Andrisano, M. P. Arce, M. I. Rodríguez-Franco, Ó. Huertas, T. Dafni and F. J. Luque, J. Med. Chem., 2009, 52, 5365–5379 CrossRef CAS PubMed.
- F. Wohnsland and B. Faller, J. Med. Chem., 2001, 44, 923–930 CrossRef CAS PubMed.
Footnote |
† Electronic supplementary information (ESI) available: NMR spectrums, LC-HRMS spectrums, computation studies, BBB-assay and GPx-like of target compounds. See DOI: 10.1039/c5ra26797h |
|
This journal is © The Royal Society of Chemistry 2016 |